the Creative Commons Attribution 4.0 License.
the Creative Commons Attribution 4.0 License.
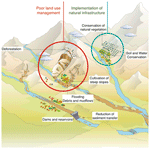
The effect of natural infrastructure on water erosion mitigation in the Andes
Veerle Vanacker
Armando Molina
Miluska A. Rosas
Vivien Bonnesoeur
Francisco Román-Dañobeytia
Boris F. Ochoa-Tocachi
Wouter Buytaert
To expand the knowledge base on natural infrastructure for erosion mitigation in the Andes, it is necessary to move beyond case by case empirical studies to comprehensive assessments. This study reviews the state of evidence on the effectiveness of interventions to mitigate soil erosion by water and is based on Andean case studies published in gray and peer-reviewed literature. Based on a systematic review of 118 case studies from the Andes, this study addressed the following research questions. (1) Which erosion indicators allow us to assess the effectiveness of natural infrastructure? (2) What is the overall impact of working with natural infrastructure on on-site and off-site erosion mitigation? (3) Which locations and types of studies are needed to fill critical gaps in knowledge and research?
Three major categories of natural infrastructure were considered: restoration and protection of natural vegetation, such as forest or native grasslands, forestation with native or exotic species and implementation of soil and water conservation measures for erosion mitigation. From the suite of physical, chemical and biological indicators commonly used in soil erosion research, two indicators were particularly relevant: soil organic carbon of topsoil and soil loss rates at plot scale. The protection and conservation of natural vegetation has the strongest effect on soil quality, with 3.01±0.893 times higher soil organic carbon content in the topsoil compared to control sites. Soil quality improvements are significant but lower for forestation and soil and water conservation measures. Soil and water conservation measures reduce soil erosion to 62.1 % ± 9.2 %, even though erosion mitigation is highest when natural vegetation is maintained. Further research is needed to evaluate whether the reported effectiveness holds during extreme events related to, for example, El Niño–Southern Oscillation.
- Article
(1700 KB) - Full-text XML
-
Supplement
(239 KB) - BibTeX
- EndNote
The Andes Mountains stretch over about 8900 km and cross tropical, subtropical, temperate and arid latitudes. Very few, if any, of the diverse physiographic, climatic and biogeographic regions in the Andes have been preserved from human impact. The area has been inhabited by humans for more than 15 000 years (Jantz and Behling, 2012; Keating, 2007). By the mid-20th century, all Andean nations with the exception of Argentina experienced an exponential population growth that caused substantial migration both within and between national borders (Little, 1981). More than 85 million people lived in the Andean region by 2020, with the northern Andes being one of the most densely populated mountain regions in the world (Devenish and Gianella, 2012). The demographic growth and a stagnating agricultural productivity per hectare led to an expansion of the total agricultural land area, either upward to steep hillsides at high elevations covered by native grassland-wetlands ecosystems (Velez et al., 2021), or downward to lands east and west of the Andes covered by tropical and subtropical forests (Wunder, 1996). Land abandonment is widespread where smallholders faced unfavorable economic conditions due to restricted land bases, limited availability of farm credit and low productivity in fragile agro-ecological environments (Zimmerer, 1993).
The strong latitudinal gradients in climate and vegetation are reflected in the pronounced north-south gradient in natural erosion processes and rates (Latrubesse and Restrepo, 2014; Montgomery et al., 2001). Natural erosion rates are lowest (<25 t km−2 yr−1) in the hyper-arid and arid regions but show high temporal variability as a result of extreme events, in particular during warm El Niño–Southern Oscillation (ENSO) conditions or earthquakes (Carretier et al., 2018; Morera et al., 2017). Erosion rates are usually higher (with rates of >250 t km−2 yr−1) in the humid regions where the catchment areas are deeply dissected by bedrock river channels and where landslides are common (Blodgett and Isacks, 2007; Vanacker et al., 2020). Land use and management have significantly altered the magnitude and frequency of erosion events (Restrepo et al., 2015; Tolorza et al., 2014; Vanacker et al., 2007a). Deforestation and agricultural practices (such as soil tillage and cattle grazing) increase erosion rates (Molina et al., 2007; Podwojewski et al., 2002), river sediment loads (Restrepo et al., 2015) and landslide occurrences (Guns and Vanacker, 2014). Changes in smallholder livelihoods leading to the abandonment of agricultural land have a nonlinear impact on soil erosion rates as they are often associated with an initial increase in soil erosion, followed by a steady decrease in erosion rates in the long term (Harden, 2001).
To tackle soil erosion and mitigate the on-site and off-site effects, governmental and nongovernmental organizations in the Andean countries launched rural development and soil conservation programs in the 1970s and 1980s: for example, the programs by PRONAREG-MAG-ORSTOM and USAID in Ecuador (De Noni et al., 2001), IIDE and USAID in Bolivia (Zimmerer, 1993) and PRONAMACHCS in Peru (Torero Zegarra et al., 2010). The implementation of large-scale soil conservation and management programs and policies required considerable investments in labor and capital (Bilsborrow, 1992; Zimmerer, 1993; Posthumus and De Graaff, 2005). While the direct and indirect environmental benefits have been demonstrated on a case by case basis (Farley and Bremer, 2017; Romero-Díaz et al., 2019), comprehensive evaluations of environmental programs rarely reach beyond case by case assessments (Bonnesoeur et al., 2019). For example, the PRONAMACHCS program of the Ministry of Agriculture of Peru promoted the implementation of a specific type of intervention, the infiltration trenches. They consist of dozens of earthen ditches dug over mountain slopes following contour lines with the objective of increasing water infiltration in the soils. They have been implemented in several catchment areas throughout the country for over three decades, before the impact of these practices was systematically assessed at the regional scale (Vásquez and Tapia, 2011). In a global systematic review, Locatelli et al. (2020) found that case studies provide evidence that infiltration trenches are effective in reducing surface run-off and laminar erosion at plot scale but they also highlight that their impacts on water infiltration are uncertain as well as their effects at catchment scale or on other erosion forms. There is an urgent need to identify which soil conservation and management practices are most effective to combat soil erosion and to mitigate the on-site and off-site effects in the Andean region.
Soil conservation measures are receiving renewed interest in the context of nature-based solutions. They are defined by the International Union for Conservation of Nature (IUCN) as “services that nature provides, such as peatlands sequestering carbon, lakes storing large water supplies and floodplains absorbing excess water run-off” (Cohen-Shacham et al., 2016). Natural infrastructure is part of nature-based solutions and their infrastructure-like function helps to protect, sustainably manage or restore ecosystems while simultaneously providing human well-being and biodiversity benefits. In the Andean context, three large groups of water-related interventions can be identified: interventions based on land use and protective land cover including (1) restoration and protection of native ecosystems, such as montane forests or grasslands and (2) forestation with native or exotic species and (3) soil and water conservation measures including crop management, conservation tillage and slow-forming terraces and the implementation of linear elements such as vegetation strips and check dams. Several studies have shown that working with the natural infrastructure can help mitigate soil erosion and reduce risks of natural hazards (Vanacker et al., 2014; Cohen-Shacham et al., 2016).
To expand the knowledge base on natural infrastructure for erosion mitigation in the Andes, moving beyond case by case empirical studies to comprehensive assessments is needed (Bonnesoeur et al., 2019). This study systematically reviews the state of evidence on the effectiveness of interventions to mitigate soil erosion by water and is based on Andean case studies published in gray and peer-reviewed literature. This study addresses the following research questions: (1) which soil erosion indicators are useful to assess the overall effectiveness of natural infrastructure interventions from empirical studies in the Andes, (2) what is the overall impact of implementing natural infrastructure on on-site and off-site erosion mitigation and (3) which locations and types of studies are needed to fill critical gaps in knowledge and research?
