the Creative Commons Attribution 4.0 License.
the Creative Commons Attribution 4.0 License.
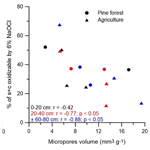
Tropical Andosol organic carbon quality and degradability in relation to soil geochemistry as affected by land use
Sastrika Anindita
Peter Finke
Steven Sleutel
Land use is recognized to impact soil geochemistry on the centennial to millennial timescale, with implications for the distribution and stability of soil organic carbon (SOC). Young volcanic soils in tropical areas are subject to much faster pedogenesis, noticeable already on the centennial or even decadal timescale. As land use is a recognized factor for soil formation, it is thus conceivable that even relatively recent land use conversion in such areas would already bear a significant impact on the resulting formed soils., e.g., in terms of content of pedogenic oxides. Very scarce observational evidence exists, so such indirect implications of land use on SOC cycling are largely unknown. We here investigated SOC fractions, substrate-specific mineralization (SOC or added plant residue), and net priming of SOC as a function of forest or agricultural land use on Indonesian volcanic soils. The content of oxalate-extracted Al (Alo) correlated well with organic carbon (OC) associated with sand-sized aggregates, particularly in the subsoil. The proportion of SOC in sand-sized ultrasonication-resistant (400 J mL−1) aggregates was also higher in agricultural land use compared to pine forest land use, and a likewise contrast existed for Alo. These combined observations suggest that enhanced formation of Al (hydr)oxides promoted aggregation and physical occlusion of OC. This was, importantly, also consistent with a relatively lesser degradability of SOC in the agricultural sites, though we found no likewise difference in degradability of added 13C-labeled ryegrass or in native SOC priming between the pine forest and agricultural land uses. We expected that amorphous Al content under agricultural land use would mainly have promoted mineral association of SOC compared to under pine forest land use but found no indications for this. Improved small-scale aggregation of tropical Andosols caused by conversion to agriculture and high carbon input via organic fertilizer may thus partially counter the otherwise expectable decline of SOC stocks following cultivation. Such indirect land use effects on the SOC balance appeared relevant for correct interpretation and prediction of the long-term C balance of (agro)ecosystems with soil subject to intense development, like the here-studied tropical Andosols.
- Article
(1965 KB) - Full-text XML
-
Supplement
(333 KB) - BibTeX
- EndNote
Storage of soil organic carbon (SOC) in terrestrial ecosystems can improve ecosystem services such as soil health, agricultural productivity, and climate change mitigation (Baldock, 2007; Lehmann and Kleber, 2015). The storage of SOC is influenced by the interaction of ecological processes with net primary productivity and heterotrophic respiration usually being the most important terms of the SOC balance. Land use determines the net C input to soil and in doing so often bears an overriding control on the SOC balance compared to other drivers of the C cycle at ecosystem level. Forest ecosystems usually have relatively high net primary productivity compared to agricultural land use (Smith et al., 2014). The net result of cultivation then typically leads to a decline in SOC stock and increased human-induced CO2 emission (Don et al., 2011; Wei et al., 2014). The long-term SOC balance of ecosystems also depends on the capacity of soils to stabilize newly entering organic carbon (OC) against microbial decomposition, resulting from heterotrophic respiration. Physical occlusion of OC at the microaggregate scale and binding of OC onto reactive mineral surfaces constitute two major mechanisms for stabilization of SOC (Matus et al., 2014). Soil characteristics such as content of poorly crystalline minerals, specific surface area, clay content, and degree of soil microaggregation have all been linked to the capacity of soils to accumulate SOC (Hernández et al., 2012; Kleber et al., 2015; Mikutta et al., 2006, 2009; Poirier et al., 2020). While soil mineralogy itself is thus a well-recognized controller of SOC stabilization (Basile-Doelsch et al., 2005; Hernández et al., 2012; Lyu et al., 2021; Tamrat et al., 2019), surprisingly little attention has gone into understanding how land use history, through its impact on soil geochemistry, might in turn indirectly control stability of SOC and organic matter (OM) entering soil.
The geochemistry of the reactive mineral phase of a soil is the resultant of both the composition of its parent material and soil weathering status (Mikutta et al., 2009). Weathering status is crucially driven by the time since the onset of weathering, local climate and hydrologic conditions, resilience of minerals to weathering, and vegetation cover (Doetterl et al., 2015). Land use, through its control on vegetation, can impact the weathering process by modifying the pH, influencing soil biological activity and nutrient levels, and releasing organic complexing compounds (Van Breemen et al., 1983; Cronan, 2018). On agricultural lands, moreover, fertilizer addition and soil disturbance caused by tillage have also been reported to significantly enhance weathering (Churchman and Lowe, 2012; Li et al., 2021; Taylor et al., 2016). While an extensive and still-growing number of studies considered land use impacts on SOC stock and soil OM quality and its degradability (Covaleda et al., 2011; Cusack et al., 2013; Huygens et al., 2005), just very few have considered the potential indirect impact of land use on SOC stability through its effect on the geochemical properties. Moreover, the coverage remains limited for several soil groups, including tropical Andosols. A recent study on the Galápagos Islands demonstrated that, even after a maximum of 15 years, conversion of native forest to cultivated land strikingly accelerated soil weathering (Gerzabek et al., 2019). In particular, formation of secondary minerals should impact SOC retention in volcanic soils as their high capacity to do so is acknowledged to emerge from abundantly present poorly crystalline Al and Fe oxides. Indeed, Asano and Wagai (2014) highlighted the importance of short-range-order minerals or organo-Al complexes for SOC stabilization in Andosols from correlations with OC stock (Miyazawa et al., 2013), from mean residence time (reviewed by Parfitt, 2009), and by chemical characterization of organo-mineral associations (Basile-Doelsch et al., 2007; Mikutta et al., 2009). Next to a large specific surface area, also microaggregation (and occlusion therein) of intra-microaggregate particulate OM (iPOM) is known to grant a degree of protection against its microbial decay (Six et al., 2000a), particularly in Andosols (Asano et al., 2018; Asano and Wagai, 2014). Introduction of tillage and removal of permanent vegetative coverage usually adversely affect iPOM-induced physical protection (Besnard et al., 1996). However, differences between untilled native vegetation or secondary forest, on the one hand, and tilled cropland, on the other, might not be significant for volcanic soils (Dörner et al., 2012; Huygens et al., 2005; Linlin et al., 2016) as abundant nano-sized organo-mineral composites especially act as binding agents in microaggregates in Andosols (Asano and Wagai, 2015).
In Indonesia, the Sunda volcanic arc region (Mt. Tangkuban Perahu and Mt. Burangrang) in West Java was covered by native forest until only about two centuries back but has since then been largely replaced by secondary pine forest or agricultural land use. The co-occurrence of native forest, secondary forest, and agricultural land use forms a useful means to understand how land use would influence geochemical properties and impact SOC storage and stability in volcanic soils. A first objective was to further investigate how cultivation history in this region would have resulted in differential soil aggregation and levels of iPOM, as well as mineral-associated OM vs. free relatively unprotected OM. We did so by assessing soil OM fractions for a set of six Indonesian volcanic soils (Cambisols and Andosols) with native forest, secondary pine forest, or agricultural land use. A second goal was to infer how OM degradability in the topsoil of these tropical volcanic soils itself differs as a function of forest vs. agricultural land use. Assessing the indirect effect of land use on SOC degradability through its mediation of soil mineralogy is, however, complicated by the fact that the quality of native SOC itself is also a function of land use. We therefore assessed the degradability of a single model exogenous OM source (13C-labeled ryegrass residues) to see how land use changes affect stability of OC in soil. We hypothesize that the enhanced formation of pedogenic poorly crystalline Al under agricultural land use, as we previously confirmed (Anindita et al., 2022), results in a relative stabilization of the exogenous OM as compared to native forest or secondary forest. In doing so, we take account of the well-known phenomenon that labile OC inputs can accelerate the decomposition of native SOC, a process called positive priming (Chen et al., 2014), or conversely restrain decomposition of SOC and induce negative priming (Blagodatskaya et al., 2014; Qiao et al., 2014). By using a C-isotope mixing model (Werth and Kuzyakov, 2010), mineralization of the exogenous OM (i.e., ryegrass) and native SOC are distinguished in the soil incubation experiments.
2.1 Site description and soil sampling
Our study covered soils from the Mount Tangkuban Perahu and Mount Burangrang regions that are part of the Sunda volcanic complex in West Java, Indonesia. Six sites were selected to represent the dominant land use types in the area, viz. primary forest (NF-y), pine forest (PF-i and PF-o), and horticulture (AG-y, AG-i, and AG-o). The mean annual temperature in the study sites is 19–21 ∘C, and mean annual precipitation ranges around 2000–3000 mm per year. Soils at sites NF-y and PF-i are andic Cambisols, and at the PF-o, AG-y, AG-i, and AG-o sites they are aluandic Andosols (Anindita et al., 2022). All soils contain silicate minerals (i.e., quartz, cristobalite, tridymite), gibbsite, albite, hornblende, 1:1 clay minerals, and non-crystalline materials (Anindita et al., 2022). The proportion of primary minerals is higher in the younger NF-y soil as it is closer (within 1.5 km) to the crater of Mt. Tangkuban Perahu and received ash more recently. The pine forest and agricultural sites were originally all under the primary forest vegetation. At PF-i and PF-o, secondary forests were planted in 1962 for restoration purposes, following earlier deforestation. Agricultural land use at AG-y, AG-i, and AG-o dates back to just 30–50 years ago with mainly diverse horticultural crop rotations with crops such as potatoes (Solanum tuberosum), cabbage (Brassica oleracea), green beans (Phaseolus vulgaris), tomatoes (Solanum lycopersicum), and chayote (Sechium edule). Detailed information about land use history and land management is given in (Anindita et al., 2022).
