the Creative Commons Attribution 4.0 License.
the Creative Commons Attribution 4.0 License.
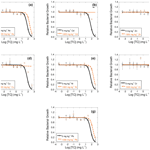
Tolerance of soil bacterial community to tetracycline antibiotics induced by As, Cd, Zn, Cu, Ni, Cr, and Pb pollution
Vanesa Santás-Miguel
Avelino Núñez-Delgado
Esperanza Álvarez-Rodríguez
Montserrat Díaz-Raviña
Manuel Arias-Estévez
David Fernández-Calviño
The widespread use of both heavy metals and antibiotics in livestock farming, followed by their subsequent arrival on agricultural soils through manure and slurry spreading, has become a problem of vital importance for human health and the environment. In the current research, a laboratory experiment was carried out for 42 d to study tolerance and co-tolerance of three tetracycline antibiotics (tetracycline, TC; oxytetracycline, OTC; chlortetracycline, CTC) in soils polluted with heavy metals (As, Cd, Zn, Cu, Ni, Cr, and Pb) at high concentrations (1000 mg kg−1 of each one, separately). Pollution induced community tolerance (PICT) of the bacterial community was estimated using the leucine incorporation technique. The log IC50 (logarithm of the concentration causing 50 % inhibition in bacterial community growth) values obtained in uncontaminated soil samples for all the heavy metals tested showed the following toxicity sequence: Cu > As > Cr ≥ Pb ≥ Cd > Zn > Ni. However, in polluted soil samples the toxicity sequence was Cu > Pb ≥ As ≥ Cd ≥ Cr ≥ Ni ≥ Zn. Moreover, at high heavy metal concentrations, the bacterial communities showed tolerance to the metal itself, this taking place in the long term for all the metals tested. The bacterial communities of the soil polluted with heavy metals showed also long-term co-tolerance to TC, OTC, and CTC. This kind of study, focusing on the eventual increases of tolerance and co-tolerance of bacterial communities in agricultural soil, favored by the presence of different kinds of pollutants, is of crucial importance, mostly bearing in mind that the appearance of antibiotic resistance genes in soil bacteria could be transmitted to human pathogens.
- Article
(820 KB) - Full-text XML
-
Supplement
(346 KB) - BibTeX
- EndNote
The accumulation of heavy metals in soils is a widespread problem all over the world. Some anthropogenic activities, such as mining, industrial production, agriculture, or livestock farming, are among the main sources of release of heavy metals to the terrestrial environment (Abdu et al., 2017). The use of heavy metals in livestock production is a common practice, since commercial feeds are enriched with essential elements (such as Cu and Zn) to prevent diseases and obtain an optimal growth rate (Sager, 2007; Wu et al., 2013). In fact, the presence of high heavy metal concentrations in swine manure has been reported by Hölzel et al. (2012), specifically for Zn (up to 8239 mg kg−1) and for Cu (up to 3387 mg kg−1). In addition, Wang et al. (2013) found up to 1602 mg kg−1 for Cr in layer manure, while Zhang et al. (2012) detected up to 300 mg kg−1 for Pb and up to 100 mg kg−1 for Ni in poultry manure. Finally, Liu et al. (2020) reported up to 60 mg kg−1 for Cd and up to 89 mg kg−1 for As in pig manure. In view of that, these and other heavy metals are considered a serious problem for public health, especially because these toxic elements tend to accumulate in soils, where they may reach high and progressively increasing concentrations (Kabata-Pendias, 2000; Huang et al., 2007). Moreover, veterinary antibiotics are also widely used for the treatment of bacterial infections (Boxall et al., 2003), and their consumption has been increasing in recent decades, with the estimate of world use for 2030 being 105 596 t (Van Boeckel et al., 2015). The antibiotics most widely used in veterinary medicine in the European Union are tetracyclines (TCs), and specifically tetracycline (TC), oxytetracycline (OTC), and chlortetracycline (CTC) (European Medicines Agency, 2016). Both heavy metals and antibiotics for veterinary use are poorly absorbed by the intestines of animals, causing a high percentage of them to be excreted in feces and urine (Kornegay et al., 1976; Sarmah et al., 2006). In this sense, it has been reported that tetracycline antibiotic concentrations in manure may reach values as high as 746 mg kg−1 for CTC (Pan et al., 2011), 211 mg kg−1 for OTC, and 300 mg kg−1 for TC (Widyasari-Mehta et al., 2016). The presence of high levels of heavy metals and veterinary antibiotics in farmland soils is due to the repeated applications of manure and slurries (as well as sewage sludge) as organic fertilizers (Hamscher et al., 2002; Nicholson et al., 2003). Once in the soil, these compounds can interact with soil microbial communities and modify their structure and function (Hattori, 1992; Thiele-Bruhn and Beck, 2005; Chien et al., 2008; Giller et al., 2009; Caban et al., 2018).
The increase of the concentration of any pollutant in soils may suppose a selection pressure for soil bacterial communities, causing tolerance to that pollutant (Blanck, 2002). This effect may be useful to quantify the harmful repercussions produced by pollutants on soil bacteria and is called pollution-induced community tolerance (PICT). Agricultural soils, highly influenced by anthropogenic activities, deserve special attention since they are recognized as the largest reservoirs of antibiotic-resistant genes, receiving antibiotics from veterinary use (through repeated applications of manure and slurries, as indicated above), as well as heavy metals (Ji et al., 2012). Therefore, the resistance of soil bacterial communities to antibiotics has become a crucial threat at a world scale, and the study of whether bacterial communities generate co-tolerance to antibiotics in the presence of heavy metals (as well as of how and to which degree it is developed) is of vital importance.
Several previous studies have focused on co-tolerance among different heavy metals and antibiotics (Berg et al., 2010; Sarma et al., 2010; Fernández-Calviño and Bååth, 2013; Song et al., 2017; Santás-Miguel et al., 2020a; Zhong et al., 2021). Specifically, Fernández-Calviño and Bååth (2013) evaluated the tolerance of soil bacterial community to three antibiotics (vancomycin, tetracycline, and tylosin) in one soil polluted with different Cu concentrations (2–32 mmol Cu kg−1) and observed an increase in the co-tolerance of soil bacterial communities to tetracycline (at ≥ 8 mmol Cu kg−1), tylosin (at ≥ 16 mmol Cu kg−1), and vancomycin (at ≥ 16 mmol Cu kg−1). Song et al. (2017) assessed the effects of adding different Cu and Zn concentrations (33–1000 and 165–5000 mg kg−1, respectively) on the tolerance of soil bacterial communities to tetracycline, finding that the co-tolerance to the antibiotic significantly increased in soils where Cu concentrations were ≥ 365 mg kg−1, and Zn concentration was ≥ 264 mg kg−1. Zhong et al. (2021) studied the tolerance to tetracycline and vancomycin in 10 mine soil samples with the presence of Cu (361–4399 mg kg−1), Zn (33–3811 mg kg−1), and Pb (195–20 239 mg kg−1). These authors found that, in general, there were increases in tolerance of soil bacterial communities to antibiotics, although they did not detect a systematic pattern in the co-tolerance to tetracycline associated with heavy metal concentrations, whereas the induced levels of heavy metal tolerance coincided with elevated levels of tolerance to vancomycin. However, until now, there have been no studies evaluating the effect of a wide range of different heavy metals present in livestock manure on the tolerance shown by soil bacterial communities against each of the three tetracycline antibiotics most used in animal husbandry. Therefore, the objective of this study is to determine the eventual development of tolerance in soil bacterial communities to heavy metals in agricultural soils contaminated individually with 1000 mg kg−1 of As, Cd, Zn, Cu, Ni, Cr, and Pb and also the eventual generation of co-tolerance to the antibiotics tetracycline, oxytetracycline, and chlortetracycline, using the leucine incorporation technique as the endpoint. Previous works using Cu and/or Zn showed that the heavy metal concentrations needed to induce bacterial community tolerance to antibiotics in soils are high, generally ≥ 1000 mg kg−1 (Fernández-Calviño and Bååth, 2013; Song et al., 2017). Although these values are not very frequent, they could accumulate after enough time has elapsed, since heavy metals are not degraded in soils. In this regard, Mirlean et al. (2007) found Cu concentrations higher than 1000 mg kg−1 in Brazilian vineyard soils. Therefore, the results of the current research could be relevant in order to define appropriate management practices for waste and fertilizers in agricultural soils.