The systematic review focuses on natural infrastructure interventions that are expected to influence erosion mitigation. We adapted the typology to the Andean region and defined three large groups of interventions: (i) the restoration and protection of native ecosystems, (ii) the forestation with native or exotic species and (iii) the implementation of soil and water conservation measures. We quantified their effects on the mitigation of water erosion by investigating measurable indicators of soil erosion. Besides commonly used indicators of soil erosion, such as soil loss rate, sediment yield, water turbidity and run-off coefficients, we also considered measures of soil quality, such as soil organic carbon, soil nutrient content and bulk density. The definition of terms and search criteria are provided in the Supplement A and B, the database structure in Supplement C and the studies that were included in the systematic review in Supplement D.
Based on the systematic review of published case studies from the Andean region, we first summarized the current state of knowledge, explored general patterns and identified research gaps. We applied the reporting guidelines established in the preferred reporting items for systematic reviews and meta-analyses (PRISMA, Gurevitch et al., 2018; Moher et al., 2015). Then, we performed analyses of variance to explore systematic differences in soil erosion indicators in relation to the interventions in natural infrastructure. Lastly, we estimated the overall effect of the interventions on soil quality, and on on-site and off-site erosion mitigation.
2.1 Literature search
The peer-reviewed literature search was conducted using the Scopus bibliographic database, and targeting studies published between 1980 and 2020. We searched within the article title, abstract and keywords for the following terms:
For the gray literature, we searched in 35 different databases from specialist organizations, public institutions and local repositories of private and public universities in the Andean region. The abovementioned search criteria were adapted for the gray literature given the limited search capabilities of some of the databases. Full details on the literature search are provided in the Supplement B, including the complete search terms, the number of records generated for specific searches and the name, location and search dates in Scopus and the national and regional databases of research institutions, universities and specialist organizations. For international peer-reviewed literature, we used a test library of 20 references (Supplement E) that confirmed that the search strings captured relevant literature.
2.2 Inclusion and exclusion criteria
The number of studies that were identified, screened, selected and included in the analysis are shown in a PRISMA flow diagram (Fig. 1). Between 10 January and 27 February 2020 we identified 1798 potentially relevant studies: 91 % corresponding to peer-reviewed articles and 9 % to gray literature. After removing duplicate studies, the dataset was reduced to 813 studies. These records were screened and articles that fulfilled the following criteria were included in the database: (1) they present quantitative data on soil erosion or soil quality comparing sites with different land use and protective land cover, soil and water conservation measures or elements of hydraulic regulation, (2) they are experimental studies including observational datasets or are modeling studies that are fully validated with field experiments or measurements and (3) they were realized in the Andean region. During the screening stage, we excluded 623 studies because of absence of quantitative on-site or off-site soil erosion or soil quality measurements.
We assessed 190 studies in full-text and further excluded 54 papers as the studies did not report quantitative measures of erosion rates or soil quality for different classes of land use and protective land cover, soil and water conservation measures or elements of hydraulic regulation. At this stage, this mainly concerned scientific reports on landslides and landslide-related erosion events.
2.3 Database development
A total of 136 studies were included in the systematic review. Where a study encompassed several independent case studies, the case studies were included in the final database as separate entries. Each case study was coded by a unique study identifier and recorded in the georeferenced database (Supplement C). We recorded the following ancillary geographic data: (1) country, (2) site name, (3) coordinates (latitude and longitude in decimal degrees), (4) elevation (meters above sea level, m a.s.l.), and information on (5) bioclimate, (6) surface lithology, (7) ecosystem and (8) landform. The latter four variables were derived from the 2005 Nature Conservancy datasets via the USGS dataviewer for South America (https://rmgsc.cr.usgs.gov/, last access: 27 April 2021). We included additional information on the type of study: (8) the experimental design following the classification scheme of Nichols et al. (2011), (9) the modeling approach based on a classification in statistical, process-based and mixed models, (10) the existence of field data and (11) the spatial scale and organization of the study based on a classification in plot (<0.01 km2), small catchment (between 0.01 and 1000 km2), large catchment (>1000 km2), and landscape scale (regional) analyses. The latter contained data collections that are not organized by hydrological units and that include measurements taken over a larger geographical area.
In the analyses, we quantified the effect of restoration and protection of natural vegetation, such as forest or native grasslands (PRO), forestation with native or exotic species (FOR) and implementation of soil and water conservation measures (SWC) for soil erosion and mitigation (Fig. 2). Soil and water conservation measures (SWC) include crop management, conservation tillage and slow-forming terraces and the implementation of linear elements, such as vegetation strips and check dams. We compared the three natural infrastructure interventions (PRO, FOR and SWC) with untreated areas under traditional agriculture, either cropland (CROP) or rangeland (RANGE), and bare land (BARE). Bare land corresponds to abandoned cropland or degraded land with very low (<10 %) vegetation cover.
The erosion indicators included in this study were (Fig. 2): soil loss rate (Sloss), determined as soil loss in t km−2 yr−1; plot run-off coefficient (RC), determined as event-based run-off coefficient from rainfall simulation experiments, in %; specific sediment yield (SSY), determined as the catchment-wide sediment yield per surface area in t km−2 yr−1, in abandoned cropland or degraded land with very low (<10 %) vegetation cover; and catchment-wide run-off ratio (RCC), determined as the annual total run-off ratio of the catchment, in %. While Sloss and SSY are direct measures of soil erosion at the plot and catchment scale, the plot and catchment-wide run-off coefficients (RC and RCC) are indirect indicators of soil erosion by water: the rainfall regime plays a role as raindrop impact and run-off water are involved in the detachment of soil particles and transport of sediment in surface water flow. Empirical studies compiled by, for example Bonnesoeur et al., 2019 and Valentin et al., 2008 have shown the strong association between run-off coefficients and soil erosion rates.
In addition to the four erosion indicators, two soil quality indicators were included: SOC (total soil organic carbon of the uppermost soil horizon, between 5 and 30 cm, in %), and BD (dry bulk density of the topsoil horizon, between 5 and 30 cm, in g cm−3). The SOC is the main indicator of soil quality (Franzluebbers, 2002) and is directly linked to key soil functions (Wiesmeier et al., 2019) including soil water retention, erosion prevention and resilience to drought and floods (Paustian et al., 2016). The BD is a commonly reported soil physical property that is related to soil aeration, water and air permeability and soil microporosity (Horn et al., 1995). Increased bulk density can be indicative of soil compaction and affect the water retention capacity and accelerate soil erosion (Molina et al., 2007; Patiño et al., 2021). Other erosion indicators were recorded in the database but not included in the statistical analyses because of a lack of statistical representation. These include plot-based indicators like the stock in SOC over the entire soil depth or the saturated hydraulic conductivity of the topsoil or catchment-wide indicators like the presence or relative occurrence of erosion signs or the suspended sediment concentration in the river channels. Mean, sample size and deviation metrics were extracted from figures using PlotDigitizer. Information from in-text tables and supplementary material was copied and tabulated in spreadsheets.
Of the 136 studies included in the systematic review, 118 studies contained sufficient information on the soil erosion and soil quality indicators to be statistically analyzed. Besides the abovementioned information, the georeferenced database includes bibliographic details and a URL link to the individual case studies (Supplement D).
2.4 Statistical analyses
First, we tested whether sites with natural infrastructure interventions (PRO, FOR and SWC) are different in on-site (Sloss, RC) and off-site (SSY, RCC) soil erosion and soil quality (SOC, BD) compared to untreated areas under traditional agriculture (CROP, RANGE) or bare land (BARE) as illustrated in Fig. 2. The comparison of the four erosion and two soil quality indicators between the treatments was performed using one-way analysis of variance (ANOVA). In this analysis, we pooled all observations from the 118 case-studies. Because of the limited number of quantitative case studies for the Andes, the number of observations is not the same for each group. Given the low number of observations per group, the Kruskal–Wallis ANOVA on the ranks was applied, with Dunn's post hoc test. We rejected the null hypotheses (i.e., that there are no differences between the means of the groups) at the 0.05 significance level. We used R (R Core Team, 2021) with the “PMCMRplus” package (Pohlert, 2018) in R to perform the non-parametric comparisons.