Table 1Physicochemical soil properties of six sampled soil profiles in the Sunda volcanic complex in West Java, Indonesia (partly taken from Anindita et al., 2022).
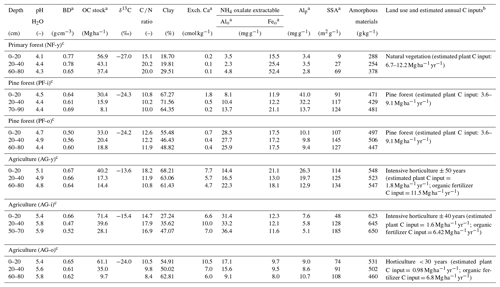
a BD = bulk density; OC = organic carbon; Alo = aluminum extracted by NH4 oxalate; Feo = iron extracted by NH4 oxalate; Alp = aluminum extracted by Na-pyrophosphate; SSA = specific surface area; Exch. Ca = exchangeable calcium. b C inputs from vegetation (forest or annual crops) and organic amendments. Forest C inputs estimated from studies on Indonesian pine forest (Bruijnzeel, 1985) and Indonesian tropical primary forest (Guillaume et al., 2018; Hertel et al., 2009). Crop C inputs based on farmer interviews as well as the Soil Service of Belgium (SSB) and the University of Ghent (2008). C inputs from organic amendments were based on farmer interviews and lab analyses. c History of land use can be referred to in Anindita et al. (2022).
At every site, soil samples were taken at 0–20, 20–40, and 60–80 cm depths. At each depth, the bulk samples were mixed into composite ones (three subsamples), which were then dried in air, homogenized, and sieved (< 2 mm) until further analysis at the Department of Environment at Ghent University (Belgium). The pH of H2O was measured using a glass electrode pH meter in a 1:5 soil solution slurry. The estimated clay content was determined using the pipet method after full soil dispersion with Na+ resin (Bartoli et al., 1991), after testing with several other procedures for soil texture analysis that proved incompatible with the investigated set of volcanic soils. The concentrations of total C and N were determined by dry combustion at 1100 ∘C using a LECO 928 series CN analyzer. Aluminum extracted by 0.1 M pyrophosphate solution (Alp) was taken as a measure of complexed Al to OM (McKeague, 1967). Iron and aluminum extractable by ammonium oxalate (Alo, Feo) were used as estimates of X-ray amorphous Al and Fe. We previously quantified crystalline minerals using X-ray diffraction (XRD) analysis. The amount of amorphous materials undetectable by XRD was semi-quantitatively estimated from the difference between the obtained and the real amount of internal standard (zincite 20 %) after semi-quantification of crystalline minerals using BGMN Rietveld and Profex as the user interface. Soil specific surface area (SSA) was assessed from adsorption–desorption of N2 at 77 ∘C following the Brunauer–Emmett–Teller (BET) approach. Detailed methods and the results of geochemical analyses of the samples were documented in Anindita et al. (2022). Selected physicochemical soil properties are given in Table 1. More detailed information on geochemical soil properties can be found in Table S1 in the Supplement.
2.2 Soil organic matter fractionation
Soils were fractionated using size, density, and chemical separation steps according to a modified version of a fractionation scheme proposed by Zimmermann et al. (2007) (Fig. S1 in the Supplement). This procedure separates SOC into five pools, namely, (i) OC contained in sand and associated with soil aggregates (S + A), (ii) in free particulate organic matter (POM), (iii) in water dissolvable OC (DOC), (iv) in oxidizable OC associated with the silt and clay (s + c − rSOC) fraction, and (v) in a chemically resistant SOC (rSOC) pool. Briefly, 30 g of equivalent dry soil (< 2 mm) was initially dispersed in water by a calibrated ultrasonic probe with an output energy of 22 J mL−1. The resulting slurries were wet sieved over a 63 µm sieve and rinsed with deionized water until the rinsing water became clear. In the original Zimmermann et al. (2007) method, the > 63 µm fraction is further separated into S + A and POM based on density differences using sodium polytungstate at 1.8 g cm−3. However, several samples could not be well separated into free OM, on the one hand, and predominantly mineral material of the S + A fraction, on the other. After pre-tests, we instead used a 1.5 g cm−3 sodium polytungstate solution to separate free POM from the S + A soil fraction, as per the advice by Cerli et al. (2012) to optimize the density cutoff per soil for best separation of free and occluded POM. A subsample of the < 63 µm suspension (± 10 mL) derived from the wet sieving step was filtered through a 0.45 µm nylon membrane filter. The filtrate was analyzed for its dissolved OC concentration using a FormacsHT total organic carbon analyzer (Skalar, the Netherlands). The DOC pool was calculated by multiplying this OC concentration with the volume of flush water used in the preceding wet sieving step. The < 63 µm suspension was dried at 40 ∘C and weighed to constitute the overall silt plus clay (s + c) fraction. A chemically inert share of the SOC (rSOC) was isolated by subjecting the s + c fraction to oxidation by 6 % NaOCl. To this end, 1 g of s + c material was weighed inside a 65 mL Nalgene centrifuge tube, and 50 mL of 6 % NaOCl adjusted to pH 8 was added and allowed to react for 18 h at 25 ∘C. After centrifugation, remnant NaOCl was discarded by decantation. This procedure was repeated three times; the pellet was washed three more times with deionized water, transferred to a pre-weighed aluminum cup, dried at 40 ∘C, and weighed. The obtained S + A fraction remains composite as it contains silt + clay-associated OC next to occluded POM. In addition to the original scheme proposed by Zimmermann et al. (2007) and Poeplau et al. (2013), a further fractionation of the S + A into s + c and POM and sand-associated OM was included: A 3 g subsample of the obtained S + A fraction was further dispersed by ultrasonication at 400 J mL−1 and then separated into two size fractions by passing the obtained slurries over a 63 µm sieve. The obtained < 63 µm fraction contains s + c OM. Meanwhile, the > 63 µm fraction primarily contains occluded POM and sand-bound OM contained in the S + A fraction as well as OM contained in the > 63 µm aggregates resistant to dispersal at 400 J mL−1. The S + A and s + c fractions were further on considered to constitute a single joint OM fraction with s + c obtained after the first 22 J mL−1 ultrasonication and 63 µm sieving step. All fractions were analyzed for their C concentration using a LECO 928 series CN analyzer.
2.3 Soil incubation experiment and isotopic signature measurements
Soils were incubated in a standardized way to compare degradability of a model plant C substrate and SOC in the topsoil (0–20 cm) of the six considered sites. Decomposition of either substrate was derived by regular measurement of the soil CO2 efflux and inference of its δ13C signature. Soil mesocosms were prepared by repacking approximately 150 g of soil (depending on bulk density) into PVC tubes (diameter: 6.8 cm, height: 7 cm) to reach a height of 6.2 cm and bulk density as encountered at the field sites. Soil moisture content was set to 50 % water-filled pore space by addition of deionized water. A 13C-labeled plant substrate, i.e., pulse-labeled ryegrass (Lolium perenne) with δ13C of + 53.7 ‰, was applied at a dose of 1 g kg−1 of dry matter. The contrast in δ13C with SOC (Table 1) allowed us to distinguish emitted CO2 into parts stemming from either native SOC or grass C mineralization. The δ13C of topsoil (expressed as δ13C value (‰) vs. the international Vienna Pee Dee Belemnite standard) was measured using a PDZ Europe ANCA-GSL elemental analyzer, interfaced with a Sercon 20-22 isotope-ratio mass spectrometer (IRMS) with SysCon electronics (SerCon, Cheshire, UK).
Soils were incubated at 20 ∘C for 120 d. On days 1, 3, 5, 8, 12, 17, 24, 32, 38, 47, 59, 73, 87, 101, 111, and 120, soil CO2 emission was inferred by measuring CO2 buildup in a cylindrical closed chamber attached consecutively on top of each PVC tube for at least 10 min. The evolution of the headspace CO2 concentration and its δ13C was measured every 4 s by connecting the closed chamber with a cavity ring-down spectrometer (G2201-i CRDS isotopic CO2 analyzer, Picarro, USA) in a loop via Teflon tubing. The soil CO2 efflux rate (in ) was calculated from the slope of the accumulating CO2 concentration as a function of time using the ideal gas law. The δ13C of emitted CO2 was estimated from the y-axis intercept of derived Keeling plots (Keeling, 1958). The fraction of CO2 derived from grass was calculated using the following equations introduced by Werth and Kuzyakov (2010):
with εSOC (in ‰) being the net C isotopic fractionation resulting from native SOC mineralization and diffusive CO2 transport in soil until its efflux into the headspace air. The εSOC was obtained from CO2 efflux monitoring of parallel triplicate sets of control soils without grass added. A third parallel set of soils was amended with a very high dose of grass (viz. 6 g kg−1 added grass, i.e., about 4 t C ha−1) to estimate εgrass. This value was operationally calculated by subtracting from the peak CO2 emission δ13C-CO2 of soil with 6 g kg−1 grass under the assumption that virtually any emitted CO2 was then derived from grass mineralization.