2.1 Chemicals
Tetracycline hydrochloride (CAS 64-75-5; ≥ 95 % in purity), oxytetracycline hydrochloride (CAS 2058-46-0; ≥ 95 % in purity), and chlortetracycline hydrochloride (CAS 64-72-2; ≥ 97 % in purity), all three supplied by Sigma-Aldrich (Steinheim, Germany), were used for soil spiking.
The heavy metals added to the soil samples for determining bacterial community tolerance were arsenic (as Na2HAsO4 7H2O, CAS; 10048-95-0), cadmium (as Cd(NO3)2 4H2O, CAS 10022-68-1), zinc (as Zn(NO3)2 6H2O, CAS; 10196-18-6), copper (as Cu(NO3)2 2.5H2O, CAS 19004-19-4), nickel (as Ni(NO3)2 6H2O, CAS 13478-00-7), chromium (as K2Cr2O7, CAS 7778-60-9), and lead (as Pb(NO3)2, CAS 10099-74-8), all of them supplied by Panreac (Barcelona, Spain).
2.2 Soil samples
An agricultural soil from Sarria (NW of Spain) was selected from a set of soils previously analyzed by Conde-Cid et al. (2018). Total concentrations of Na, K, Ca, Mg, Al, Fe, and Mn, as well as of As, Cd, Cr, Cu, Ni, Pb, and Zn, were determined using inductively coupled plasma mass spectrometry (ICP-MS; 820-NS, Varian, Palo Alto, CA, USA), which was carried out after performing a microwave-assisted digestion using 65 % nitric acid. As, Cd, Cr, Cu, Ni, Pb, and Zn bioavailability at 0 and 42 d of incubation was assessed performing extractions with 0.01 M CaCl2 (Novozamsky et al., 1993) and with EDTA (ethylenediamine tetra-acetic acid) (Lakanen and Erviö, 1971). The electrical conductivity (EC) was analyzed using distilled water for extraction, with 1.5 as the soil : water ratio, and measuring in a conductivity meter. Moreover, this soil was previously used for measurement of the bacterial growth after being polluted with antibiotics (tetracycline, oxytetracycline, and chlortetracycline), with results shown in Santás-Miguel et al. (2020b) and Santás-Miguel et al. (2020c).
The main characteristics of the soil studied are shown in Table S1 (Supplement). Briefly, its texture was silt loam, with pH in water of 6.0 and pH in KCl (0.1 M) of 5.2. The organic carbon (C) and total nitrogen (N) contents were 1.8 % and 0.2 %, respectively, and the effective cation exchange capacity (eCEC) was 13.2 cmolc kg−1. The electrical conductivity value was 298.0 µS cm−1. The total contents (in mg kg−1) for the determined heavy metals were the following: CrT 43.4, NiT 25.4, CuT 43.4, AsT 27.5, CdT < detection limit, PbT 17.1, and ZnT 135.5. These values were similar to those found in non-polluted soils in the study area (Macías and Calvo, 2008).
2.3 Experimental design
The agricultural soil was polluted, separately, with 1000 mg kg−1 of As, Cd, Zn, Cu, Ni, Cr, and Pb. The procedure was as follows: an amount of 90 g of the air-dried soil was placed in a polypropylene tube (500 mL) and then moistened to up to 60 %–80 % of the water-holding capacity and incubated for 1 week at 22 ∘C in the dark, this time being adequate for the recovery and stabilization of the growth of the bacterial communities after moisture adjustment (Meisner et al., 2013). After this time, the soil sample was distributed in eight polypropylene tubes (100 mL) (putting 12 g of soil, dry weight, in each), with seven of them being individually polluted with 1000 mg kg−1 of one of the seven heavy metals (As, Cd, Zn, Cu, Ni, Cr, or Pb), and the eighth tube acting as control (containing soil without metal). Then, the eight microcosms were distributed in 24 polypropylene tubes (50 mL) (eight microcosms × three replicates), placing 3.8 g (dry weight) in each tube, and they were incubated for 42 d in the dark. After 1 and 42 d of incubation, 1 g of each tube was used to measure the growth of bacterial communities, and after 42 d, 1.8 g samples were used to estimate the bacterial community tolerance to heavy metals. A schematic description of the experimental design is shown in Fig. S1 (Supplement).
The concentration of bioavailable heavy metals at days 0 and 42 is shown in Table S2 (Supplement). The concentrations of bioavailable heavy metals at day 0, determined in polluted soil samples extracted with CaCl2, were 617.4, 333.4, 834.8, 71.1, 429.0, 220.3, and 302.2 mg kg−1 (for As, Cd, Cr, Cu, Ni, Pb, and Zn respectively), whereas, for those extracted with EDTA, the concentrations were 917.4, 1016.9, 904.4, 982.1, 802.6, 1080.7, and 953.2 mg kg−1 for As, Cd, Cr, Cu, Ni, Pb, and Zn respectively (Table S2, Supplement). The bioavailable concentrations determined in polluted soil samples at day 42 of incubation were 95.7, 140.9, 191.3, 17.4, 252.2, 3.5, and 146.1 mg kg−1, when extracted with CaCl2, while the values were 426.1, 852.2, 252.2, 687.0, 800.0, 765.2, and 713.0 mg kg−1 when extracted with EDTA, corresponding to As, Cd, Cr, Cu, Ni, Pb, and Zn, respectively, in both extractants (Table S2, Supplement).