Next, we analyzed the overall effect of natural infrastructure interventions on soil erosion and soil quality indicators. In this analysis, we only included case studies with a control–treatment design, where quantitative measures of soil erosion and quality were available to establish the control–treatment contrast. The response ratio (RR) was then used to determine the effect sizes. In this study, the RR was calculated for each natural infrastructure intervention (PRO, FOR, SWC) and soil erosion and quality indicator (Sloss, RC, SSY, RCC, SOC, BD). For the control group, we combined data of sites with traditional agriculture, either cropland (CROP) or rangeland (RANGE) and bare land (BARE) given the limited number of matched pairs of control and single or multiple treatment(s). For each pairwise comparison, we plotted the effect size of the individual studies in forest plots and explored the heterogeneity in the response among the case studies. These plots were used to identify the magnitude and sources of variation among the studies and to identify possible outliers. We then extracted the central tendency (mean effect) and confidence limits (standard error) for each indicator and pairwise comparison. The mean effect and its standard error were plotted in summary forest plots (per pairwise comparison) to assess the overall effectiveness of a specific intervention on soil erosion and quality indicators. The graphs were produced using the R-package “metafor” (Viechtbauer, 2010).
3.1 Overall descriptive statistics
Of the 118 studies evaluating the effect of natural infrastructure interventions on soil erosion and quality indicators, 54 studies contained data on soil and water conservation practices (SWC), 50 studies on protective vegetation (PRO, FOR or both) and 14 studies on all 3 (SWC, PRO and FOR). The majority of studies were journal articles (79 %), followed by gray literature (14 %) and chapters from books (7 %). The studies covered a 6500 km long stretch across the Andes, with 4 % of the studies in Venezuela (n=5), 6 % in Colombia (n=7), 36 % in Ecuador (n=43), 35 % in Peru (n=41), 7 % in Bolivia (n=8), 8 % in Chile (n=9) and 4 % in Argentina (n=5). Ecuador and Peru had the highest number of case studies (Fig. 3). The large majority, i.e., 89 %, of the studies investigated soil erosion in tropical climates, with 59 % of the studies performed in pluvial seasonal, 19 % in pluvial and 10 % in desertic or xeric climates. The remaining studies were performed in temperate or Mediterranean climate regimes. Field studies mostly involved erosion measurements at the plot scale (48 %, n=57), small catchment scale (18 %, n=21), and landscape scale (22 %, n=26). Only 12 % of the studies included erosion assessment at the scale of large catchments (>1000 km2).
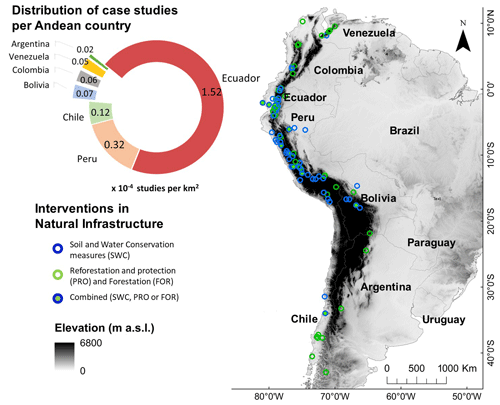
Figure 3Spatial distribution of case studies in the Andean region, classified per type of natural infrastructure intervention. The background map corresponds to the 30 arcsec DEM of South America (GTOPO30, U.S. Geological Survey's Center for Earth Resources Observation and Science, EROS). m a.s.l. meters above sea level.
3.2 Erosion mitigation assessed from different soil erosion indicators
The one-way analysis of variance revealed significant differences between treatment and control in soil quality and on-site soil erosion with notable differences in SOC (), BD (p=0.02, n=46), soil loss (p<0.01, n=123) and plot run-off coefficient (p=0.03, n=37) (Table 1; Fig. 4). Notably, none of the erosion indicators that were measured at the catchment scale were significant at the 0.05 level, as we observed only small differences between categories for specific sediment yields (p=0.10, n=37) and no differences for catchment-wide run-off coefficient (p=0.59, n=18). The latter might be due to the limited number of observations documenting the effect of natural infrastructure interventions on SSY (n=37) or RCC (n=18) and inherent variability in run-off and sediment discharge at the catchment scale as shown by Tolorza et al. (2014) and Molina et al. (2015). Below, we only present tendencies that are statistically significant at the 0.05 level.
Table 1Summary of the mean indicator values per treatment, with indication of the number of individual case studies between brackets. The values are reported for three interventions in natural infrastructure. PRO is restoration and protection of natural vegetation like forest or native grasslands, FOR is forestation with native and/or exotic species, and SWC is implementation of soil and water conservation measures, and for three untreated areas: CROP (cropland), RANGE (rangeland under traditional agricultural management), and BARE (bare land corresponding to abandoned cropland or degraded land with very low (<10 %) vegetation cover). Difference between groups was tested with the Kruskal–Wallis rank sum test, and p-values are estimated using the chi-squared distribution.
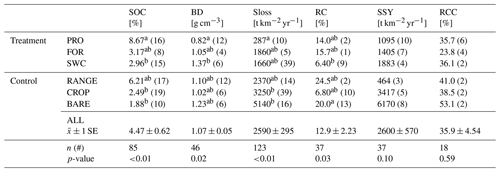
Means followed by a common letter are not significantly different by the Dunn's non-parametric all-pairs comparison test at 5 % level of significance.
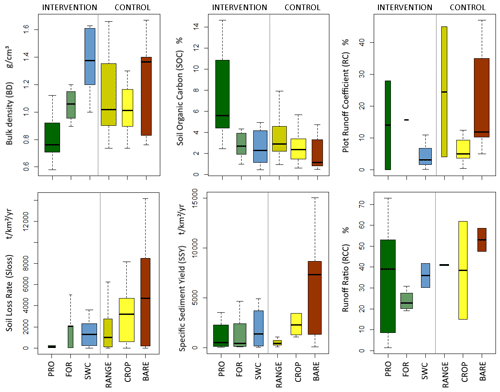
Figure 4Variation in bulk density, soil organic carbon content, runoff coefficient, soil loss rate, specific sediment yield and catchment runoff coefficient between the three categories of natural infrastructure intervention (PRO, FOR, SWC), the untreated agricultural (RANGE, CROP) and bare land (BARE). Bold lines represent the median values, boxes extend to first and third quantiles and whiskers to 1.5 times the interquartile range from the box.
The SOC concentration of topsoil is the indicator with the highest significance level, showing strong differences in soil quality between protected sites, cropland and bare soil (Table 1, Fig. 4). Based on 85 observations, we observed soil organic carbon concentrations of the topsoil between 0.47 % and 34.06 %, with mean values of 4.47 % ± 0.62 %. Based on the results of the post hoc Kruskal–Wallis rank sum test, two distinct groups can be identified: (1) areas covered by natural vegetation such as forests and native grasslands with a mean SOC value of 8.67 % ± 1.89 %, and (2) areas covered by agricultural crops and bare land having a mean SOC value of 2.49 % ± 0.32 % and 1.88 % ± 0.49 %,respectively. Areas with SWC measures belong to the second group with a SOC of 2.96 % ± 0.70 %. Rangelands and plantation forests have intermediate SOC values of 6.21 % ± 2.05 % and 3.17 % ± 0.71 %, respectively. These results are consistent with the systematic review of Bonnesoeur et al. (2019) that reported lower levels of topsoil organic matter in plantations compared to native forests and grasses. However, the differences reported here are not statistically significant (p=0.12).