Using Eqs. (1)–(3), the fraction of emitted CO2 derived from grass C mineralization was calculated per soil and per point in time. The rate of grass C mineralization (Cgrass-min, in ) for each measurement day was calculated by multiplying the fgrass value with the total CO2 emission. The rate of SOC mineralization (CSOC min, in ) was calculated by subtracting the rate of Cgrass-min from the total CO2-C emission rate. Cumulative CO2 emissions derived from native SOC-derived C and grass C were obtained by consecutively summing up and , respectively, per time increment between flux measurements. The percent (%) of mineralized CSOC was then derived from dividing by CSOC.
2.4 Statistics
Two-way ANOVA with depth increment and land use as fixed factors was used to compare soil properties and the distribution of OC over soil fractions. For individual increments (0–20, 20–40, and ± 60–80 cm), additional t tests were used as well to test for specific differences between pine forest and agricultural land use. Total, ryegrass, and native SOC cumulative OC mineralization and net cumulative priming of native SOC were only assessed for the 0–20 cm depth increment, and the comparison of means between the pine forest and agriculture land uses was made by independent sample t tests. The primary forest land use was excluded from this comparison as it emerged that the parent material from which it developed differed from the other sites (Anindita et al., 2022). Pearson correlation coefficients were used to investigate relations between soil fraction SOC proportions, soil OC mineralization, and geochemical properties for the group of pine forest and agricultural soils. Analyses were completed in IBM SPSS Statistics 27.
3.1 Organic carbon in soil fractions
Across the studied soils, a greater part of the soil material (± 70 %) was found as the s + c fraction (Table 2). There were particularly large proportions of s + c in the PF-i, PF-o, and AG-o soils (> 80 %). Conversely, in the AG-i subsoil, the S + A fraction was the largest. The OC concentration of soil fractions decreased in the following order: POM > s + c (including rSOC) > S + A > DOC and was generally higher in the topsoil than subsoil. The OC concentration of the S + A fraction was higher under agricultural than under forest land use (p < 0.1), particularly in topsoils of the sites under agricultural land use (30–50 g C kg−1 fraction). No such difference in OC concentration existed between SOC in the s + c, POM, DOC, or rSOC fractions under agriculture compared to forest (i.e., including primary forest). The OC concentrations of s + c and s + c − rSOC fractions were generally higher (p < 0.1) in the agricultural soils when compared to the pine forest soils solely. The size of the DOC fraction was negligibly small (± 0.002 g C kg−1 fraction) and is further disregarded. The mean total SOC content in all fractions was higher in agricultural soils (29.6 ± 15.1 g C kg−1 soil) than pine forest soils (16.3 ± 7.7 g C kg−1 soil) (p < 0.05).
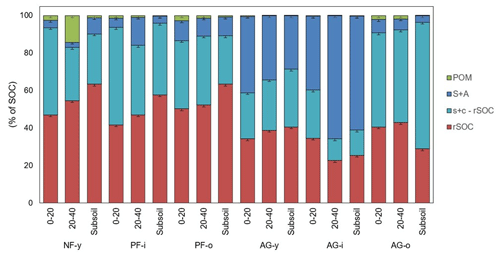
Figure 1The distribution of OC over soil fractions obtained by a modified version of the Zimmermann et al. (2007) procedure for six soil profiles sampled at 0–20, 20–40, ± 60–80 cm in the Sunda volcanic complex in West Java, Indonesia. Error bars indicate standard deviations for three lab replicates (n = 3).
Overall, the bulk of SOC was present in the s + c fraction (± 79 %) with higher proportion in rSOC (± 44 %) than in the s + c − rSOC (± 35 %) fraction, followed by S + A (± 19 %) and POM (± 1 %) fractions (Fig. 1). The mean OC content in the S + A fraction was higher in the agricultural (11.3 ± 10.3 g C kg−1 soil) than pine forest (1.5 ± 0.6 g C kg−1 soil; though only p = 0.09) soils. Likewise, its relative contribution to SOC was larger in the agricultural soils (32 % of SOC), when compared to all three forest sites (8 % of SOC) or to just the two pine forest sites (9 % of SOC) (though only at p = 0.08). Particularly in AG-y at a depth range of 0–20 cm (12.8 g C kg−1 soil) and in AG-i at depths of 0–20 and 20–40 cm (22.3 and 30.6 g C kg−1 soil, respectively), a larger share of the SOC was in the S + A fraction. The s + c fraction followed an opposite trend with a larger SOC proportion under forest (all three (89.5 % of SOC) or just the two pine forests (89.8 % of SOC)) than under agricultural land use (68 % of SOC) (p < 0.08) (Fig. 1). Out of the s + c fraction the resistant SOC (rSOC) fraction accounted for approximately a third to half of SOC, and its proportion (52 % of SOC) was likewise higher under pine forest when compared to agricultural land use (p < 0.05). Free POM contributed on average less than 5 % of SOC. The occasional low concentrations of free POM C (Table 2) indicate that some mineral matter was left in the isolated POM fraction. However, given its very small mass proportion (median 0.1 %), this artifact has negligible further impact on the overall distribution of SOC across soil fractions. The SOC proportion of free POM (percent of SOC) was also higher under pine forest than agricultural land use (p < 0.01).
3.2 C:N ratio, amorphous aluminum and iron in the sand aggregates (S + A), and silt–clay (s + c) fractions
The ratio of C:N ranged from 7.3–19.8 in S + A and 7.5–20.1 in s + c (Table 3), and their mean values did not differ. The S + A C:N ratio was higher in the agricultural soils than in the pine forest soils (p = 0.07). The content of Alo varied from 1.7 to 56.6 and from 3.2 to 46.6 g kg−1 in the S + A and s + c fractions, respectively. Meanwhile, Feo ranged from 9.6 to 34.3 and from 7.6 to 74.2 g kg−1 in the S + A and s + c fractions, respectively. A much lower Alo content in the NF-y site s + c and S + A fractions compared to all other soils was in line with its distinctive total elemental composition previously reported by Anindita et al. (2022). The mean Alo content in the S + A fraction at all depths was higher under agriculture (28.9 ± 20.8 mg g−1) than pine forest (20.9 ± 12.4 mg g−1) (p = 0.09). The Feo contents in S + A and s + c fractions were generally higher under pine forest than agricultural soils, particularly at depths of 20–40 and ± 60–80 cm, with no significant difference.
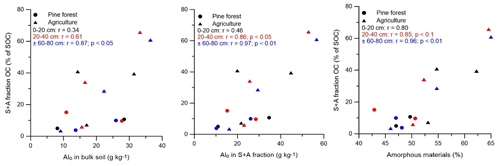
Figure 2Scatter plots of the SOC proportion of the S + A fraction and the amounts of Alo (in bulk soil and sand-aggregate fraction) and amorphous materials. Symbols and Pearson correlation coefficients in black, red, and blue represent correlation at depths of 0–20, 20–40, and ± 60–80 cm, respectively. Sites under agriculture and pine forest are indicated by ▴ and •, respectively.
3.3 Correlations between SOC fractions and selected chemical soil properties
The proportion of several of the isolated SOC fractions correlated to some of the geochemical soil properties (Fig. 2). By considering only pine forest and agricultural soils, the SOC proportion of the S + A fraction was positively correlated with Alo (in bulk soil as well as in the S + A fraction), C:N ratio of the S + A fraction, and content of amorphous materials at all depths (p < 0.05). These soil properties were also positively correlated with the amount of OC in the S + A fraction (g C kg−1 soil) and OC concentration in the S + A fraction (g C kg−1 fraction). Positive correlations existed between SOC proportion in the s + c fraction with the amount of the Feo s + c fraction at a depth of ± 60–80 cm (p < 0.05), but the amount of OC (g C kg−1 soil) and OC concentration (g C kg−1 fraction) in the s + c fraction correlated negatively to the Feo s + c fraction at all depths. The proportion of rSOC in the s + c fraction (i.e., rSOCs + c × 100) correlated positively (p < 0.05) to Alo content at a depth of 60–80 cm (r = 0.9), SSA at 20–40 cm (r = 0.9), and the micropore volume at 0–20 and 20–40 cm (r = 0.66 and r = 0.86, respectively).
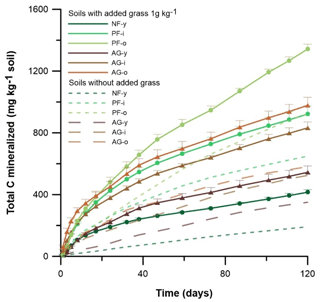
Figure 3Gross cumulative amount of C mineralized in soil with and without 1 g C kg−1 ryegrass added. Vertical bars indicate standard deviations of three lab replicates (n = 3) and are presented for the grass-amended soils only. Sites under forest and agriculture are indicated by • and ▴, respectively.
3.4 Carbon mineralization experiment
3.4.1 Gross soil carbon mineralization
Across all sites, C mineralization (C-min) rates were highest for AG-i and AG-o and peaked already during the first day of incubation, while for the other soils rates peaked at day 3 or 5 only. After day 38, the C-min rates stabilized across time. The cumulative total CO2-C emission and derived amount of C mineralized (SOC + ryegrass) generally followed a sequence of NF-y < AG-y < AG-i < PF-i < AG-o < PF-o. Pine forest soils displayed higher cumulative C mineralization than agricultural soils (p < 0.05). Overall, the 120 d cumulative C mineralization was 194–419 mg kg−1 higher in soil with grass added than without (Fig. 3).