The toxicity exerted individually by the heavy metals on the bacterial communities was measured by estimating the bacterial growth on days 1 and 42 of incubation, following the protocol established by Bååth (1994) and Bååth et al. (2001). The bacterial community tolerance was determined according to Bååth (1992) and to Díaz-Raviña et al. (1994), with certain modifications indicated below and using the leucine incorporation method (Bååth, 1994; Bååth et al., 2001). Briefly, soil samples obtained from each microcosm were mixed with distilled water (rate 1:20 ()), using a multi-vortex shaker for 3 min at maximum intensity. Then, the soil / water mixture was centrifuged at 1000×g for 10 min to obtain the soil bacterial suspension (supernatant). Aliquots of 1.5 mL of the supernatant were transferred to 2 mL centrifugation tubes, in which 150 µL of pollutant solution (containing heavy metals or antibiotics) was subsequently added. As a result, a total of seven heavy metals (As, Cd, Zn, Cu, Ni, Cr, and Pb) and three antibiotics (TC, OTC, and CTC) were added to the bacterial suspensions (thus, a total of 10 pollutants were studied), and these plus a control (only water added) were used for each sample. The final concentrations of heavy metals added ranged between 10−2 and 10−6 mol L−1, while the range for antibiotics went from 400 to mg L−1. The bacterial community growth was estimated after a pre-incubation step of 24 h, where bacterial suspensions were added with the different concentrations of antibiotics before the leucine incorporation assay (Berg et al., 2010; Fernández-Calviño and Bååth, 2013). The [3H] leucine incorporation was then measured on each micro-centrifugation tube, as follows: a volume of 0.2 µL [3H] leucine (3.7 MBq mL−1 and 0.574 TBq mmol−1; Amersham) was added with non-labeled leucine to each tube, resulting in 275 nM leucine in the bacterial suspensions. After 3 h of incubation, the bacterial growth was terminated by adding 75 mL of 100 % trichloroacetic acid. Washing was performed as described by Bååth et al. (2001), and a subsequent measurement of radioactivity was carried out using a liquid scintillation counter (Tri-Carb 2810 TR, PerkinElmer, USA).
2.4 Data analyses
The tolerance of the bacterial community to the seven heavy metals (As, Cd, Zn, Cu, Ni, Cr, and Pb) and the three tetracycline antibiotics (TC, OTC, and CTC) was estimated as log IC50, the logarithm of the concentration that resulted in 50 % inhibition of bacterial community growth. The log IC50 was calculated using a logistic model, as follows:
where Y is the measured level of leucine incorporation, X is the logarithm of the contaminant substance (heavy metals (As, Cd, Zn, Cu, Ni, Cr, or Pb) or antibiotics (TC, OTC, or CTC)) added to the bacterial suspension, a is the log IC50, c is the bacterial growth rate in absence of the toxic substance, and b is a slope parameter indicating the inhibition rate. A higher value of log IC50 indicates a higher community tolerance, while a lower value indicates that the pollutants (heavy metals or antibiotics) are more toxic to the bacterial community.
3.1 Toxicity of heavy metals on soil bacteria
The toxicity of the heavy metals on soil bacterial communities was tested in the agricultural soil in the short and long term (1 and 42 d of incubation). The bacterial growth results obtained for the soil samples polluted with 1000 mg kg−1 of As, Cd, Zn, Cu, Ni, Cr, or Pb (separately) and for the unpolluted soil (0 mg kg−1) allowed us to establish a toxicity index for these heavy metals (Table 1). On day 1, the toxicity sequence was the following: Cr ≥ Cu ≥ Ni > Cd ≥ Zn > As ≥ Pb. After 42 d of incubation the sequence was similar, with changes mainly affecting Ni and Cd: Cr > Cu > Cd > Ni ≥ Zn ≥ As > Pb.
Table 1Toxicity index values ± error of soil samples containing 0 mg kg−1 (control soil) and 1000 mg kg−1 of As, Cd, Zn, Cu, Ni, Cr, or Pb (individually), after 1 and 42 d of incubation.
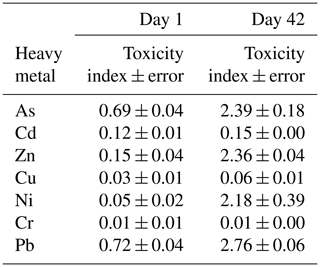
The data obtained in this study differ from those reported by other authors, which was expected, since the toxicity of heavy metals in soil microorganisms depends on the physicochemical characteristics of the soils (such us pH and carbon content), the concentrations of heavy metals added, and the incubation period (Hattori, 1992; Diaz-Raviña and Bååth, 1996; Nannipieri et al., 1997; Ahmad et al., 2005). Hattori (1992) evaluated the influence of Cd, Cr, Cu, Ni, Pb, and Zn on the microorganisms present in two soils after 4 weeks and obtained the toxicity sequence Cd > Cu > Ni > Cr > Zn for one of them (a Gleysol, with pH = 5.8 % and C = 0.5 %) and the sequence Cu > Cd > Ni > Zn > Cr for the other soil (an Andosol, with pH = 6.4 % and C = 3.2 %). Ahmad et al. (2005) measured the response of the microbial populations of one soil (pH = 7.6 % and C = 0.4 %) to Cu, Cd, Cr, Hg, Mn, Ni, Pb, and Zn at five incubation times, finding that the sensitivity of the bacterial populations depended on the functional group to which they belonged, the aerobic-heterotrophic bacteria being more sensitive to Ni, Pb, and Cu, while nitrogen-fixing bacteria were more sensitive to Cd and Pb. Bååth (1989) observed that the relative toxicity of metals decreased in the order Cd > Cu > Zn > Pb, although some differences were found among different investigations. Analyzing data obtained in different studies, it can be observed that, in general, the heavy metals that exert a higher effect on soil microorganisms are Cd and Cu. These results agree with those obtained using other organisms as endpoints, such as Daphnia magna, Vibrio fischeri, Lemna minor, and Eisenia fetida, which show that there is great variability in the toxicities caused by heavy metals but also that Cd and Cu are the most toxic (Neuhauser et al., 1985; Arambašić et al., 1995; Huynh and Bulich, 1995; Dirilgen, 2001; Hsieh et al., 2004; Teodorovic et al., 2009).
3.2 Soil bacterial communities' tolerance to heavy metals in the long term
Figure 1 shows the dose–response curves obtained for each heavy metal after 42 d of incubation, including data for each microcosm. These dose–response curves are sigmoidal, such as those reported by other authors (Díaz-Raviña et al., 1994; Cruz-Paredes et al., 2017; Song et al., 2017; Santás-Miguel et al., 2020a). The inhibition curves show the absence of bacterial growth inhibition at low heavy metals' concentrations, but inhibition takes place at high doses, and it increased with dose. As a general trend, it is observed that the inhibition curves obtained for all heavy metals tested shift to the right with respect to the control (unpolluted soil). This shift to the right in the dose–response curves suggests the existence of soil bacterial community tolerance to the high heavy metals' concentrations added to the soil used in this research (1000 mg kg−1).
In this study, the dose–response curves fitted well to the logistic model, with R2 ranging between 0.966 and 0.991 (mean R2=0.983) for the control sample (0 mg kg−1) and R2 between 0.861 and 0.987 (mean R2=0.950) for samples polluted with heavy metals (1000 mg kg−1 of each one, individually).
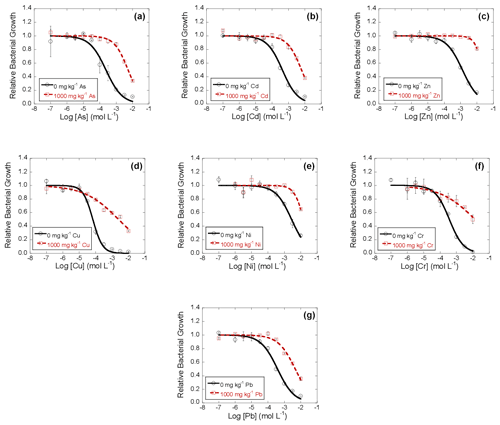
Figure 1Dose–response curves obtained after the addition of 10 different concentrations of the heavy metals As (a), Cd (b), Zn (c), Cu (d), Ni (e), Cr (f), and Pb (g) to bacterial suspensions extracted from soils polluted with 1000 mg kg−1 of each of the same heavy metals, separately, after 42 d of incubation. Black lines represent the dose–response curves corresponding to the unpolluted soil (control), and dashed red lines are dose–response curves corresponding to the soil polluted with 1000 mg kg−1 of heavy metal. Average values (n=3) with coefficients of variation always < 5 %.