Soil BD of the topsoil is reported in 15 % of the reported case studies for different natural infrastructure interventions. Soil BD ranges between 0.36 and 1.67 g cm−3 with a mean value of 1.07±0.05 (Fig. 4; Table 1). The lowest mean BD values, i.e., 0.82±0.08 g cm−3, are observed in soils covered by native vegetation. Although the mean BD values are notably higher in areas with cropland, forestation, rangeland, and particularly bare land, the wide range in reported BD values per category does not allow us to distinguish them from areas covered by natural vegetation at the 0.05 significance level. Remarkably, areas under SWC treatment have significantly higher BD values compared to natural vegetation (Table 1), which might reflect the advanced state of physical soil degradation due to compaction before SWC intervention (e.g., Rymshaw et al., 1997). It also highlights that it may take several years to decades for impacts to be reversed and that high levels of subsurface compaction may be irreversible without soil restoration (Borja, 2018).
The rate of soil loss measured at the plot scale (t km−2 yr−1) is one of the most common indicators of soil erosion, as it is reported in 43 % of the case studies. The 125 quantitative measurements of Sloss reveal that Sloss rates vary widely with mean value of 2590±295 t km−2 yr−1 and minimum and maximum values of 0.001 and 14761 t km−2 yr−1, respectively. Significant differences in Sloss are observed between areas covered by natural vegetation and crop or bare land, with soil losses being on average 11 to 18 times lower in areas with protected vegetation (Table 1). Rangelands, areas with forestation and SWC measures have intermediate values of Sloss (2370, 1860 and 1660 t km−2 yr−1),respectively, and are not significantly different from natural vegetation, crop or bare land.
The run-off coefficient (RC) is measured as surface run-off at the plot scale, and is here reported as the percentage of the rainfall that becomes run-off. The number of case studies that report run-off coefficients for different categories of natural infrastructure is low (12 %). Figure 4 illustrates the wide range of RC values (min: 0 %, max: 47 %) that are observed in the Andes, with mean values of 12.9±2.23. The large variation might be the result of inherent spatial heterogeneity in rainfall-run-off response (Guzman et al., 2019). However, methodological bias cannot be excluded as multiple field methods to estimate plot RC were used: portable rainfall simulators covering a few cm2 (e.g., Harden, 2001), runoff plots covering 1 m2 (e.g., Perrin et al., 2001; Molina et al., 2007), and experimental sites covering >10 m2 (Molina et al. 2009; Suescún et al. 2017). Also, the amount and intensity of the (simulated) rainfall often vary between case studies. Notwithstanding, significant differences are observed in RC between areas with soil and water conservation measures and bare land, with RC values being on average 3.5 times lower in SWC compared to BARE (Fig. 4).
3.3 Reported effectiveness of natural infrastructure interventions on soil erosion and soil quality
When limiting the quantitative analysis to matched pairs of control and single or multiple treatment, the number of independent empirical studies is reduced from 118 to 89. For the analysis of the response ratios, the sites with traditional agriculture, either cropland (CROP) or rangeland (RANGE), and bare land (BARE) were regrouped into one control group. This is justified by the fact that the soil quality and erosion indicators are not significantly different between the three types of control sites (Table 1). Figure 5 and Table 2 show the effect size of (i) restoration and protection of natural vegetation, (ii) forestation, and (iii) SWC on SOC, BD, RC, Sloss rate, SSY and RCC. Below, we only discuss results that are based on a minimum of 4 independent treatment-control studies.
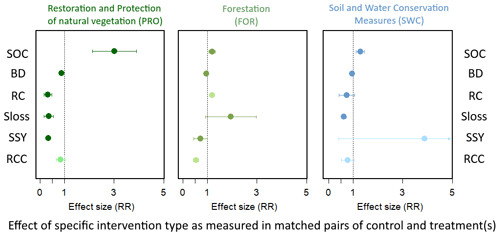
Figure 5Response ratio of natural infrastructure interventions (PRO, FOR, SWC) relative to cropland and rangeland. The plots show the mean response ratio (points) and its standard error (solid lines) for soil organic carbon (SOC) and bulk density (BD) in the topsoil, runoff coefficient (RC) and soil loss rate (Sloss), specific sediment yield (SSY) and catchment-wide runoff ratio (RCC). When the number of individual treatment-control studies is below 4, the symbols are shown in lighter colors.
Table 2Summary of response ratios, showing the effect of restoration and protection of natural vegetation (PRO), forestation (FOR), and soil and water conservation (SWC) on soil quality and erosion. The mean value and the 68 % confidence interval (CI) are given, as well as the number of treatment-control studies (#).
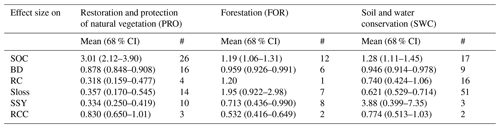
Amongst the three intervention types, the protection and conservation of natural vegetation (PRO) has the strongest effect on soil quality (SOC, BD) and erosion (Sloss, SSY). When native forests and grasses are protected from conversion to agricultural land, the topsoil contains 3.01±0.893 times more SOC than in the control sites. At the same time, the soil physical structure is better with a dry BD of 0.82±0.08 g cm−3 in natural vegetation, being 0.878±0.030 times lower compared to control sites. The high soil porosity enhances structural support, water and solute movement and soil aeration (Podwojewski et al., 2002). There is a clear and positive effect on soil erosion mitigation, with Sloss being 0.357±0.187 times lower, and SSY being 0.334±0.085 times lower than at the control sites. Experimental work by, for example, Janeau et al. (2015) showed the importance of native vegetation in facilitating soil water infiltration as it can conduct over 50 % of rainwater through stemflow to the soil. This is confirmed by other empirical data (e.g., Harden, 1996; Poulenard et al., 2001) collected at the plot scale, and the RC is on average 0.318±0.159 times lower in areas where natural vegetation is protected or conserved. The empirical data are not sufficient to systematically assess the effect on run-off processes at the catchment scale.
Only 17 % of the records on treatment-control experiments contain information on the effect of forestation with native and/or exotic species (FOR) on soil quality, on-site and off-site soil erosion (Table 2). The database counts less than 3 empirical studies on rainfall-run-off generation, at the plot and catchment scale. Compared to the control sites, a positive effect is reported on soil quality, with 1.19±0.125 times higher SOC and 0.959±0.032 lower BD. The pairwise analysis did not show evidence of a net effect of forestation on soil erosion (Sloss): the response ratio shows large scatter with RR values ranging between 0.37 in the study by Henry et al. (2013) and 7.75 in the case published by Pesantez and Seminario (2010). Notwithstanding the high variability in response ratios for on-site erosion (Sloss), a positive effect was observed for the catchment-wide sediment yields with SSY being on average 71.3 % ± 27.7 % of the yields measured in control sites. Similar observations were made by Bonnesoeur et al. (2019) who attributed the scatter in the empirical studies to the type of forestation (native vs. exotic species) and forestation age. In addition to this, the prior state of the environment (soil quality and erosion) has a major impact on erosion mitigation as Balthazar et al. (2015) showed for a case in the Ecuadorian Andes.
Almost 50 % of the treatment-control studies concern interventions with soil and water conservation measures (SWC). There is a net positive effect of the implementation of conservation measures on the soil organic carbon content of the topsoil, with values that are 1.28±0.170 times higher compared to control sites (Fig. 5). The effect on the BD is small, with BD in treated sites being 94.6 % ± 3.2 % of the values measured in control sites. The limited effect on soil BD suggests that the recovery of the soils' physical structure from compaction is slow, even within the topsoil (Jacobi et al., 2015). Soil loss rates changed significantly after the application of SWC measures: Slosses are reduced to 62.1 % ± 9.2 % of their original values after the intervention. The effect of infiltration ditches on soil erosion mitigation is particularly well-documented for the Peruvian Andes, where Vasquez and Tapia (2011) reported soil erosion rates that were more than two times reduced after the intervention (i.e., from 4500 to 2060 t km−2 yr−1). The effect is strongest when the measures are applied on abandoned cropland or degraded land with very low (<10 %) vegetation cover (Fig. 3), as shown by De Noni et al. (2001) in various case studies distributed along the Ecuadorian Andes. In contrast to the response ratios for Sloss, the plot-scale RC shows large scatter with values of 0.740±0.316. The scatter can be attributed to strong differences in hydrological response between control sites, with degraded and abandoned land generating more run-off than rangeland or arable land (Molina et al., 2007).