3.4.2 Grass C mineralization
The Cgrass-min rates peaked within 12 d after the start of the incubation, and they dropped thereafter to just half to one-third of the initial rates. The Cgrass mineralization rate was conspicuously high on the first day of incubation in soils AG-i and AG-o, whereas for the other soils mineralization peaked on day 5 only. From day 38 onwards, the Cgrass-min rate had become more or less similar in all six sites. By the end of the 120 d incubations, 22 %–38 % of the added ryegrass had been mineralized. The highest cumulative Cgrass-min rate was found for PF-o (389 mg kg−1), followed by AG-i > AG-o≈ PF-i ≈ AG-y > NF-y. There were no significant differences in Cgrass-min between the sets of agricultural and forest soils.
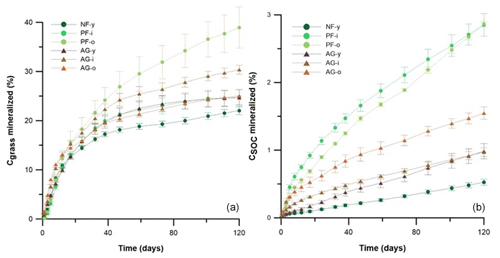
Figure 4The percentage of Cgrass (left) and CSOC (right) mineralized during 120 d of incubation in soils with 1 g kg−1 grass or no grass added, respectively. Vertical bars indicate standard deviations of triplicate lab repetitions. Sites under forest and agriculture are indicated by • and ▴, respectively.
3.4.3 Native SOC mineralization
Mineralization of SOC (CSOC-min) contributed more to gross soil C mineralization than Cgrass-min did. There were large differences in CSOC-min between the 0–20 cm soil layers of the six sites. By the end of the incubation, the highest cumulative amount of SOC mineralized was found for PF-o (953 ± 13 mg kg−1), while a 4-fold lower amount was mineralized in the case of NF-y (195 ± 17 mg kg−1). Significantly less SOC was mineralized in the agricultural soils (517 ± 90 mg kg−1) than in the pine forest soils (813 ± 156 mg kg−1) (p < 0.01). Relatively, 0.5 %–2.9 % of the SOC had been mineralized within the 120 d incubations (Fig. 4). A larger relative share of SOC was mineralized in the pine forest soils (2.9 ± 0.1 % of SOC) than in the agricultural soils (1.2 ± 0.3 % of SOC) (p < 0.01).
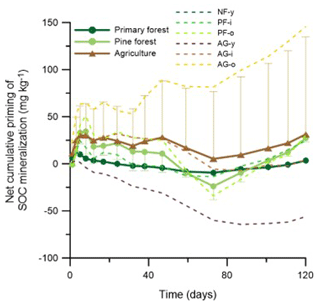
Figure 5Net effect of grass addition (1 g kg−1) on cumulative native SOC mineralization. Positive and negative values denote net positive or net negative cumulative priming of SOC mineralization. Dashed lines present the temporal evolution of the mean net cumulative priming effect for either forest or agricultural land uses, with, respectively, only upper or lower error bars representing the standard deviations on the data.
Priming of native SOC mineralization was assessed by comparing CSOC-min between the soils with and without 1 g kg−1 grass added (Fig. 5). During the first week, grass addition tended to increase the CSOC-min rate in most soils; that is, there was positive priming of native SOC. Along the 120 d experiment, however, the direction and magnitude of the net cumulative priming effect varied strongly among soils. Little or no net priming of CSOC-min occurred in the NF-y soil, as a result of initial positive priming until day 17 but negative priming afterwards. In the pine forest soils, likewise, periods of positive and negative priming alternated, with at the end of the 120 d period an alike limited net positive priming of SOC at both PF sites. Priming of SOC was diverse among the three agricultural soils. While there was strong positive priming throughout the 120 d period in the AG-o soil, the opposite was true for AG-y, and no net priming occurred in the case of AG-i. On average, the net 120 d cumulative priming of SOC in forest (19.3 ) was lower than agricultural (31.2 ) land use, but due to a wide variation between replicate sites, this difference proved insignificant; moreover, net priming rates were not significantly different from zero.
4.1 Land use effects on free and aggregate-protected OC
Overall, the portion of the S + A fraction material (i.e., > 63 µm, ultrasonication-resistant (400 J mL−1) aggregates, and sand particles) was nearly double in the agricultural sites (34 ± 22 %) than in pine forest sites (13 ± 3 %), and it was nearly double compared to all forest, including primary forest (though only at p = 0.1, probably due to the small sample number). This indicated stronger soil aggregation and aggregate stability under agriculture. In agreement, a 4-fold share of SOC resided in the S + A fraction under agriculture (32 ± 22 %) than pine forest (8 ± 4 %) (p = 0.08). The important role of soil microaggregates for SOC storage has been displayed by numerous studies (Dungait et al., 2012; Rabbi et al., 2016; Six et al., 2002). We expected a lesser share of SOC to be occluded inside aggregates in the tilled agricultural soils, as absence of tillage results in less disruption of macroaggregates with increased formation of microaggregates and occlusion of POM (Six et al., 2000b; Zheng et al., 2018). However, tillage is not the only determinant of soil aggregate formation and breakdown as aggregate stability also depends on the presence of binding agents that cluster mineral and organic particles into aggregates as well as size and mineralogy of the less-reactive “skeletal” particles. A further analysis of the soil mineralogy could prove useful to explain the observed variation in storage of OM in the S + A fraction between both land uses. With respect to skeletal particles, the main identified constituents were from the quartz group (tridymite, quartz, cristobalite) (7 %–14 %) and 1:1 clay minerals (5 %–35 %) (Anindita et al., 2022), which, because of their inherent low permanent negative charge, may be considered relatively unreactive as well. In addition, smaller shares of hornblende, andesine, albite, and rutile were present as well. Unsurprisingly, the XRD-estimated levels of these primary minerals and 1:1 clays did not correlate to the mass proportion of the S + A fraction, indeed suggesting that instead mainly other soil constituents acted as binding agents. Moreover, as contents of these relatively unreactive phases did not differ between the PF and AG soils, their variation could not explain the strong contrast in S + A-contained OC between both land uses.
Major binding agents considered to work at micron-to-submicron scales are instead poorly crystalline minerals, redox-sensitive minerals, and microbial products (detritus and metabolites) (Oades and Waters, 1991). Particularly, XRD amorphous materials constituted the bulk of the mineral phase (up to 65 %). The limited oxalate-extracted Si suggested allophane, imogolite, and volcanic glass levels to be minor (Anindita et al., 2022). Hence a large pool of unidentified Al and Fe (oxy-)(hydr)oxides instead likely formed the chief part of the XRD amorphous mineral phase. Particularly, minerals associated with Alo would appear to be relevant colloids as the bulk soil Alo level correlated well with bulk soil micropore volume and specific surface area. Wagai et al. (2018) indeed also found the reactive metal phase to be important binding agents in allophanic Andosols. As NH4 oxalate extraction is not entirely selective toward poorly crystalline minerals (Rennert, 2019), it is probable that Alo partly derived from crystalline gibbsite as well. The same goes for Fe and crystalline pedogenic Fe. All soils contained rather large proportions of XRD-detectable Gibbsite (3 %–15 %) with the exception of AG-y, also with 1 %–3 % magnetite. However, with no consistent differences in their XRD-spectrum proportions between both land uses, and no correlation to the S + A fraction mass proportion, it is unlikely that variation in these secondary crystalline minerals led to contrasting soil aggregation and distribution of OC between the isolated S + A or s + c fractions between the PF and AG land uses. Instead, the S + A mass proportion (in g kg−1 bulk soil) correlated to S + A concentrations (in g kg−1 S + A fraction) of both Alo (r = 0.86; p < 0.05) and OC (r = 0.73; p < 0.05). It is thus likely that the glue between skeletal mineral particles consisted of a mixture of OM and poorly crystalline non-allophane/imogolite Al-containing minerals. In turn, the SOC proportion of the S + A fraction correlated positively with contents of Alo and the percent of X-ray amorphous materials, particularly at depths of 20–40 and ± 60–80 cm (r ± 0.8; p < 0.05). This indicates that higher levels of Alo as well as accumulation of SOC under agriculture and pine forest would have enhanced aggregation and thus a larger share of SOC inside these aggregates. Furthermore, repeated addition of labile C via manure and compost amendments was shown to result in greater formation of macroaggregate (Du et al., 2014; Mikha and Rice, 2004; Yu et al., 2012) by increased production of microbial-derived binding agents. Manure and compost amendments to the AG sites were vast (Table 1), and so the enhanced addition of labile C as compared to the forest sites may have boosted aggregate formation with more SOC storage in the S + A fraction under agricultural land use. Nevertheless, it is long known that soil organic matter (SOM) can have an inhibiting effect on the crystallization process of Al oxides, similar to the anti-gibbsite effect, leading to polymerization rather than crystallization (Borggaard et al., 1990). Kang et al. (2009) as well argued that SOM can inhibit the crystallization of Al, resulting in less crystalline Al oxides, which are in turn adsorbents of SOM. Particularly in a tropical climate where weathering and SOC decomposition occur fast, there may be important positive feedback between accumulation of SOM, which is induced by vast addition of exogenous OM, and formation of poorly crystalline Al in Andosols that has not been looked into to date. Lastly, less free POM was found in the agricultural than forest soils, likely as a logical consequence of more POM occlusion under agriculture. However, we cannot exclude that a better degradability of crop-derived residues vs. pine or native forest litter co-explains these trends. It is well established that litter rich in cutin, waxes, and lignin such as those derived from pine forests is less degradable than plant litter richer in carbohydrates and organic N like most crop residues (Berg and McClaugherty, 2003).