Table 2 shows the log IC50 values obtained from each dose–response curve. The unpolluted soil showed the lowest log IC50 value for Cu (−4.17 ± 0.05) and the highest value for Ni (2.56 ± 0.08); i.e, the bacteria found in the bacterial suspension of unpolluted soils show high toxicity effects due to Cu and lower toxicity due to Ni. As regards the log IC50 values obtained for the whole set of heavy metals tested, the following toxicity sequence was observed for the control (unpolluted soil): Cu > As > Cr ≥ Pb ≥ Cd > Zn > Ni. This toxicity sequence is similar to that reported by Díaz-Raviña et al. (1994), who studied the bacterial community tolerance to Cu, Cd, Zn, Ni, and Pb using the thymidine incorporation technique and found that Cu was the most toxic, while Ni showed the lowest toxicity on bacterial communities. However, the order of the rest of heavy metals differed from that observed in the current study, with the sequence being Cu > Cd > Zn > Pb > Ni.
Regarding the samples polluted with 1000 mg kg−1 of each of the heavy metals separately, the lowest log IC50 score corresponded to Cu (−2.61 ± 0.12) and the highest to Zn (−1.63 ± 0.09) (Table 2); i.e., the bacterial communities present in these microcosms are more sensitive to Cu than to Zn. The toxicity sequence, as a function of the log IC50 values, was Cu > Pb ≥ As ≥ Cd ≥ Cr ≥ Ni ≥ Zn.
The log IC50 values obtained for each soil sample polluted with the highest heavy metal concentrations increased with respect to the control (unpolluted soil), indicating that the bacteria exposed to high levels of heavy metals developed tolerance to these pollutants. The highest increases in log IC50 (Δlog IC50, Table 2) corresponded to Cr and Cu (1.58 and 1.56 units, respectively), followed by As, Zn, Cd, and Pb (1.35, 1.26, 1.16 and 1.08 units, respectively), whereas the increase was of 0.74 units for Ni. It is noted that the presence of high amounts of heavy metals in the soil causes bacterial communities to increase their tolerance to these pollutants (Díaz-Raviña et al., 1994; Díaz-Raviña and Bååth, 1996, 2001; Fernández-Calviño and Bååth, 2013; Santás-Miguel et al., 2020a; Zhong et al., 2021), although the magnitude of these increases seems to depend on the toxicity or initial pressure exerted by the metal on the soil bacterial communities.
Figure S2 (Supplement) shows the relation between the increase in tolerance to heavy metals and the toxicity exerted by each metal on the bacterial growth in the short term (1 d) and in the long term (42 d). It can be observed that the higher the bacterial growth inhibition on days 1 and 42 is, the greater the increase in tolerance is generated by bacterial communities to heavy metals. This behavior was observed for all the heavy metals studied except Ni. In fact, eliminating Ni, the relation becomes significant at day 1 (r=0.789, P < 0.05) and at day 42 (r=0.730; P < 0.05). The toxicity on soil bacterial communities due to heavy metals is related to their bioavailability in the soil environment. The time-course evolution of heavy metals' bioavailability is shown in Table S2 (Supplement), indicating that in general they are highly bioavailable in the short term (with Ni showing the lowest bioavailability), while in the long term (concentrations in EDTA) their bioavailability decreases in a percentage of between 20 % and 70 % (although being less in the case of Ni, which remains constant over time).
The results suggest that the increase in soil bacterial community tolerance after the addition of heavy metals can be attributed to the immediate effect caused by the decay of sensitive species and to a posterior effect caused by different competitive capacities and ulterior adaptation in the bacteria remaining. This effect of heavy metals in soil has been observed previously by Diaz-Raviña and Bååth (1996) for Zn, Cd, Ni, Cu, and Pb and by Fernández-Calviño and Bååth (2016) for Cu.
3.3 Soil bacterial communities' tolerance to tetracycline antibiotics in the long term
Figures 2, 3, and 4 show dose–response curves obtained at 42 d of incubation for each heavy metal added to the soil at 1000 mg kg−1, after the exposure of bacterial suspension from each microcosm to concentrations of tetracyclines antibiotics (TC, OTC, and CTC) ranging from 400 to mg L−1. The inhibition curves obtained for these tetracycline antibiotics are sigmoidal. As a general trend, the dose–response curves are shifted to the right with respect to the control, and in some cases the tetracycline antibiotics did not show an inhibitory effect on bacterial communities.
These dose–response curves fitted well to the logistic model, with R2 ranging between 0.925 and 0.982 (mean R2=0.956) for TC samples, between 0.771 and 0.997 (mean R2=0.952) for OTC samples, and from 0.959 to 0.992 (mean R2=0.976) for CTC samples.
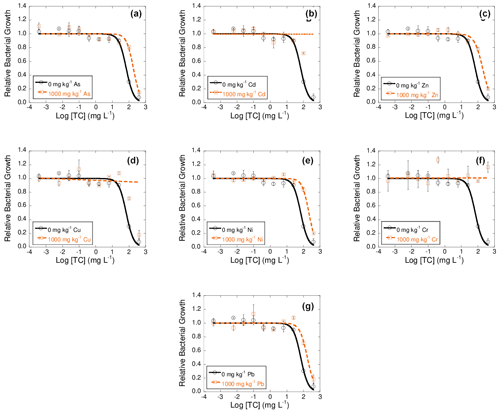
Figure 2Dose–response curves obtained after the addition of 10 different concentrations of tetracycline (TC) to bacterial suspension extracted from soil samples polluted with 1000 mg kg−1 of As (a), Cd (b), Zn (c), Cu (d), Ni (e), Cr (f), or Pb (g), each of them added separately, after 42 d of incubation. Black lines represent the dose–response curves obtained for the unpolluted soil (control), and dashed orange lines are dose–response curves for soils polluted with 1000 mg kg−1 of one of the heavy metals. Average values (n=3) with coefficients of variation always < 5 %.
Table 3 shows the log IC50 values obtained from each dose–response curve, being 1.83 ± 0.06 for the control sample after exposure of the bacterial suspension to different concentrations of TC. In general, the log IC50 values obtained for each microcosm polluted with 1000 mg kg−1 of each heavy metal and subsequently exposed to TC increased with respect to the control. The magnitude of these increases varies as a function of the heavy metal, being 0.40, 0.45, and 0.43 units for Pb, Zn, and As and 0.50 units to Ni. However, the bacterial communities of soils contaminated with Cd, Cu, and Cr do not show inhibition in their growth when exposed to TC, and, therefore, their log IC50 cannot be determined due to the high community tolerance to TC. Consequently, heavy metals that do not show inhibition have been assigned a log IC50 value of > 2.60, since it is the maximum concentration tested in the current study.
Table 2Estimated values of log IC50 ± error and R2 from fits to a logistic model of soil samples containing 0 mg kg−1 (control soil) and 1000 mg kg−1 of As, Cd, Zn, Cu, Ni, Cr, or Pb (individually). The increases in tolerance (Δlog IC50= log IC50 polluted − log IC 50 control) were calculated for each heavy metal.