3.4 Knowledge gaps and prospects for future research
3.4.1 Representation of natural variability in environmental conditions within the Andean region
The literature reviewed in this study showed an unequal distribution of empirical studies over the Andean countries, with an underrepresentation of studies from Argentina, Venezuela, Colombia and Bolivia (Fig. 3). Gray literature (e.g., technical reports) from these countries was often inaccessible via standard search methods, in contrast to gray literature from Peru or Ecuador. When compared to the Andean region, the dataset of 118 case studies contains a disproportionally high amount of studies from mid-elevations (i.e., between 2000 and 4000 m a.s.l.) and moderate relief with hillslope gradients below 15∘ (Fig. 6). High elevation sites, and areas with either low or high relief are underrepresented. Similarly, the regions with intermediate precipitation amounts are overrepresented, and there is a disproportionally low amount of studies with either low to very low (<400 mm yr−1) or very high (>3000 mm yr−1) precipitation (Fig. 6). Given the spatial bias in the data compilation, the records do not allow a statistically unbiased regional scale assessment of water erosion mitigation to be performed. It is necessary that future studies collect empirical data on soil quality, erosion and sediment yield before/after interventions in the abovementioned data-scarce regions.
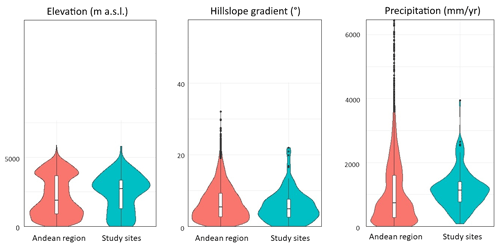
Figure 6Distribution of the mean elevation (m a.s.l.), mean annual precipitation (mm yr−1) and mean hillslope gradient (∘) for the 118 empirical studies and the entire Andean region. The delineation of the Andean region is based on Körner et al. (2017). The topographic information is derived from the 30 arcsec DEM of South America (GTOPO30, U.S. Geological Survey's Center for Earth Resources Observation and Science, EROS), and the mean annual precipitation data from the Tropical Rainfall Measuring Mission (TRMM 3B43) dataset (Ceccherini et al., 2015).
There is a particular lack of knowledge on soil erosion processes before, after or during extreme rainfall or seismic events. Of the 118 quantitative studies, only 20 studies or 17 % explicitly referred to flooding or erosion processes during extreme (i.e., high-magnitude but rare and episodic) events. Reliable, quantitative information about the return period of extreme erosion and flooding events, and their influence on soil quality, long-term erosion rates and sediment discharge is scarce (Aguilar et al., 2020; Carretier et al., 2018). The severe scarcity of studies on the impact of extreme events has major implications for providing information on land use management practices (Coppus and Imeson, 2002), as the effectiveness of policy-based interventions on natural infrastructure could not be methodically evaluated for extreme events. A number of model applications by, for example, Bathurst et al. (2011, 2020) conveyed the limitations of forestation as an intervention for reducing peak discharges of floods derived from extreme but infrequent rainfall events. There is a clear need to thoroughly evaluate whether our results on the effectiveness of natural infrastructure interventions during frequent erosion events can be extended to extreme events related to, for example, El Niño–Southern Oscillation (ENSO).
3.4.2 Gap between plot-scale and catchment-scale erosion assessments
There is a clear gap between the number of case studies on water erosion at the plot-scale and the catchment-scale with about 48 % of all articles on plot-scale erosion phenomena, and only 30 % on sediment yield at small and large catchment scale. The remaining 22 % of the studies are conducted at landscape scale, with observations made at different topographic positions within a larger geographical region. Due to their replicability, erosion plot studies are the most used and standardized experimental method, whereby run-off and sediment are measured from bounded run-off plots of ≤ 1 m2 (e.g., Harden, 1996; Poulenard et al., 2001) to 1000 m2 (e.g., De Noni et al., 2001). The strong focus on soil erosion mitigation on farmland is in line with past and ongoing efforts on sustainable and resilient agriculture by programs like PRONAMACHCS and MARENASS in Peru, PRONAREG-MAG-ORSTOM and USAID in Ecuador and IIDE and USAID in Bolivia. Even when local interventions have proven very successful, they are rarely implemented at a large scale. Only a handful of studies (e.g., Molina et al., 2007; 2008) in our database evaluated water erosion simultaneously at the plot and catchment scales. Therefore, it is necessary that future studies are designed to assess the effectiveness of water-related interventions on the specific sediment yield or catchment-wide runoff ratio at the broader catchment scale.
Transferring knowledge on erosion mitigation from the plot-scale to the catchment scale remains a challenge. First, local scale erosion phenomena might not be representative for the dominant erosion processes at the catchment scale. For example, while farming terraces or infiltration ditches enhance infiltration and reduce run-off and erosion on hillslopes (e.g., Sandor and Eash, 1995), localized sediment sources such as run-off generating unpaved roads or debris flows might overwhelm the sediment yield (e.g., Vanacker et al., 2007b). This can also be observed by the divergence in response ratios of Sloss rates and SSYs, after implementation of SWC measures (Fig. 5). Second, when the sediment that is generated by water erosion on the hillslopes is transferred downslope to the river network, sediment storage, erosion and remobilization can occur across the river system (Romans et al., 2016; Verstraeten et al., 2017). The effect of a specific intervention (like forestation) on soil erosion on the hillslopes is therefore not directly leading to a similar change in sediment yield at the outlet of the catchment (Fig. 5). Further empirical work is needed to decipher how environmental signals, such as changes in erosion rates after natural infrastructure interventions, are transferred through hillslopes, floodplains and river channels.
The systematic review of gray and peer-reviewed literature on natural infrastructure interventions and erosion mitigation in the Andean region resulted in 1798 potentially relevant case studies. After screening the records, 118 empirical studies were eligible and included in the quantitative analysis on soil quality and soil erosion. From the suite of physical, chemical and biological indicators commonly used in soil erosion research, six indicators were pertinent to study the effectiveness of natural infrastructure: soil organic carbon and bulk density of the topsoil, soil loss rate and run-off coefficient at the plot scale, and specific sediment yield and catchment-wide run-off coefficient at the catchment scale. The one-way analysis of variance revealed significant differences between treatment and control in soil organic carbon (p<0.01, n=85), bulk density (p=0.02, n=46), soil loss (p<0.01, n=123) and plot run-off coefficient (p=0.03, n=37). None of the erosion indicators that were measured at the catchment scale were significant at the 0.05 level.
The protection and conservation of natural vegetation has the strongest effect on soil quality and erosion. When native forests and grasses are protected from conversion to agricultural land, the topsoil contains 3.01±0.893 times more soil organic carbon, and has a better physical structure than the control sites. At the same time, there is a clear effect on erosion mitigation, with soil losses being 0.357±0.187 times lower, and specific sediment yields being 0.334±0.085 times lower than at control sites.
The effect of forestation with native and/or exotic species is less documented. A positive effect is reported on soil quality, with 1.19±0.125 times higher SOC and 0.959±0.032 lower BD compared to control sites. The pairwise analysis did not show evidence of a net effect on soil erosion, although a positive effect was observed for catchment-wide sediment yields being 71.3 % ± 27.7 % of the yields measured in control sites. The implementation of SWC measures has a net positive effect on the SOC content of the topsoil, with values that are 1.28±0.170 times higher than control sites and on Sloss rates that are reduced to 62.1 % ± 9.2 % of their original values after the intervention.