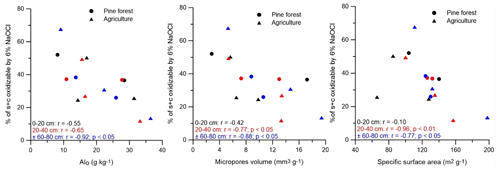
Figure 6Relation between the proportion of SOC that was oxidizable by 6 % NaOCl in the pine forest and agricultural soils with the soil Alo, micropore volume, and soil specific surface area (at each depth n = 5). Sites under agriculture and pine forest are indicated by ▴ and •, respectively. Symbols and Pearson correlation coefficients in black, red, and blue represent correlations at depths of 0–20, 20–40, and ± 60–80 cm, respectively.
4.2 Effect of land use on SOC in the silt and clay fraction
Interaction of SOC with silt and clay provides long-term stabilization of SOC against decomposition and therefore forms a key mechanism for SOC sequestration (De Clercq et al., 2015). SOC sorbs to phyllosilicate clays; Al, Fe, and Mn oxides; poorly crystalline minerals; and polyvalent cations that form a bridge between minerals and organic constituents (Blanco-Canqui and Lal, 2004). However, for our soils we found that, despite higher concentrations of Alo under agriculture, a lesser share of the SOC was present in the s + c fraction (as percent of SOC) compared to the pine forest soils (though only at p = 0.08). Association of OC with Alo–containing minerals could not explain the higher SOC proportion of OC in s + c under forest. As the amount of s + c OC (in g C kg−1 soil) was in fact similar between both land uses, it appears that a lower s + c SOC contribution in the agricultural soils was instead simply mirroring trends in the S + A fraction. A positive correlation between the s + c SOC proportion and Feo in s + c at ± 60–80 cm (r = 0.93; p < 0.05) could indicate that association with poorly crystalline Fe stabilizes OM in the silt and clay fraction. But, again, as s + c SOC content (g kg−1 soil) and OC concentration (g kg−1 fraction) correlated negatively at all measured depths with Feo in the s + c fraction, such land use control on s + c Feo-associated SOC seems unlikely. On the other hand, the higher SOC content (g C kg−1 soil) in the s + c fraction of NF-y compared to other soils, particularly at a depth of ± 60–80 cm, might be due to the differences in mineralogy. At this depth range, the amorphous Fe content (Feo and Feh) in NF-y was very high compared to the other soils. The s + c fraction was as per the Zimmermann et al. (2007) fractionation procedure subdivided into oxidizable and resistant parts, expected to coincide roughly with SOC stabilized by mineral association as opposed to its own biochemical inertness, respectively. Negative correlations existed between the share of s + c OC (percent of s + c OC) that was oxidizable by 6 % NaOCl (i.e., s + c − rSOC) with the contents of Alo, the bulk soil of SSA, and micropore volume (Fig. 6). These correlations suggest that the association of SOC with short-range-order Al (hydr)oxides has been found to limit oxidation of SOC by 6 % of NaOCl (Mikutta et al., 2006). It appears that geochemical properties influence the degree of mineral association of OC and its content in these Andosols. As neither chemical inertness of the s + c OC (agriculture at 53 % of s + c vs. pine forest at 59 % of s + c) nor the s + c Alo, bulk soil SSA, or micropore volume differed between both land uses, it appears that land use history did not impact the degree of mineral association of OC and its content in these Andosols. This outcome further contradicts the fact that more s + c OC would accumulate under forest because of a more biochemical inertness of the OC inputs compared to agriculture.
4.3 Land use effects on native SOC degradability
The percentage of SOC mineralized after 120 d of incubation was less than half in the agricultural soils (1.2 %) than in the pine forest soils (2.9 %) (p < 0.01). This result was rather unexpected, given the lower soil pH by 0.8 under pine forest, as acidic conditions are well known to retard decomposition of SOC (Högberg et al., 2007; Malik et al., 2018) in line with Anda and Dahlgren (2020). To understand the apparent relative stabilization of SOC in the agricultural soils, we again consider the distribution of SOC across soil fractions. We (Anindita et al., 2022) previously hypothesized that the nearly doubled Alo content of the agricultural soils (21.8 g kg−1 on average) compared to the pine forest soils (13.9 g kg−1) would partially explain the relatively elevated SOC stocks under agriculture for our study area due to enhanced organo-mineral association. Basile-Doelsch et al. (2005) likewise found that volcanic ash soil horizons containing much poorly crystalline material (proto-imogolite and proto-imogolite allophane) store large amounts of organic matter which turns over very slowly. But as no negative correlations existed between the amount or proportion of SOC mineralized and Alo content, this hypothesis was not confirmed. As mentioned above, we did find a higher percent of SOC to be present in the rSOC fraction under forest, but its content (g kg−1 soil) was not higher than under agricultural land use, and again no correlation existed with SOC mineralization. Mikutta et al. (2006) indeed demonstrated that a larger chemical recalcitrance of SOC does not really translate into a larger biological stability. Once more, it appears that the larger SOC proportion of rSOC seems to simply result from less accumulation of SOC in the S + A fraction under forest.
The proportion and concentration of the S + A fraction of OC were higher under agriculture, so enhanced soil aggregation and occlusion of SOC might have significantly physically protected SOC against microbially mediated decomposition compared to pine forest land use. A study of Andosols in Colombia by Gijsman and Sanz (1998) also reported that aggregation holds considerable control on SOC decomposability as these authors observed a significant increase in CO2 mineralization after crushing large and small macroaggregates (> 53 µm). Indeed, we found that the amount of OC (g C kg−1 soil) in the S + A fraction negatively correlated to percent SOC mineralized for the set of soils with agricultural and pine forest land use (r = −0.78; p < 0.05). It then appears that enhanced soil aggregation and occlusion of SOC under agricultural land use in part limited degradability of SOC rather than enhanced association of SOC with soil minerals, including poorly crystalline or amorphous Al-containing minerals. As argued in Sect. 4.2, we can add to this that formation of amorphous materials and Alo in the agricultural soils most likely promoted soil aggregation. Hence, we hypothesize that enhanced pedogenesis forms a relevant indirect mechanism via which conversion to agricultural land use impacts the SOC balance of these relatively young tropical volcanic soils. However, since large amounts of exogenous OM are annually being applied to the investigated agricultural fields in this study (Table 1), we cannot unequivocally exclude that these instead explain the high SOC stocks and contribution of aggregate-associated OC here, and more observations on an expanded set of sites will be required to test this hypothesis.
4.4 Land use effects of exogenous organic matter decomposition and net priming of SOC
In contrast to native SOC mineralization, added grass C mineralization did not differ between forest and agricultural soils. Therefore, apparent protection of ryegrass OC either by mineral association or occlusion inside aggregates must have been similar between both land uses and, in any case, did not impact its degradability in soil. Laboratory incubations with disturbed soils do not necessarily adequately reproduce field conditions in terms of soil structure, microclimate, and food webs. The observed similar degradability of ryegrass-C does not suggest a likewise enhanced storage of freshly added OM like for native SOC under agriculture than under forest. Possibly, such an impact only emerges in the longer term when the added OM has been subject to sufficient diminution into smaller POM that could be occluded in microaggregates. Six et al. (2002) summarized that particularly 50–250 µm scaled microaggregates grant sizable physical protection to POM, while the macroaggregate (> 250 µm) structure exerts little control on POM decomposability. Adding ryegrass to the 0–20 cm soils initially seemed to impact mineralization of native SOC with a general positive priming effect in the first week of incubation, in line with many other studies, e.g., Liu et al. (2017). Thereafter, however, in four of the six studied soils net negative priming countered this initial stimulation of SOC mineralization. Such an adverse impact of adding fresh OM on SOC mineralization could be due to a preferential utilization of the added substrate by microorganisms (Derrien et al., 2014). Regardless, the net result was that after 120 d SOC mineralization was not significantly stimulated (on average by 1.6 %). There was also no land use effect on the net priming of SOC after 120 d. Hence, in spite of differences in SOC quality under both land uses, as inferred from different SOC proportions of S + A and free POM, no differential stimulation of SOC mineralization by addition of a relatively labile plant C substrate existed between agricultural or forest land uses. These observations imply that no adverse effect is to be expected for the addition of generally relatively labile C (aboveground crop residues, animal manure) on stability of the native SOC present under agricultural land use.