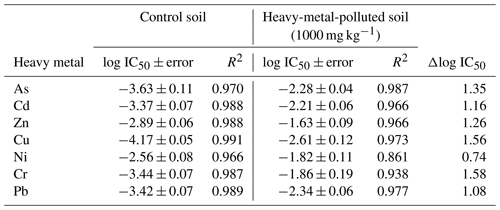
Table 3Estimated values of log IC50 ± error and R2 from fits to a logistic model of soil samples polluted with 1000 mg kg−1 of As, Cd, Zn, Cu, Ni, Cr, and Pb. The increases in tolerance (Δlog IC50= log IC50 polluted − log IC50 control) were calculated for tetracycline (TC), oxytetracycline (OTC), and chlortetracycline (CTC).
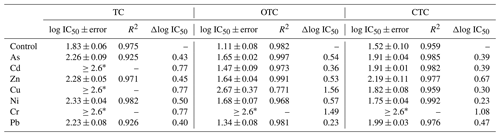
* The value of 2.6 is the maximum value tested.
The log IC50 value obtained for the control soil in presence of OTC was 1.11 ± 0.08. Regarding the log IC50 values obtained for the samples polluted with heavy metals, it can be observed that, after the exposure of the bacterial suspension to OTC, there was an increase in these values with respect to the control, going from 0.23 to 0.57 for Pb, Cd, Zn, As, and Ni and being 1.56 for Cu. Regarding Cr, the exposure of the soil bacterial suspension to OTC did not cause inhibition of its growth, and, therefore, as in the case of Cd, Cu, and Cr for TC, the value of > 2.60 (maximum concentration tested) was assigned.
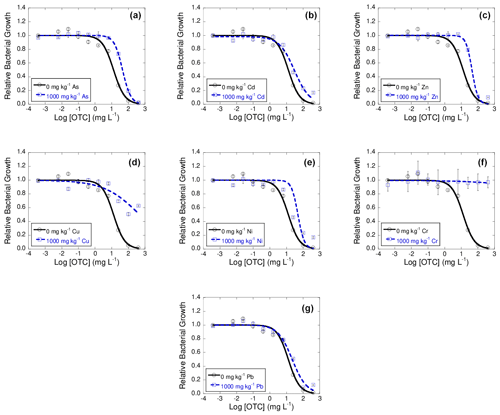
Figure 3Dose–response curves obtained after the addition of 10 different concentration of oxytetracycline (OTC) to bacterial suspension extracted from soil samples polluted with 1000 mg kg−1 of As (a), Cd (b), Zn (c), Cu (d), Ni (e), Cr (f), or Pb (g), each of them added separately, after 42 d of incubation. Black lines represent the dose–response curves obtained for the unpolluted soil (control), and dashed blue lines are dose–response curves for soils polluted with 1000 mg kg−1 of one of the heavy metals. Average values (n=3) with coefficients of variation always < 5 %.
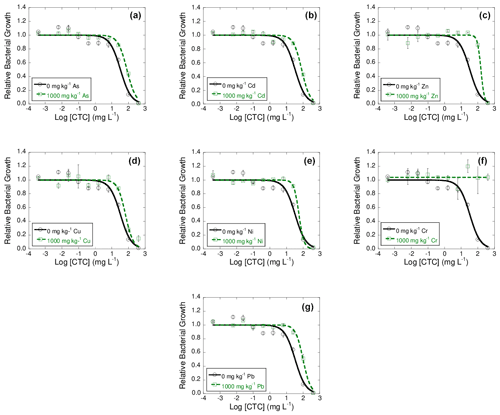
Figure 4Dose–response curves obtained after the addition of 10 different concentration of chlortetracycline (CTC) to bacterial suspension extracted from soil samples polluted with 1000 mg kg−1 of As (a), Cd (b), Zn (c), Cu (d), Ni (e), Cr (f), or Pb (g), each of them added separately, after 42 d of incubation. Black lines represent the dose–response curves obtained for the unpolluted soil (control), and dashed green lines are dose–response curves for soils polluted with 1000 mg kg−1 of one of the heavy metals. Average values (n=3) with coefficients of variation always < 5 %.
Finally, the log IC50 value obtained for the control soil added with CTC was 1.52 ± 0.10. After exposure of the bacterial suspensions to CTC, the log IC50 values obtained for the samples polluted with heavy metals showed an increase with respect to the control, going from 1.75 to 2.19 for all the heavy metals except for Cr, which did not show inhibition of bacterial growth when exposed to different concentrations of CTC. Therefore, it can be indicated that bacterial communities exposed to high concentrations of heavy metals show great co-tolerance to CTC. In general, the increase in tolerance obtained for TC, OTC, and CTC cannot be related to the toxicity of heavy metals in the soil, at 1 and 42 d of incubation (data not shown).
Co-tolerance to TC generated by soil bacterial communities exposed to heavy metals has been previously demonstrated (Berg et al., 2010; Fernández-Calviño and Bååth, 2013; Song et al., 2017). Song et al. (2017) observed the existence of co-tolerance to TC in a microcosm exposed to concentrations of Cu ≥ 333 and Zn ≥ 500 mg kg−1. Fernández-Calviño and Bååth (2013) found co-tolerance to TC of soil bacterial communities in contaminated soils with concentrations of Cu ≥ 500 mg kg−1. The results shown in these studies agree with what was observed in the present work, indicating that soil bacterial communities exposed to high concentrations of heavy metals (1000 mg kg−1) generate co-tolerance to tetracycline. However, Zhong et al. (2021) did not observe co-tolerance to TC in soils contaminated with Cu, Zn, and Pb, while they found co-tolerance of soil bacterial communities to vancomycin.
This co-tolerance of soil bacterial communities to TC at high concentrations of heavy metals is also observed for OTC and CTC. The results obtained in this study agree with the data reported in studies previously carried out by Santás-Miguel et al. (2020a), where co-tolerance of soil bacterial communities to tetracycline antibiotics (TC, OTC, and CTC) was observed in soils with concentrations of Cu ≥ 1000 mg kg−1 after 42 d of incubation.
It is relevant that co-tolerance studies, in general, focus on a reduced number of heavy metals (generally Cu) and tetracycline antibiotics, while for the first time the present work shows the effect of seven different heavy metals as regards the co-tolerance to the three most used tetracycline antibiotics (TC, OTC, and CTC).
It is also remarkable that the role of heavy metals as a co-selection factor to generate antibiotic resistance genes has been studied for a wide variety of heavy metals and antibiotics (Berg et al., 2010; Dickinson et al., 2019; Cao et al., 2020; Mazhar et al., 2021), and, although in the present study antibiotic resistance genes (ARGs) have not been studied directly, the resistance of soil bacterial communities to heavy metals and tetracycline antibiotics (TC, OTC, and CTC) could be detected using the leucine incorporation method. The heavy metal levels of the tested microcosms (1000 mg kg−1) are really very high, but they should not be considered unrealistic, since heavy metals are not easily biodegradable, and therefore their concentrations can increase over time (Li et al., 2015), reaching very high levels in the soil (Smolders et al., 2004; Spiteri et al., 2005; Zhuang et al., 2009; Komárek et al., 2010). Therefore, soils contaminated with high concentrations of heavy metals could become hotspots of ARGs, which would pose a potential danger to public health, since they could be transmitted to humans (Forsberg et al., 2012).