The systematic review of the existing literature allowed us to identify critical gaps in knowledge and research. We observed spatial bias in the data compilation. There is a need for future empirical work on soil quality, erosion and sediment yield before/after interventions in data-scarce regions, such as high elevations, regions with either low or high relief, and low to very low or very high precipitation. Besides, most erosion assessments are based on short-term measurements that tend to miss the impact of rare high-magnitude events. It is necessary to evaluate whether the results of this study on the effectiveness of natural infrastructure interventions hold during extreme events related to, for example, El Niño–Southern Oscillation (ENSO). In addition, future climate variability and global warming might trigger erosion events, as freshly exposed deglaciated terrain is particularly prone to soil erosion.
The data underpinning this study have been archived with Zenodo and are available at https://doi.org/10.5281/zenodo.6203174 (Vanacker et al., 2022). The data may also be requested from the corresponding author by email.
-
Supplement A. Definitions of terms used in the systematic review (in English and Spanish)
-
Supplement B. List of databases that were searched, with indication of search terms
-
Supplement C. Structure of the database
-
Supplement D. List of the literature studies that were included in the systematic review
-
Supplement E. Test library of 20 references compiled by experts in the fields
The supplement related to this article is available online at: https://doi.org/10.5194/soil-8-133-2022-supplement.
VV and AM conceived the study and conducted the statistical analyses, with backstopping of VB, FRD, BFOT and WB. AM and MRB compiled and verified the database from peer-reviewed and gray literature. All authors contributed to shaping the research and analyses, as well as writing the paper.
The contact author has declared that neither they nor the co-authors have any competing interests.
Publisher's note: Copernicus Publications remains neutral with regard to jurisdictional claims in published maps and institutional affiliations.
We thank CIFOR, Forest Trends, Universidad Nacional Agraria La Molina, Universidad Nacional Mayor de San Marcos, Universidad de Chile and Universidad de los Andes for digitizing and sharing technical reports and undergraduate theses.
This research was supported by the United States Agency for International Development (Natural Infrastructure for Water Security Project), the Government of Canada (Natural Infrastructure for Water Security Project), the Secretaría de Educación Superior, Ciencia, Tecnología e Innovación (PhD scholarship to Boris F. Ochoa-Tocachi), the Université catholique de Louvain (grant no. ADRI/CD/CA/2016-NR 51), the Académie de recherche et d'enseignement supérieur de la Fédération Wallonie-Bruxelles (ARES PRD ParamoSUS, and PhD scholarship to Miluska A. Rosas), and the UKRI Natural Environment Research Council (grant no. NE/S013210/1).
This paper was edited by Olivier Evrard and reviewed by two anonymous referees.
Aguilar, G., Cabré, A., Fredes, V., and Villela, B.: Erosion after an extreme storm event in an arid fluvial system of the southern Atacama Desert: an assessment of the magnitude, return time, and conditioning factors of erosion and debris flow generation, Nat. Hazards Earth Syst. Sci., 20, 1247–1265, https://doi.org/10.5194/nhess-20-1247-2020, 2020.
Balthazar, V., Vanacker, V., Molina, A., and Lambin, E. F.: Impacts of forest cover change on ecosystem services in high Andean mountains, Ecol. Indic., 48, 63–75, https://doi.org/10.1016/j.ecolind.2014.07.043, 2015.
Bathurst, J. C., Iroumé, A., Cisneros, F., Fallas, J., Iturraspe, R., Gaviño Novillo, M., Urciuolo, A., de Bievre, B., Guerrero Borges, V., Coello, C., Cisneros, P., Gayoso, J., Miranda, M., and Ramirez, M.: Forest Impact on Floods Due to Extreme Rainfall and Snowmelt in Four Latin American Environments 1: Field Data Analysis, J. Hydrol., 400, 281–291, https://doi.org/10.1016/j.jhydrol.2010.11.044, 2011.
Bathurst, J. C., Fahey, B., Iroumé, A., and Jones, J.: Forests and Floods: Using Field Evidence to Reconcile Analysis Methods, Hydrol. Process., 34, 3295–3310, https://doi.org/10.1002/hyp.13802, 2020.
Bilsborrow, R. E.: Population Growth, Internal Migration, and Environmental Degradation in Rural Areas of Developing Countries, Eur. J. Popul., 8, 125–148, https://doi.org/10.1007/BF01797549, 1992.
Blodgett, T. A. and Isacks, B. L.: Landslide Erosion Rate in the Eastern Cordillera of Northern Bolivia, Earth Interact., 11, 1–30, https://doi.org/10.1175/2007EI222.1, 2007.
Bonnesoeur, V., Locatelli, B., Guariguata, M. R., Ochoa-Tocachi, B. F., Vanacker, V., Mao, Z., Stokes, A., and Mathez-Stiefel, S. L.: Impacts of Forests and Forestation on Hydrological Services in the Andes: A Systematic Review, Forest Ecol. Manag., 433, 569–584, https://doi.org/10.1016/j.foreco.2018.11.033, 2019.
Borja, P., Molina, A., Govers, G., and Vanacker, V.: Check dams and afforestation reducing sediment mobilization in active gully systems in the Andean mountains, Catena, 165, 42–53, https://doi.org/10.1016/j.catena.2018.01.013, 2018.
Carretier, S., Tolorza, V., Regard, V., Aguilar, G., Bermúdez, M. A., Martinod, J., Guyot, J. L., Hérail, G., and Riquelme, R.: Review of Erosion Dynamics along the Major N-S Climatic Gradient in Chile and Perspectives, Geomorphology, 300, 45–68, https://doi.org/10.1016/j.geomorph.2017.10.016, 2018.
Ceccherini, G., Ameztoy, I., Hernández, C. P. R., and Moreno, C. C.: High-Resolution Precipitation Datasets in South America and West Africa based on Satellite-Derived Rainfall, Enhanced Vegetation Index and Digital Elevation Model, Remote Sens., 7, 6454–6488, https://doi.org/10.3390/rs70506454, 2015.
Cohen-Shacham, E., Walters, G., Janzen, C., and Maginnis, S.: Nature-based Solutions to address global societal challenges, Gland, Switzerland: IUCN, 97 pp., 2016.
Coppus, R. and Imeson, A. C.: Extreme Events Controlling Erosion and Sediment Transport in a Semi-Arid Sub-Andean Valley, Earth Surf. Proc. Land., 27, 1365–1375, https://doi.org/10.1002/esp.435, 2002.
De Noni, G., Viennot, M., Asseline, J., and Trujillo, G.: Terre d'altitude, terres de risque. La lutte contre l'érosion dans les Andes équatoriennes, IRD éditions, Collection Latitudes 23, Paris, France, ISBN 2-7099-1469-7, 2001.
Devenish, C. and Gianella, C.: 20 years of sustainable mountain development in the Andes – from Rio 1992 to 2012 and beyond, Consorcio para el Desarollo Sostenible de la Ecoregion Andina, Lima, Peru, 66pp., 2012.
Farley, K. A. and Bremer, L. L.: “Water Is Life”: Local Perceptions of Páramo Grasslands and Land Management Strategies Associated with Payment for Ecosystem Services, Ann. Am. Assoc. Geogr., 107, 371–381, https://doi.org/10.1080/24694452.2016.1254020, 2017.
Franzluebbers, A. J.: Soil Organic Matter Stratification Ratio as an Indicator of Soil Quality, Soil Till. Res., 66, 95–106, https://doi.org/10.1016/S0167-1987(02)00018-1, 2002.
Guns, M. and Vanacker, V.: Shifts in Landslide Frequency-Area Distribution after Forest Conversion in the Tropical Andes, Anthropocene, 6, 75–85, https://doi.org/10.1016/j.ancene.2014.08.001, 2014.
Gurevitch, J., Koricheva, J., Nakagawa, S., and Stewart, G.: Meta-Analysis and the Science of Research Synthesis, Nature, 555, 175–182, https://doi.org/10.1038/nature25753, 2018.