4.5 Importance of physical occlusion of POM for SOC stabilization
As an important side note to the interpretation of the SOC fractionation data, it should be borne in mind that there is no guarantee that isolated SOM fractions coincide with distinct SOM pools, neither in terms of bioavailability nor intrinsic degradability. By expanding the Zimmermann et al. (2007) fractionation procedure with a strong (400 J mL−1) ultrasonic dispersion step, we attempted to isolate s + c-associated OC from POM contained in the S + A fraction. This ultrasonication step proved to result in incomplete disruption of the apparently very stable > 63 µm sized aggregates. With high levels of both OM and amorphous materials as well as poorly crystalline metal oxides, this is perhaps not surprising. The insignificant difference in the C:N ratio between the S + A and s + c fractions suggests that, while they obviously differ in their physical organization, there is probably no strong contrast in the biochemical composition of the OM. Previous studies also found no clear difference in C composition from sand to clay fractions in Andosols (Baldock et al., 1992). Regardless, the here-achieved subdivision of the SOM into OM present inside (S + A) or not inside (s + c) the microaggregate structure would yet still appear relevant as the S + A SOC proportion correlated negatively with SOC mineralization, suggesting indeed that such a physical organization of soil grants “physical protection” against microbial breakdown, thought to result from reduced accessibility of microbes, exoenzymes, and/or e-acceptors (Keil and Mayer, 2013; Lehmann et al., 2007). The overall rather low C:N ratio of the S + A fraction (13.0 ± 4.2) indicates that it mainly contained mineral-associated OM and probably little POM, complicating interpretation of mechanisms for a particular S + A SOC storage in agricultural sites. Still, in the 0–20 cm layer, a higher S + A fraction C:N ratio of the agriculture (17.0) vs. pine forest (11.0) S + A fraction suggests that, at least to some extent, enhanced SOC storage in aggregates under agricultural land use might have resulted from extra occlusion of POM. However, our data do not provide unequivocal proof that the physical protection of SOM in the S + A fraction causes relative protection against mineralization. In addition, the absence of any land use effect on protection of freshly added plant litter (see Sect. 4.4) leads us to question this. Extra aggregation of OM into the S + A fraction in the agricultural soils might just be but a concomitant effect of the larger presence of tightly intermixed organometallic phases, while such smaller-scaled structures themselves are instead zones of SOC stabilization. On the other hand, the fact that there was no negative correlation between Alo or Feo and the relative SOC mineralization, while Alo did positively relate to the S + A SOC proportion, would instead suggest that indeed the physical arrangement of OM into stable aggregates led to a relative stabilization of SOC. From the limited presence of POM in the S + A fraction, it logically emerges that mainly occlusion of smaller-sized OM and its protection into aggregates was important. To further elucidate this, however, more detailed investigation of the formation of organomineral phases and their organization into the microstructure is required, building on the recently forwarded organometallic glue hypothesis and proposed methodologies by Wagai et al. (2020).
Following the fractionation of SOC by Zimmermann et al. (2007), the silt- and clay-associated OC forms the dominant SOC pool in tropical volcanic soils in Indonesia, regardless of land use. But land use strongly impacts SOC contained within ultrasonic-dispersion-resistant (400 J mL−1) soil aggregates as its contribution to SOC rose from 8 % of SOC in forest to as much as 32 % of SOC several decades after conversion to agricultural use, with, alongside also, more OC stored in this fraction. The S + A SOC proportion was positively related to Alo and the percent of amorphous materials. Our study thus indicates that the larger abundance of Alo in agricultural soils promoted soil aggregation and physical occlusion of OC compared to pine forest. A negative correlation between relative decomposability of the SOC and the portion of SOC that is physically occluded further suggests that enhanced soil aggregation under cropland effectively stabilizes part of the SOC compared to under forest land use. Contrary to our hypothesis, however, we found no proof that stimulated formation of Alo and amorphous minerals would have increased association of SOC with soil minerals under agriculture. Based on the present study, we hypothesize that enhanced formation of amorphous minerals and Alo under agriculture with high OM inputs promoted the development of stable soil aggregates and OC occlusion therein, and this would in part counter otherwise expected losses of SOC compared to primary and secondary forest. However, the contribution of large OM inputs vs. land use conversion, per se, could not be elucidated here, and this will require study of other tropical Andosol forest–agricultural land use pairs with a detailed inventory of OC inputs. Moreover, confirmation that agricultural land use promotes Alo formation vs. forest will require time series analysis of such sites. At least, our study points at the overall need to account for potential indirect land use effects on the stability of SOC via its control on pedogenesis. This is especially so for pedoclimatic combinations where weathering can be very fast, like in tropical Andosols that have only relatively recently been cultivated.
The data generated in this study are available from the corresponding author upon reasonable request.
The supplement related to this article is available online at: https://doi.org/10.5194/soil-9-443-2023-supplement.
SS, PF, and SA conceptualized the study. SA conducted soil sampling and performed the experiment. SA prepared the manuscript with contributions from all the co-authors.
At least one of the (co-)authors is a member of the editorial board of SOIL. The peer-review process was guided by an independent editor, and the authors also have no other competing interests to declare.
Publisher's note: Copernicus Publications remains neutral with regard to jurisdictional claims in published maps and institutional affiliations.
We thank the Indonesia Endowment Fund for Education (LPDP) for the internal support of this research, as well as Orly Mendoza, Haichao Li, and Heleen Dero from the Soil Fertility Research Group (Ghent University) for laboratory assistance.
This research has been supported by the Indonesia Endowment Fund for Education (LPDP).
This paper was edited by Karsten Kalbitz and reviewed by three anonymous referees.
Anda, M. and Dahlgren, R. A.: Long-term response of tropical Andisol properties to conversion from rainforest to agriculture, Catena, 194, 104679, https://doi.org/10.1016/j.catena.2020.104679, 2020.
Anindita, S., Sleutel, S., Vandenberghe, D., Grave, J. De, Vandenhende, V., and Finke, P.: Land use impacts on weathering, soil properties, and carbon storage in wet Andosols, Indonesia, Geoderma, 423, 115963, https://doi.org/10.1016/j.geoderma.2022.115963, 2022.
Asano, M. and Wagai, R.: Evidence of aggregate hierarchy at micro- to submicron scales in an allophanic andisol, Geoderma, 216, 62–74, https://doi.org/10.1016/j.geoderma.2013.10.005, 2014.
Asano, M. and Wagai, R.: Distinctive organic matter pools among particle-size fractions detected by solid-state 13C-NMR, δ13C and δ15N analyses only after strong dispersion in an allophanic andisol, Soil Sci. Plant Nutr., 61, 242–248, https://doi.org/10.1080/00380768.2014.982492, 2015.
Asano, M., Wagai, R., Yamaguchi, N., Takeichi, Y., Maeda, M., Suga, H., and Takahashi, Y.: In search of a binding agent: Nano-scale evidence of preferential carbon associations with poorly-crystalline mineral phases in physically-stable, clay-sized Aggregates, Soil Syst., 2, 32, https://doi.org/10.3390/soilsystems2020032, 2018.
Baldock, J. A.: Composition and Cycling of Organic Carbon in Soil, in: Nutrient Cycling in Terrestrial Ecosystems, edited by: Marschner, P. and Rengel, Z., Springer Berlin, Heidelberg, 1–35, https://doi.org/10.1007/978-3-540-68027-7_1, 2007.
Baldock, J. A., Oades, J. M., Waters, A. G., Peng, X., Vassallo, A. M., and Wilson, M. A.: Aspects of the chemical structure of soil organic materials as revealed by solid-state13C NMR spectroscopy, Biogeochemistry, 16, 1–42, https://doi.org/10.1007/bf00024251, 1992.
Bartoli, F., Burtin, G., and Herbillon, A. J.: Disaggregation and clay dispersion of Oxisols: Na resin, a recommended methodology, Geoderma, 49, 301–317, https://doi.org/10.1016/0016-7061(91)90082-5, 1991.
Basile-Doelsch, I., Amundson, R., Stone, W. E. E., Masiello, C. A., Bottero, J. Y., Colin, F., Masin, F., Borschneck, D., and Meunier, J. D.: Mineralogical control of organic carbon dynamics in a volcanic ash soil on La Réunion, Eur. J. Soil Sci., 56, 689–703, https://doi.org/10.1111/j.1365-2389.2005.00703.x, 2005.
Basile-Doelsch, I., Amundson, R., Stone, W. E. E., Borschneck, D., Bottero, J. Y., Moustier, S., Masin, F., and Colin, F.: Mineral control of carbon pools in a volcanic soil horizon, Geoderma, 137, 477–489, https://doi.org/10.1016/j.geoderma.2006.10.006, 2007.
Berg, B. and McClaugherty, C.: Plant litter: decomposition, humus formation, carbon sequestration, 3rd Edn., Springer Berlin Heidelberg, Berlin, Heidelberg, https://doi.org/10.1007/978-3-662-05349-2, 2003.
Besnard, E., Chenu, C., Balesdent, J., Puget, P., and Arrouays, D.: Fate of particulate organic matter in soil aggregates during cultivation, Eur. J. Soil Sci., 47, 495–503, https://doi.org/10.1111/j.1365-2389.1996.tb01849.x, 1996.
Blagodatskaya, E., Khomyakov, N., Myachina, O., Bogomolova, I., Blagodatsky, S., and Kuzyakov, Y.: Microbial interactions affect sources of priming induced by cellulose, Soil Biol. Biochem., 74, 39–49, https://doi.org/10.1016/j.soilbio.2014.02.017, 2014.
Blanco-Canqui, H. and Lal, R.: Mechanisms of carbon sequestration in soil aggregates, CRC Cr. Rev. Plant Sci., 23, 481–504, https://doi.org/10.1080/07352680490886842, 2004.
Borggaard, O. K., Jørgensen, S. S., Møberg, J. P., and Raben-Lange, B.: Influence of organic matter on phosphate adsorption by aluminium and iron oxides in sandy soils, J. Soil Sci., 41, 443–449, https://doi.org/10.1111/j.1365-2389.1990.tb00078.x, 1990.