In the current study it was evidenced that heavy metals present in soils show wide variability as regards the toxicity they exert on bacterial communities, with Cd and Cu being the most toxic. Specifically, soil samples contaminated with a high concentration (1000 mg kg−1) of each of seven different heavy metals (As, Cd, Zn, Cu, Ni, Cr, and Pb), added separately, showed tolerance of the soil bacterial communities to the heavy metals themselves, compared to the control soil. Besides, it was shown that the higher the effect of heavy metals in the short and long term, the greater the increase in tolerance to metals generated in the soil bacterial communities (except for Ni). In addition, soil samples contaminated with high concentrations of heavy metals also showed co-tolerance of soil bacterial communities to tetracycline, oxytetracycline, and chlortetracycline for all the metals tested, compared to the control soil. However, in general, there was no correlation between the initial damage exerted by heavy metals in the short and long term and the co-tolerance shown by soil bacterial communities to tetracycline antibiotics. Future studies could give further details about the potential interactions of different pollutants present in agricultural soils, which can be considered relevant as regards environmental preservation and public health.
All raw data can be provided by the corresponding authors upon request.
The supplement related to this article is available online at: https://doi.org/10.5194/soil-8-437-2022-supplement.
MAE, EAR, AND, MDR and DFC conceptualized the study, methodology and data curation; VSM performed the investigation; VSM and DFC used the software; VSM, MAE and DFC wrote the original draft; VSM, MAE, EAR, AND, MDR and DFC visualized the original draft; MAE and AND supervised the final paper; MAE, EAR, AND, MDR and DFC validated the data; and MAE, AND and DFC wrote, reviewed and edited the final paper.
The contact author has declared that neither they nor their co-authors have any competing interests.
Publisher’s note: Copernicus Publications remains neutral with regard to jurisdictional claims in published maps and institutional affiliations.
This study has been funded by the Spanish Ministry of Economy and Competitiveness through the projects CGL2015-67333-C2-1-R and CGL2015-67333-C2-2-R (FEDER Funds). David Fernández-Calviño holds a Ramón y Cajal contract (RYC-2016-20411), financed by the Spanish Ministry of Economy, Industry and Competitiveness. Vanesa Santás-Miguel holds a pre-doctoral fellowship (ED481A-2020/089) financed by Xunta de Galicia.
This study has been funded by the Spanish Ministry of Economy and Competitiveness through the projects CGL2015-67333-C2-1-R and CGL2015-67333-C2-2-R (FEDER Funds). David Fernández-Calviño holds a Ramón y Cajal contract (RYC-2016-20411), financed by the Spanish Ministry of Economy, Industry and Competitiveness. Vanesa Santás-Miguel holds a pre-doctoral fellowship (ED481A-2020/089) financed by Xunta de Galicia.
This paper was edited by Rafael Clemente and reviewed by two anonymous referees.
Abdu, N., Abdullahi, A. A., and Abdulkadir, A.: Heavy metals and soil microbes, Environ. Chem. Lett., 15, 65–84, https://doi.org/10.1007/s10311-016-0587-x, 2017.
Ahmad, I., Hayat, S., Ahmad, A., and Inam, A.: Effect of heavy metal on survival of certain groups of indigenous soil microbial population, J. Appl. Sci. Environ. Mgt., 9, 115–121, 2005.
Arambašić, M. B., Bjelić, S., and Subakov, G.: Acute toxicity of heavy metals (copper, lead, zinc), phenol and sodium on Allium cepa L., Lepidium sativum L. and Daphnia magna St.: Comparative investigations and the practical applications, Water Res., 29, 497–503, https://doi.org/10.1016/0043-1354(94)00178-A, 1995.
Bååth, E.: Effects of heavy metals in soil on microbial processes and populations (a review), Water Air Soil Pollut., 47, 335–379, https://doi.org/10.1007/BF00279331, 1989.
Bååth, E.: Measurement of heavy metal tolerance of soil bacteria using thymidine incorporation into bacteria extracted after homogenization-centrifugation, Soil Boil. Biochem., 24, 1167–1172, https://doi.org/10.1016/0038-0717(92)90067-8, 1992.
Bååth, E.: Thymidine and leucine incorporation in soil bacteria with different cell size, Microb. Ecol., 27, 267–278, https://doi.org/10.1007/BF00182410, 1994.
Bååth, E., Petterson, M., and Söderberg, K. H.: Adaptation of a rapid and economical microcentrifugation method to measure thymidine and leucine incorporation by soil bacteria, Soil Biol. Biochem., 33, 1571–1574, https://doi.org/10.1016/S0038-0717(01)00073-6, 2001.
Berg, J., Thorsen, M. K., Holm, P. E., Jensen, J., Nybroe, O., and Brandt, K. K.: Cu exposure under field conditions coselects for antibiotic resistance as determined by a novel cultivation-independent bacterial community tolerance assay, Environ. Sci. Technol., 44, 8724–8728, https://doi.org/10.1021/es101798r, 2010.
Blanck, H.: A critical review of procedures and approaches used for assessing pollution-induced community tolerance (PICT) in biotic communities, Hum. Ecol. Risk Assess., 8, 1003–1034, https://doi.org/10.1080/1080-700291905792, 2002.
Boxall, A. B., Kolpin, D. W., Halling-Sørensen, B., and Tolls, J.: Peer reviewed: are veterinary medicines causing environmental risks?, Environ. Sci. Technol., 37, 286A–294A, 2003.
Caban, J. R., Kuppusamy, S., Kim, J. H., Yoon, Y. E., Kim, S. Y., and Lee, Y. B.: Green manure amendment enhances microbial activity and diversity in antibiotic-contaminated soil, Appl. Soil Ecol., 129, 72–76, https://doi.org/10.1016/j.apsoil.2018.04.013, 2018.
Cao, J., Yang, G., Mai, Q., Zhuang, Z., and Zhuang, L.: Co-selection of antibiotic-resistant bacteria in a paddy soil exposed to as (III) contamination with an emphasis on potential pathogens, Sci. Total Environ., 725, 138367, https://doi.org/10.1016/j.scitotenv.2020.138367, 2020.
Chien, C., Yumei, K. U. O., Changchieh, C. H. E. N., Chunwei, H. U. N. G., Chihwei, Y. E. H., and Weijen, Y. E. H.: Microbial diversity of soil bacteria in agricultural field contaminated with heavy metals, J. Environ. Sci., 20, 359–363, https://doi.org/10.1016/S1001-0742(08)60056-X, 2008.
Conde-Cid, M., Álvarez-Esmorís, C., Paradelo-Núñez, R., Nóvoa-Muñoz, J. C., Arias-Estévez, M., Álvarez-Rodríguez, E., Fernández-Sanjurjo, M. J., and Núñez-Delgado, A.: Occurrence of tetracyclines and sulfonamides in manures, agricultural soils and crops from different areas in Galicia (NW Spain), J. Clean. Prod., 197, 491–500, https://doi.org/10.1016/j.jclepro.2018.06.217, 2018.
Cruz-Paredes, C., Wallander, H., Kjøller, R., and Rousk, J.: Using community trait-distributions to assign microbial responses to pH changes and Cd in forest soils treated with wood ash, Soil Biol. Biochem., 112, 153–164, https://doi.org/10.1016/j.soilbio.2017.05.004, 2017.
Díaz-Raviña, M., Bååth, E., and Frostegård, Å.: Multiple heavy metal tolerance of soil bacterial communities and its measurement by a thymidine incorporation technique, Appl. Environ. Microbiol., 60, 2238–2247, https://doi.org/10.1128/aem.60.7.2238-2247.1994, 1994.