Guzman, C. D., Hoyos-Villada, F., Da Silva, M., Zimale, F. A., Chiranda, N., Botero, C., Morales Vargas, A., Rivera, B., Moreno, P., and Steenhuis, T. S.: Variability of soil surface characteristics in a mountainous watershed in Valle del Cauca, Colombia: Implications for runoff, erosion, and conservation, J. Hydrol., 576, 273–286, https://doi.org/10.1016/j.jhydrol.2019.06.002, 2019.
Harden, C. P.: Interrelationship between land abandonment and land degradation: a case from the Ecuadorian Andes, Mt. Res. Dev., 16, 274–280, https://doi.org/10.2307/3673950, 1996.
Harden, C. P.: Soil Erosion and Sustainable Mountain Development, Mt. Res. Dev., 21, 77–83, https://doi.org/10.1659/0276-4741(2001)021[0077:SEASMD]2.0.CO;2, 2001.
Henry, A., Mabit, L., Jaramillo, R. E., Cartagena, Y., and Lynch, J. P.: Land Use Effects on Erosion and Carbon Storage of the Río Chimbo Watershed, Ecuador, Plant Soil, 367, 477–491, https://doi.org/10.1007/s11104-012-1478-y, 2013.
Horn, R., Domzzał, H., Słowińska-Jurkiewicz, A., and van Ouwerkerk, C.: Soil Compaction Processes and Their Effects on the Structure of Arable Soils and the Environment, Soil Till. Res., 35, 23–36, https://doi.org/10.1016/0167-1987(95)00479-C, 1995.
Jacobi, J., Schneider, M., Bottazzi, P., Pillco, M., Calizaya, P., and Rist, S.: Agroecosystem resilience and farmers' perceptions of climate change impacts on cocoa farms in Alto Beni, Bolivia, Renew. Agr. Food Syst., 30, 170–183, https://doi.org/10.1017/S174217051300029X, 2015.
Janeau, J. L., Grellier, S., and Podwojewski, P.: Influence of rainfall interception by endemic plants versus short cycle crops on water infiltration in high altitude ecosystems of Ecuador, Hydrol. Res., 46, 1008–1018, https://doi.org/10.2166/nh.2015.203, 2015.
Jantz, N. and Behling, H.: A Holocene Environmental Record Reflecting Vegetation, Climate, and Fire Variability at the Páramo of Quimsacocha, Southwestern Ecuadorian Andes, Veg. Hist. Archaeobot., 21, 169–185, https://doi.org/10.1007/s00334-011-0327-x, 2012.
Keating, P. L.: Fire Ecology and Conservation in the High Tropical Andes: Observations from Northern Ecuador, Journal of Latin American Geography, 6, 43–62, https://www.jstor.org/stable/25765157 (last access: 15 June 2021), 2007.
Körner, C., Jetz, W., Paulsen, J., Payne, D., Rudmann-Maurer, K. and Spehn, E. M.: A global inventory of mountains for bio-geographical applications, Alp Botany, 127, 1–15, https://doi.org/10.1007/s00035-016-0182-6, 2017.
Latrubesse, E. M. and Restrepo, J. D.: Sediment Yield along the Andes: Continental Budget, Regional Variations, and Comparisons with Other Basins from Orogenic Mountain Belts, Geomorphology, 216, 225–233, https://doi.org/10.1016/j.geomorph.2014.04.007, 2014.
Little, M. A.: Human Populations in the Andes: The Human Science Basis for Research Planning, Mt. Res. Dev., 1, 145–170, https://doi.org/10.2307/3673120, 1981.
Locatelli, B., Homberger, J. M., Ochoa-Tocachi, B. F., Bonnesoeur, V., Román, F., Drenkhan, F., and Buytaert, W.: Impactos de Las Zanjas de Infiltración En El Agua y Los Suelos: ?`Qué Sabemos? Resumen de políticas, Proyecto “infraestructura natural para la Seguridad hídrica”, Forest Trends, Lima, Peru, http://hal.cirad.fr/cirad-02615502 (last access: 15 June 2021), 2020.
Moher, D., Stewart, L., and Shekelle, P.: All in the Family: Systematic Reviews, Rapid Reviews, Scoping Reviews, Realist Reviews, and More, Sys. Rev., 4, 1–2, https://doi.org/10.1186/s13643-015-0163-7, 2015.
Molina, A., Govers, G., Vanacker, V., Poesen, J., Zeelmaekers, E., and Cisneros, F.: Runoff Generation in a Degraded Andean Ecosystem: Interaction of Vegetation Cover and Land Use, Catena, 71, 357–370, https://doi.org/10.1016/j.catena.2007.04.002, 2007.
Molina, A., Govers, G., Poesen, J., Van Hemelryck, H., De Bièvre, B. and Vanacker, V.: Environmental factors controlling spatial variation in sediment yield in a central Andean mountain area, Geomorphology, 98, 176–186, https://doi.org/10.1016/j.geomorph.2006.12.025, 2008.
Molina, A., Govers, G., Van den Putte, A., Poesen, J., and Vanacker, V.: Assessing the reduction of the hydrological connectivity of gully systems through vegetation restoration: field experiments and numerical modelling, Hydrol. Earth Syst. Sci., 13, 1823–1836, https://doi.org/10.5194/hess-13-1823-2009, 2009.
Molina, A., Vanacker, V., Brisson, E., Mora, D., and Balthazar, V.: Multidecadal change in streamflow associated with anthropogenic disturbances in the tropical Andes, Hydrol. Earth Syst. Sci., 19, 4201–4213, https://doi.org/10.5194/hess-19-4201-2015, 2015.
Montgomery, D. R., Balco, G., and Willett, S. D.: Climate, Tectonics, and the Morphology of the Andes, Geology, 29, 579–582, https://doi.org/10.1130/0091-7613(2001)029<0579:CTATMO>2.0.CO;2, 2001.
Morera, S. B., Condom, T., Crave, A., Steer, A., and Guyot, J. L.: The Impact of Extreme El Niño Events on Modern Sediment Transport along the Western Peruvian Andes (1968–2012), Scientific Reports, 7, 1–14, https://doi.org/10.1038/s41598-017-12220-x, 2017.
Nichols, S., Webb, A., Norris, R., and Stewardson, M.: Eco Evidence analysis methods manual: a systematic approach to evaluate causality in environmental science, eWater Cooperative Research Centre, Canberra, ISBN 978-1-921543-43-2, 2011.
Patiño, S., Hernández, Y., Plata, C., Domínguez, I., Daza, M., Oviedo-Ocaña, R., Buytaert, W., and Ochoa-Tocachi, B. F.: Influence of Land Use on Hydro-Physical Soil Properties of Andean Páramos and Its Effect on Streamflow Buffering, Catena, 202, 105227, https://doi.org/10.1016/j.catena.2021.105227, 2021.
Paustian, K., Lehmann, J., Ogle, S., Reay, D., Robertson, G. P., and Smith, P.: Climate-Smart Soils, Nature, 532, 49–57, https://doi.org/10.1038/nature17174, 2016.
Perrin, J. L., Bouvier, C., Janeau, J. L., Menez, G., and Cruz, F., Rainfall/runoff processes in a small peri-urban catchment in the Andes mountains. The Rumihurcu Quebrada, Quito (Ecuador), Hydrol. Process., 15, 843–854, https://doi.org/10.1002/hyp.190, 2001.
Pesantez, P. G. and Seminario, M. O.: Identificación de zonas degradadas y en proceso de erosión, Eng. Thesis, Faculty of Agronomy, Universidad de Cuenca, Cuenca, Ecuador, 2010.
Podwojewski, P., Poulenard, J., Zambrana, T., and Hofstede, R.: Overgrazing Effects on Vegetation Cover and Properties of Volcanic Ash Soil in the Paramo of the Llangahua and Esperanza (Tungurahua, Ecuador), Soil Use Manage., 18, 45–55, https://doi.org/10.1111/j.1475-2743.2002.tb00049.x, 2002.