Bruijnzeel, L. A.: Nutrient Content of Litterfall in Coniferous Forest Plantations in Central Java, Indonesia, J. Trop. Ecol., 1, 353–372, http://www.jstor.org/stable/2559453 (last access: 6 April 2016), 1985.
Cerli, C., Celi, L., Kalbitz, K., Guggenberger, G., and Kaiser, K.: Separation of light and heavy organic matter fractions in soil – Testing for proper density cut-off and dispersion level, Geoderma, 170, 403–416, https://doi.org/10.1016/j.geoderma.2011.10.009, 2012.
Chen, R., Senbayram, M., Blagodatsky, S., Myachina, O., Dittert, K., Lin, X., Blagodatskaya, E., and Kuzyakov, Y.: Soil C and N availability determine the priming effect: Microbial N mining and stoichiometric decomposition theories, Glob. Change Biol., 20, 2356–2367, https://doi.org/10.1111/gcb.12475, 2014.
Churchman, G. J. and Lowe, D. J.: Alteration, formation, and occurrence of minerals in soils, in: Handbook of Soil Sciences, Vol. 1, edited by: Huang, P. M., Li, Y., and Sumner, M. E., CRC Press (Taylor & Francis), Boca Raton, FL, 20.1–20.72, https://doi.org/10.1201/b11267, 2012.
Covaleda, S., Gallardo, J. F., García-Oliva, F., Kirchmann, H., Prat, C., Bravo, M., and Etchevers, J. D.: Land-use effects on the distribution of soil organic carbon within particle-size fractions of volcanic soils in the Transmexican Volcanic Belt (Mexico), Soil Use Manage., 27, 186–194, https://doi.org/10.1111/j.1475-2743.2011.00341.x, 2011.
Cronan, C. S.: Ecosystem Biogeochemistry, Springer, Orono, USA, https://doi.org/10.1007/978-3-319-66444-6, 2018.
Cusack, D. F., Chadwick, O. A., Ladefoged, T., and Vitousek, P. M.: Long-term effects of agriculture on soil carbon pools and carbon chemistry along a Hawaiian environmental gradient, Biogeochemistry, 112, 229–243, https://doi.org/10.1007/s10533-012-9718-z, 2013.
De Clercq, T., Heiling, M., Dercon, G., Resch, C., Aigner, M., Mayer, L., Mao, Y., Elsen, A., Steier, P., Leifeld, J., and Merckx, R.: Predicting soil organic matter stability in agricultural fields through carbon and nitrogen stable isotopes, Soil Biol. Biochem., 88, 29–38, https://doi.org/10.1016/j.soilbio.2015.05.011, 2015.
Derrien, D., Plain, C., Courty, P. E., Gelhaye, L., Moerdijk-Poortvliet, T. C. W., Thomas, F., Versini, A., Zeller, B., Koutika, L. S., Boschker, H. T. S., and Epron, D.: Does the addition of labile substrate destabilise old soil organic matter?, Soil Biol. Biochem., 76, 149–160, https://doi.org/10.1016/j.soilbio.2014.04.030, 2014.
Doetterl, S., Stevens, A., Six, J., Merckx, R., Van Oost, K., Casanova Pinto, M., Casanova-Katny, A., Muñoz, C., Boudin, M., Zagal Venegas, E., and Boeckx, P.: Soil carbon storage controlled by interactions between geochemistry and climate, Nat. Geosci., 8, 780–783, https://doi.org/10.1038/ngeo2516, 2015.
Dörner, J., Dec, D., Feest, E., Vásquez, N., and Díaz, M.: Dynamics of soil structure and pore functions of a volcanic ash soil under tillage, Soil Till. Res., 125, 52–60, https://doi.org/10.1016/j.still.2012.05.019, 2012.
Don, A., Schumacher, J., and Freibauer, A.: Impact of tropical land-use change on soil organic carbon stocks - a meta-analysis, Glob. Change Biol., 17, 1658–1670, https://doi.org/10.1111/j.1365-2486.2010.02336.x, 2011.
Du, Z. L., Wu, W. L., Zhang, Q. Z., Guo, Y. B., and Meng, F. Q.: Long-Term Manure Amendments Enhance Soil Aggregation and Carbon Saturation of Stable Pools in North China Plain, J. Integr. Agr., 13, 2276–2285, https://doi.org/10.1016/S2095-3119(14)60823-6, 2014.
Dungait, J. A. J., Hopkins, D. W., Gregory, A. S., and Whitmore, A. P.: Soil organic matter turnover is governed by accessibility not recalcitrance, Glob. Change Biol., 18, 1781–1796, https://doi.org/10.1111/j.1365-2486.2012.02665.x, 2012.
Gerzabek, M. H., Bajraktarevic, A., Keiblinger, K., Mentler, A., Rechberger, M., Tintner, J., Wriessnig, K., Gartner, M., Valenzuela, X. S., Troya, A., Couenberg, P. M., Jäger, H., Carrión, J. E., and Zehetner, F.: Agriculture changes soil properties on the Galápagos Islands-two case studies, Soil Res., 57, 201–214, https://doi.org/10.1071/SR18331, 2019.
Gijsman, A. J. and Sanz, J. I.: Soil organic matter pools in a volcanic-ash soil under fallow or cultivation with applied chicken manure, Eur. J. Soil Sci., 49, 427–436, https://doi.org/10.1046/j.1365-2389.1998.4930427.x, 1998.
Guillaume, T., Kotowska, M. M., Hertel, D., Knohl, A., Krashevska, V., Murtilaksono, K., Scheu, S., and Kuzyakov, Y.: Carbon costs and benefits of Indonesian rainforest conversion to plantations, Nat. Commun., 9, 2388, https://doi.org/10.1038/s41467-018-04755-y, 2018.
Hernández, Z., Almendros, G., Carral, P., Álvarez, A., Knicker, H., and Pérez-Trujillo, J. P.: Influence of non-crystalline minerals in the total amount, resilience and molecular composition of the organic matter in volcanic ash soils (Tenerife Island, Spain), Eur. J. Soil Sci., 63, 603–615, https://doi.org/10.1111/j.1365-2389.2012.01497.x, 2012.
Hertel, D., Moser, G., Culmsee, H., Erasmi, S., Horna, V., Schuldt, B., and Leuschner, C.: Below- and above-ground biomass and net primary production in a paleotropical natural forest (Sulawesi, Indonesia) as compared to neotropical forests, Forest Ecol. Manag., 258, 1904–1912, https://doi.org/10.1016/j.foreco.2009.07.019, 2009.
Högberg, M. N., Högberg, P., and Myrold, D. D.: Is microbial community composition in boreal forest soils determined by pH, C-to-N ratio, the trees, or all three?, Oecologia, 150, 590–601, https://doi.org/10.1007/s00442-006-0562-5, 2007.
Huygens, D., Boeckx, P., Van Cleemput, O., Oyarzún, C., and Godoy, R.: Aggregate and soil organic carbon dynamics in South Chilean Andisols, Biogeosciences, 2, 159–174, https://doi.org/10.5194/bg-2-159-2005, 2005.
Kang, J., Hesterberg, D., and Osmond, D. L.: Soil Organic Matter Effects on Phosphorus Sorption: A Path Analysis, Soil Sci. Soc. Am. J., 73, 360–366, https://doi.org/10.2136/sssaj2008.0113, 2009.
Keeling, D.: The concentration and isotopic abundances of atmospheric carbon dioxide in rural areas, Geochim. Cosmochim. Ac., 13, 322–334, https://doi.org/10.1016/0016-7037(58)90033-4, 1958.
Keil, R. G. and Mayer, L. M.: Mineral Matrices and Organic Matter, edited by: Holland, H. D. and Turekian, K. K., 2nd Edn., Elsevier Ltd., https://doi.org/10.1016/B978-0-08-095975-7.01024-X, 2013.
Kleber, M., Eusterhues, K., Keiluweit, M., Mikutta, C., Mikutta, R., and Nico, P. S.: Mineral-Organic Associations: Formation, Properties, and Relevance in Soil Environments, in: Advances in Agronomy, Vol. 130, edited by: Sparks, D. L., Elsevier Ltd., 1–140, https://doi.org/10.1016/bs.agron.2014.10.005, 2015.
Lehmann, J. and Kleber, M.: The contentious nature of soil organic matter, Nature, 528, 60–68, https://doi.org/10.1038/nature16069, 2015.
Lehmann, J., Kinyangi, J., and Solomon, D.: Organic matter stabilization in soil microaggregates: Implications from spatial heterogeneity of organic carbon contents and carbon forms, Biogeochemistry, 85, 45–57, https://doi.org/10.1007/s10533-007-9105-3, 2007.
Li, J., Du, J., Zhong, S., Ci, E., and Wei, C.: Changes in the profile properties and chemical weathering characteristics of cultivated soils affected by anthropic activities, Sci. Rep.-UK, 11, 20822, https://doi.org/10.1038/s41598-021-00302-w, 2021.
Linlin, G., Taku, N., Hiromi, I., and Zhigang, S.: Carbon Mineralization Associated with Soil Aggregates as Affected by Short-Term Tillage, J. Resour. Ecol., 7, 101–106, https://doi.org/10.5814/j.issn.1674-764x.2016.02.004, 2016.