Díaz-Raviña, M. and Bååth, E.: Development of metal tolerance in soil bacterial communities exposed to experimentally increased metal levels, Appl. Environ. Microbiol., 62, 2970–2977, https://doi.org/10.1128/aem.62.8.2970-2977.1996, 1996.
Díaz-Raviña, M. and Bååth, E.: Response of soil bacterial communities pre-exposed to different metals and reinoculated in an unpolluted soil, Soil Biol. Biochem., 33, 241–248, https://doi.org/10.1016/S0038-0717(00)00136-X, 2001.
Dickinson, A. W., Power, A., Hansen, M. G., Brandt, K. K., Piliposian, G., Appleby, P., O'Neill, P. A., Jones, R. T., Sierocinski, P., Koskella, B., and Vos, M.: Heavy metal pollution and co-selection for antibiotic resistance: A microbial palaeontology approach, Environ. Int., 132, 105117, https://doi.org/10.1016/j.envint.2019.105117, 2019.
Dirilgen, N.: Accumulation of heavy metals in freshwater organisms: Assessment of toxic interactions, Turk. J. Chem., 25, 173–179, 2001.
European Medicines Agency: European surveillance of veterinary antimicrobial consumption, in: Sales of Veterinary Antimicrobial Agents in 29 European Countries in 2014, (EMA/61769/2016), https://doi.org/10.2809/676974, 2016.
Fernández-Calviño, D. and Bååth, E.: Co-selection for antibiotic tolerance in Cu-polluted soil is detected at higher Cu-concentrations than increased Cu-tolerance, Soil Biol. Biochem., 57, 953–956, https://doi.org/10.1016/j.soilbio.2012.08.017, 2013.
Fernández-Calviño, D. and Bååth, E.: Interaction between pH and Cu toxicity on fungal and bacterial performance in soil, Soil Biol. Biochem., 96, 20–29, https://doi.org/10.1016/j.soilbio.2016.01.010, 2016.
Forsberg, K. J., Reyes, A., Wang, B., Selleck, E. M., Sommer, M. O. A., and Dantas, G.: The shared antibiotic resistome of soil bacteria and human pathogens, Science, 337, 1107–1111, https://doi.org/10.1126/science.1220761, 2012.
Giller, K. E., Witter, E., and McGrath, S. P.: Heavy metals and soil microbes, Soil Biol. Biochem., 41, 2031–2037, https://doi.org/10.1016/j.soilbio.2009.04.026, 2009.
Hamscher, G., Sczesny, S., Höper, H., and Nau, H.: Determination of persistent tetracycline residues in soil fertilized with liquid manure by high-performance liquid chromatography with electrospray ionization tandem mass spectrometry, Anal. Chem., 74, 1509–1518, https://doi.org/10.1021/ac015588m, 2002.
Hattori, H.: Influence of heavy metals on soil microbial activities, Soil Sci. Plant Nutr., 38, 93–100, https://doi.org/10.1080/00380768.1992.10416956, 1992.
Hölzel, C. S., Müller, C., Harms, K. S., Mikolajewski, S., Schäfer, S., Schwaiger, K., and Bauer, J.: Heavy metals in liquid pig manure in light of bacterial antimicrobial resistance, Environ. Res., 113, 21–27, https://doi.org/10.1016/j.envres.2012.01.002, 2012.
Hsieh, C. Y., Tsai, M. H., Ryan, D. K., and Pancorbo, O. C.: Toxicity of the 13 priority pollutant metals to Vibrio fisheri in the Microtox® chronic toxicity test, Sci. Total Environ., 320, 37–50, https://doi.org/10.1016/S0048-9697(03)00451-0, 2004.
Huang, S. S., Liao, Q. L., Hua, M., Wu, X. M., Bi, K. S., Yan, C. Y., Chen B., and Zhang, X. Y.: Survey of heavy metal pollution and assessment of agricultural soil in Yangzhong district, Jiangsu Province, China, Chemosphere, 67, 2148–2155, https://doi.org/10.1016/j.chemosphere.2006.12.043, 2007.
Huynh, H. and Bulich, A.: Measuring chronic toxicity using luminescent bacteria (No. CONF-9410273-), Society of Environmental Toxicology and Chemistry, Pensacola, FL (United States), 1994.
Ji, X., Shen, Q., Liu, F., Ma, J., Xu, G., Wang, Y., and Wu, M.: Antibiotic resistance gene abundances associated with antibiotics and heavy metals in animal manures and agricultural soils adjacent to feedlots in Shanghai, China, J. Hazard. Mater., 235, 178–185, https://doi.org/10.1016/j.jhazmat.2012.07.040, 2012.
Kabata-Pendias, A.: Trace elements in soils and plants, CRC press, https://doi.org/10.1201/9781420039900, 2000.
Komárek, M., Čadková, E., Chrastný, V., Bordas, F., and Bollinger, J. C.: Contamination of vineyard soils with fungicides: a review of environmental and toxicological aspects, Environ. Int., 36, 138–151, https://doi.org/10.1016/j.envint.2009.10.005, 2010.
Kornegay, E. T., Hedges, J. D., Martens, D. C., and Kramer, C. Y.: Effect on soil and plant mineral levels following application of manures of different copper contents, Plant Soil., 45, 151–162, https://doi.org/10.1007/BF00011137, 1976.
Lakanen, E. and Erviö, R: A comparison of eight extractants for the determination of plant available micronutrients in soils, Acta Agric. Fenn., 123, 223–232, 1971.
Li, J., Yu, H., and Luan, Y.: Meta-analysis of the copper, zinc, and cadmium absorption capacities of aquatic plants in heavy metal-polluted water, Int. J. Environ. Res. Public Health., 12, 14958–14973, https://doi.org/10.3390/ijerph121214959, 2015.
Liu, W. R., Zeng, D., She, L., Su, W. X., He, D. C., Wu, G. Y., Ma, X. R., Jiang, S., Jiang C.H., and Ying, G. G.: Comparisons of pollution characteristics, emission situations, and mass loads for heavy metals in the manures of different livestock and poultry in China, Sci. Total Environ., 734, 139023, https://doi.org/10.1016/j.scitotenv.2020.139023, 2020.
Macías, F. and Calvo, R.: Niveles genéricos de referencia de metales pesados y otroselementos traza en suelos de Galicia (Reference values for heavy metals and other traceelements in soils in Galicia), in: Consellería de Medio Ambiente e Desenvolvemento Sostible, Xunta de Galicia, Santiago de Compostela, p. 229, ISBN 978-84-453-4664-8, 2008.
Mazhar, S. H., Li, X., Rashid, A., Su, J., Xu, J., Brejnrod, A. D., Sue J.-Q., Wu Y., Zhu Y.-G., Zhou S. G., and Rensing, C.: Co-selection of antibiotic resistance genes, and mobile genetic elements in the presence of heavy metals in poultry farm environments, Sci. Total Environ., 755, 142702, https://doi.org/10.1016/j.scitotenv.2020.142702, 2021.
Meisner, A., Bååth, E., and Rousk, J.: Microbial growth responses upon rewetting soil dried for four days or one year, Soil Biol. Biochem., 66, 188–192, https://doi.org/10.1016/j.soilbio.2013.07.014, 2013.