Liu, X. J. A., Sun, J., Mau, R. L., Finley, B. K., Compson, Z. G., van Gestel, N., Brown, J. R., Schwartz, E., Dijkstra, P., and Hungate, B. A.: Labile carbon input determines the direction and magnitude of the priming effect, Appl. Soil Ecol., 109, 7–13, https://doi.org/10.1016/j.apsoil.2016.10.002, 2017.
Lyu, H., Watanabe, T., Kilasara, M., Hartono, A., and Funakawa, S.: Soil organic carbon pools controlled by climate and geochemistry in tropical volcanic regions, Sci. Total Environ., 761, 143277, https://doi.org/10.1016/j.scitotenv.2020.143277, 2021.
Malik, A. A., Puissant, J., Buckeridge, K. M., Goodall, T., Jehmlich, N., Chowdhury, S., Gweon, H. S., Peyton, J. M., Mason, K. E., van Agtmaal, M., Blaud, A., Clark, I. M., Whitaker, J., Pywell, R. F., Ostle, N., Gleixner, G., and Griffiths, R. I.: Land use driven change in soil pH affects microbial carbon cycling processes, Nat. Commun., 9, 1–10, https://doi.org/10.1038/s41467-018-05980-1, 2018.
Matus, F., Rumpel, C., Neculman, R., Panichini, M., and Mora, M. L.: Soil carbon storage and stabilisation in andic soils: A review, Catena, 120, 102–110, https://doi.org/10.1016/j.catena.2014.04.008, 2014.
McKeague, J. A.: An evaluation of 0.1 M pyrophosphate and pyrophosphate-dithionite in comparison with oxalate as extractants of the accumulation products in podzols and some other soils, Can. J. Soil Sci., 47, 95–99, https://doi.org/10.4141/cjss67-017, 1967.
Mikha, M. M. and Rice, C. W.: Tillage and Manure Effects on Soil and Aggregate-Associated Carbon and Nitrogen, Soil Sci. Soc. Am. J., 68, 809–816, https://doi.org/10.2136/sssaj2004.8090, 2004.
Mikutta, R., Kleber, M., Torn, M. S., and Jahn, R.: Stabilization of soil organic matter: Association with minerals or chemical recalcitrance?, Biogeochemistry, 77, 25–56, https://doi.org/10.1007/s10533-005-0712-6, 2006.
Mikutta, R., Schaumann, G. E., Gildemeister, D., Bonneville, S., Kramer, M. G., Chorover, J., Chadwick, O. A., and Guggenberger, G.: Biogeochemistry of mineral-organic associations across a long-term mineralogical soil gradient (0.3–4100 kyr), Hawaiian Islands, Geochim. Cosmochim. Ac., 73, 2034–2060, https://doi.org/10.1016/j.gca.2008.12.028, 2009.
Miyazawa, M., Takahashi, T., Sato, T., Kanno, H., and Nanzyo, M.: Factors controlling accumulation and decomposition of organic carbon in humus horizons of Andosols: A case study for distinctive non-allophanic Andosols in northeastern Japan, Biol. Fert. Soils, 49, 929–938, https://doi.org/10.1007/s00374-013-0792-8, 2013.
Oades, J. and Waters, A.: Aggregates hierarchy in soils, Austr. J. Soil Res., 29, 815–825, https://doi.org/10.1071/SR9910815, 1991.
Parfitt, R. L.: Allophane and imogolite: role in soil biogeochemical processes, Clay Miner., 44, 135–155, https://doi.org/10.1180/claymin.2009.044.1.135, 2009.
Poeplau, C., Don, A., Dondini, M., Leifeld, J., Nemo, R., Schumacher, J., Senapati, N., and Wiesmeier, M.: Reproducibility of a soil organic carbon fractionation method to derive RothC carbon pools, Eur. J. Soil Sci., 64, 735–746, https://doi.org/10.1111/ejss.12088, 2013.
Poirier, V., Basile-Doelsch, I., Balesdent, J., Borschneck, D., Whalen, J. K., and Angers, D. A.: Organo-Mineral Interactions Are More Important for Organic Matter Retention in Subsoil Than Topsoil, Soil Syst., 4, 4, https://doi.org/10.3390/soilsystems4010004, 2020.
Qiao, N., Schaefer, D., Blagodatskaya, E., Zou, X., Xu, X., and Kuzyakov, Y.: Labile carbon retention compensates for CO2 released by priming in forest soils, Glob. Change Biol., 20, 1943–1954, https://doi.org/10.1111/gcb.12458, 2014.
Rabbi, S. M. F., Daniel, H., Lockwood, P. V., Macdonald, C., Pereg, L., Tighe, M., Wilson, B. R., and Young, I. M.: Physical soil architectural traits are functionally linked to carbon decomposition and bacterial diversity, Sci. Rep.-UK, 6, 33012, https://doi.org/10.1038/srep33012, 2016.
Rennert, T.: Wet-chemical extractions to characterise pedogenic Al and Fe species –a critical review, Soil Res., 57, 1–16, https://doi.org/10.1071/SR18299, 2019.
Six, J., Elliott, E. T., and Paustian, K.: Soil macroaggregate turnover and microaggregate formation: A mechanism for C sequestration under no-tillage agriculture, Soil Biol. Biochem., 32, 2099–2103, https://doi.org/10.1016/S0038-0717(00)00179-6, 2000a.
Six, J., Paustian, K., Elliott, E. T., and Combrink, C.: Soil Structure and Organic Matter I. Distribution of Aggregate-Size Classes and Aggregate-Associated Carbon, Soil Sci. Soc. Am. J., 64, 681–689, https://doi.org/10.2136/sssaj2000.642681x, 2000b.
Six, J., Conant, R. T., Paul, E. A., and Paustian, K.: Stabilization mechanisms of soil organic matter: Implications for C-saturation of soils, Plant Soil, 241, 155–176, https://doi.org/10.1023/A:1016125726789, 2002.
Smith, W. K., Cleveland, C. C., Reed, S. C., and Running, S. W.: Agricultural conversion without external water and nutrient inputs reduces terrestrial vegetation productivity, Geophys. Prospect., 41, 449–455, https://doi.org/10.1002/2013GL058857, 2014.
SSB and UGhent (The Soil Service of Belgium and Ghent University): Study LA BOD/STUD 2006 01 04 for the Flemish Government – Development of expertsystem for C-management advise for cropland soils: part 1 literature study & part 2 development of an advice system, Dept. Environment, Nature and Energy of the Flemish Government, Brussels, https://publicaties.vlaanderen.be/view-file/20207 (last access: 28 July 2023), 2008 (in Dutch).
Tamrat, W. ., Rose, J., Grauby, O., Doelsch, E., Levard, C., Chaurand, P., and Basile-Doelsch, I.: Soil organo-mineral associations formed by co-precipitation of Fe, Si and Al in presence of organic ligands, Geochim. Cosmochim. Ac., 260, 15–28, https://doi.org/10.1016/j.gca.2019.05.043, 2019.
Taylor, M. D., Lowe, D. J., Hardi, P., Smidt, G. A., and Schnug, E.: Comparing volcanic glass shards in unfertilised and fertilised Andisols derived from rhyolitic tephras, New Zealand: Evidence for accelerated weathering and implications for land management, Geoderma, 271, 91–98, https://doi.org/10.1016/j.geoderma.2016.01.035, 2016.
Van Breemen, N., Mulder, J., and Driscoll, C.: Acidification and alkalinization of soils, Plant Soil, 75, 283–308, http://www.jstor.com/stable/42934465 (last access: 9 April 2021), 1983.
Wagai, R., Kajiura, M., Uchida, M., and Asano, M.: Distinctive roles of two aggregate binding agents in allophanic andisols: Young carbon and poorly-crystalline metal phases with old carbon, Soil Syst., 2, 1–23, https://doi.org/10.3390/soilsystems2020029, 2018.
Wagai, R., Kajiura, M., and Asano, M.: Iron and aluminum association with microbially processed organic matter via meso-density aggregate formation across soils: organo-metallic glue hypothesis, SOIL, 6, 597–627, https://doi.org/10.5194/soil-6-597-2020, 2020.
Wei, X., Shao, M., Gale, W., and Li, L.: Global pattern of soil carbon losses due to the conversion of forests to agricultural land, Sci. Rep.-UK, 4, 6–11, https://doi.org/10.1038/srep04062, 2014.
Werth, M. and Kuzyakov, Y.: 13C fractionation at the root-microorganisms-soil interface: A review and outlook for partitioning studies, Soil Biol. Biochem., 42, 1372–1384, https://doi.org/10.1016/j.soilbio.2010.04.009, 2010.
Yu, H., Ding, W., Luo, J., Geng, R., Ghani, A., and Cai, Z.: Effects of long-term compost and fertilizer application on stability of aggregate-associated organic carbon in an intensively cultivated sandy loam soil, Biol. Fert. Soils, 48, 325–336, https://doi.org/10.1007/s00374-011-0629-2, 2012.
Zheng, H., Liu, W., Zheng, J., Luo, Y., Li, R., Wang, H., and Qi, H.: Effect of long-term tillage on soil aggregates and aggregate-associated carbon in black soil of northeast China, PLOS ONE, 13, 1–18, https://doi.org/10.1371/journal.pone.0199523, 2018.
Zimmermann, M., Leifeld, J., Schmidt, M. W. I., Smith, P., and Fuhrer, J.: Measured soil organic matter fractions can be related to pools in the RothC model, Eur. J. Soil Sci., 58, 658–667, https://doi.org/10.1111/j.1365-2389.2006.00855.x, 2007.