Mirlean, N., Roisenberg, A., and Chies, J. O.: Metal contamination of vineyard soils in wet subtropics (southern Brazil), Environ. Pollut., 149, 10–17, https://doi.org/10.1016/j.envpol.2006.12.024, 2007.
Nannipieri, P., Badalucco, L., Landi, L., and Pietramellara, G.: Measurement in assessing the risk of chemicals to the soil ecosystem, in: Ecotoxicology: Responses, Biomarkers and Risk Assessment, edited by: Zelikoff, J. T., Fair Haven, NJ, SOS Publications, 507–534, 1997.
Neuhauser, E. F., Loehr, R. C., Milligan, D. L., and Malecki, M. R.: Toxicity of metals to the earthworm Eisenia fetida, Biol. Fertil. Soils., 1, 149–152, https://doi.org/10.1007/BF00301782, 1985.
Nicholson, F. A., Smith, S. R., Alloway, B. J., Carlton-Smith, C., and Chambers, B. J.: An inventory of heavy metals inputs to agricultural soils in England and Wales, Sci. Total Environ., 311, 205–219, https://doi.org/10.1016/S0048-9697(03)00139-6, 2003.
Novozamsky, I., Lexmond, T. H. M., and Houba, V. J. G.: A single extraction procedure of soil for evaluation of uptake of some heavy metals by plants, Int. J. Environ. Anal. Chem., 51, 47–58, https://doi.org/10.1080/03067319308027610, 1993.
Pan, X., Qiang, Z., Ben, W., and Chen, M.: Residual veterinary antibiotics in swine manure from concentrated animal feeding operations in Shandong Province, China, Chemosphere, 84, 695–700, https://doi.org/10.1016/j.chemosphere.2011.03.022, 2011.
Sager, M.: Trace and nutrient elements in manure, dung and compost samples in Austria, Soil Biol. Biochem., 39, 1383–1390, https://doi.org/10.1016/j.soilbio.2006.12.015, 2007.
Santás-Miguel, V., Arias-Estévez, M., Díaz-Raviña, M., Fernández-Sanjurjo, M. J., Álvarez-Rodríguez, E., Núñez-Delgado, A., and Fernández-Calviño, D.: Bacterial Community Tolerance to Tetracycline Antibiotics in Cu Polluted Soils, Agronomy, 10, 1220, https://doi.org/10.3390/agronomy10091220, 2020a.
Santás-Miguel, V., Arias-Estévez, M., Díaz-Raviña, M., Fernández-Sanjurjo, M. J., Álvarez-Rodríguez, E., Núñez-Delgado, A., and Fernández-Calviño, D.: Interactions between soil properties and tetracycline toxicity affecting to bacterial community growth in agricultural soil, Appl. Soil Ecol., 147, 103437, https://doi.org/10.1016/j.apsoil.2019.103437, 2020b.
Santás-Miguel, V., Arias-Estévez, M., Díaz-Raviña, M., Fernández-Sanjurjo, M. J., Álvarez-Rodríguez, E., Núñez-Delgado, A., and Fernández-Calviño, D.: Effect of oxytetracycline and chlortetracycline on bacterial community growth in agricultural soils, Agronomy, 10, 1011, https://doi.org/10.3390/agronomy10071011, 2020c.
Sarma, B., Acharya, C., and Joshi, S. R.: Pseudomonads: a versatile bacterial group exhibiting dual resistance to metals and antibiotics, Afr. J. Microbiol. Res., 4, 2828–2835, 2010.
Sarmah, A. K., Meyer, M. T., and Boxall, A. B.: A global perspective on the use, sales, exposure pathways, occurrence, fate and effects of veterinary antibiotics (VAs) in the environment, Chemosphere, 65, 725–759, 2006.
Smolders, E., Buekers, J., Oliver, I., and McLaughlin, M. J.: Soil properties affecting toxicity of zinc to soil microbial properties in laboratory-spiked and field-contaminated soils, Environ. Toxicol. Chem., 23, 2633–2640, https://doi.org/10.1897/04-27, 2004.
Song, J., Rensing, C., Holm, P. E., Virta, M., and Brandt, K. K.: Comparison of metals and tetracycline as selective agents for development of tetracycline resistant bacterial communities in agricultural soil, Environ. Sci. Technol., 51, 3040–3047, https://doi.org/10.1021/acs.est.6b05342, 2017.
Spiteri, C., Kalinski, V., Rösler, W., Hoffmann, V., and Appel, E.: Magnetic screening of a pollution hotspot in the Lausitz area, Eastern Germany: correlation analysis between magnetic proxies and heavy metal contamination in soils, Environ. Geol., 49, 1–9, https://doi.org/10.1007/s00254-005-1271-9, 2005.
Teodorovic, I., Planojevic, I., Knezevic, P., Radak, S., and Nemet, I.: Sensitivity of bacterial vs. acute Daphnia magna toxicity tests to metals, Cent. Eur. J. Biol., 4, 482–492, https://doi.org/10.2478/s11535-009-0048-7, 2009.
Thiele-Bruhn, S. and Beck, I. C.: Effects of sulfonamide and tetracycline antibiotics on soil microbial activity and microbial biomass, Chemosphere, 59, 457–465, https://doi.org/10.1016/j.chemosphere.2005.01.023, 2005.
Van Boeckel, T. P., Brower, C., Gilbert, M., Grenfell, B. T., Levin, S. A., Robinson, T. P., Teillant, A., and Laxminarayan, R.: Global trends in antimicrobial use in food animals, P. Natl. Acad. Sci. USA, 112, 5649–5654, https://doi.org/10.1073/pnas.1503141112, 2015.
Wang, H., Dong, Y., Yang, Y., Toor, G. S., and Zhang, X.: Changes in heavy metal contents in animal feeds and manures in an intensive animal production region of China, J. Environ. Sci., 25, 2435–2442, https://doi.org/10.1016/S1001-0742(13)60473-8, 2013.
Widyasari-Mehta, A., Hartung, S., and Kreuzig, R.: From the application of antibiotics to antibiotic residues in liquid manures and digestates: A screening study in one European center of conventional pig husbandry, J. Environ. Manag., 177, 129–137, https://doi.org/10.1016/j.jenvman.2016.04.012, 2016.
Wu, L., Pan, X., Chen, L., Huang, Y., Teng, Y., Luo, Y., and Christie, P.: Occurrence and distribution of heavy metals and tetracyclines in agricultural soils after typical land use change in east China, Environ. Sci. Pollut. Res., 20, 8342–8354, https://doi.org/10.1007/s11356-013-1532-1, 2013.
Zhang, F., Li, Y., Yang, M., and Li, W.: Content of heavy metals in animal feeds and manures from farms of different scales in northeast China, Int. J. Environ. Res. Public Health, 9, 2658–2668, https://doi.org/10.3390/ijerph9082658, 2012.
Zhong, Q., Cruz-Paredes, C., Zhang, S., and Rousk, J.: Can heavy metal pollution induce bacterial resistance to heavy metals and antibiotics in soils from an ancient land-mine?, J. Hazard. Mater., 411, 124962, https://doi.org/10.1016/j.jhazmat.2020.124962, 2021.
Zhuang, P., McBride, M. B., Xia, H., Li, N., and Li, Z.: Health risk from heavy metals via consumption of food crops in the vicinity of Dabaoshan mine, South China, Sci. Total Environ., 407, 1551–1561, https://doi.org/10.1016/j.scitotenv.2008.10.061, 2009.