Pohlert, T.: PMCMRplus: Calculate Pairwise Multiple Comparisons of Mean Rank Sums Extended, Retrieved from https://CRAN.R-project.org/package=PMCMRplus (last access: 24 December 2021), 2018.
Posthumus, H. and De Graaff, J.: Cost-benefit analysis of bench terraces, a case study in Peru, Land Degrad. Dev., 16, 1–11, https://doi.org/10.1002/ldr.637, 2005.
Poulenard, J., Podwojewski, P., Janeau, J. L., and Collinet, J.: Runoff and soil erosion under rainfall simulation of Andisols from the Ecuadorian paramo: effect of tillage and burning, Catena 45, 185–207, https://doi.org/10.1016/S0341-8162(01)00148-5, 2001.
R Core Team: R: A language and environment for statistical computing, R Foundation for Statistical Computing, Vienna, Austria, https://www.R-project.org/, last access: 24 December 2021.
Restrepo, J. D., Kettner, A. J., and Syvitski, J. P. M.: Recent Deforestation Causes Rapid Increase in River Sediment Load in the Colombian Andes, Anthropocene, 10, 13–28, https://doi.org/10.1016/j.ancene.2015.09.001, 2015.
Romans, B. W., Castelltort, S., Covault, J. A., Fildani, A., and Walsh, J. P.: Environmental Signal Propagation in Sedimentary Systems across Timescales, Earth-Sci. Revi., 153, 7–29, https://doi.org/10.1016/j.earscirev.2015.07.012, 2016.
Romero-Díaz, A., de Vente, J., and Díaz-Pereira, E.: Assessment of the Ecosystem Services Provided by Agricultural Terraces, Pirineos., 174, e043, https://doi.org/10.3989/pirineos.2019.174003, 2019.
Rymshaw, E., Walter, M. F., and Van Wambeke, A., Processes of soil movement on steep cultivated hill slopes in the Venezuelan Andes, Soil Till. Res., 44, 265–272, https://doi.org/10.1016/S0167-1987(97)00055-X, 1997.
Sandor, J. A. and Eash, N. S.: Ancient Agricultural Soils in the Andes of Southern Peru, Soil Sci. Soc. Am. J., 59, 170–179, https://doi.org/10.2136/sssaj1995.03615995005900010026x, 1995.
Suescún, D., Villegas, J. C. , León, J. D., Flórez, C. P., García-Leoz, V., and Correa-Londoño, G. A.: Vegetation Cover and Rainfall Seasonality Impact Nutrient Loss via Runoff and Erosion in the Colombian Andes, Reg. Environ. Change, 17, 827–839, https://doi.org/10.1007/s10113-016-1071-7, 2017.
Tolorza, V., Carretier, S., Andermann, C., Ortega-Culaciati, F., Pinto, L., and Mardones, M.: Contrasting Mountain and Piedmont Dynamics of Sediment Discharge Associated with Groundwater Storage Variation in the Biobío River, J. Geophys. Res.-Earth, 119, 2730–2753, https://doi.org/10.1002/2014JF003105, 2014.
Torero Zegarra, E., Pender, J., Maruyama, E., Keefe, M., and Stoorvogel, J. M.: Socioeconomic and Technical Considerations to Mitigate Land and Water Degradation in the Peruvian Andes, CPWF Project Report Series PN70, Colombo, Sri Lanka, 2010.
USGS: Global Ecosystems Viewer, https://rmgsc.cr.usgs.gov/ArcGIS/rest/services/contSA/MapServer, last access: 27 April 2021.
Valentin, C., Agus, F., Alamban, R., Boosaner, A., Bricquet, J. P., Chaplot, V., de Guzman, T. de Rouw, A., Janeau, J. L., Orange, D., Phachomphonh, K., Phai, D. D., Podwojewski, P., Ribolzi, O., Silvera, N., Subagyono, K., Thiébaux, J. P., Toan, T. D., and Vadari, T.: Runoff and Sediment Losses from 27 Upland Catchments in Southeast Asia: Impact of Rapid Land Use Changes and Conservation Practices, Agriculture, Ecosystems and Environment, 128, 225–238, https://doi.org/10.1016/j.agee.2008.06.004, 2008.
Vanacker, V., von Blanckenburg, F., Govers, G., Molina, A., Poesen, J., Deckers, J., and Kubik, P.: Restoring Dense Vegetation Can Slow Mountain Erosion to near Natural Benchmark Levels, Geology, 35, 303–306, https://doi.org/10.1130/G23109A.1, 2007a.
Vanacker, V., Govers, G., Molina, A., Poesen, J., and Deckers, J.: Spatial variation of suspended sediment concentration in a tropical Andean river system: the Paute River, southern Ecuador, Geomorphology, 87, 53–67, https://doi.org/10.1016/j.geomorph.2006.06.042, 2007b.
Vanacker, V., Bellin, N., Molina, A., and Kubik, P. W.: Erosion regulation as a function of human disturbances to vegetation cover: A conceptual model, Landscape Ecol., 29, 293–309, https://doi.org/10.1007/s10980-013-9956-z, 2014.
Vanacker, V., Guns, M., Clapuyt, F. Balthazar, V., Tenorio, G., and Molina, A.: Distribución Espacio-Temporal de Los Deslizamientos y Erosión Hídrica En Una Cuenca Andina Tropical, Pirineos, 175, 051, https://doi.org/10.3989/pirineos.2020.175001, 2020.
Vanacker, V., Molina, A., and Rosas-Barturen, M.: Data and ancillary data for publication: Natural infrastructure and water erosion mitigation in the Andes, Zenodo [data set], https://doi.org/10.5281/zenodo.6203174, 2022.
Vásquez, A. and Tapia, M.: Cuantificación de la erosión hídrica superficial en las laderas semiáridas de la Sierra Peruana, Revista Ingenieria UC, 18, 42–50, 2011.
Velez, M. I., Salgado, J., Brenner, M., Hooghiemstra, H., Escobar, J., Boom, A., Bird, B., Curtis, J. H., Temoltzin-Loranca, Y., Patiño, L. F., Gonzalez-Arango, C., Metcalfe, S. E., Simpson, G. L., and Velasquez, C.: Novel responses of diatoms in neotropical mountain lakes to indigenous and post-European occupation, Anthropocene, 34, 100294, https://doi.org/10.1016/j.ancene.2021.100294, 2021,
Verstraeten, G., Broothaerts, N., Van Loo, M., Notebaert, B., D'Haen, K., Dusar, B., and De Brue, H.: Variability in Fluvial Geomorphic Response to Anthropogenic Disturbance, Geomorphology, 294, 20–39, https://doi.org/10.1016/j.geomorph.2017.03.027, 2017.
Viechtbauer, W.: Conducting Meta-Analyses in R with the Metafor, J. Stat. Softw., 36, 1–48, 2010.
Wiesmeier, M., Urbanski, L., Hobley, E., Lang, B., von Lützow, M., Marin-Spiotta, E., van Wesemael, B., Rabot, E., Ließ, M., Garcia-Franco, N., Wollschläger, U., Vogel, H. J., and Kögel-Knabner, I.: Soil Organic Carbon Storage as a Key Function of Soils – A Review of Drivers and Indicators at Various Scales, Geoderma, 333, 149–162, https://doi.org/10.1016/j.geoderma.2018.07.026, 2019.
Wunder, S.: Deforestation and the Uses of Wood in the Ecuadorian Andes, Mt. Res. Dev., 16, 367–381, https://doi.org/10.2307/3673987, 1996.
Zimmerer, K. S.: Soil Erosion and Labor Shortages in the Andes with Special Reference to Bolivia, 1953–1991: Implications for “Conservation-with-Development”, World Dev., 21, 1659–1675, https://doi.org/10.1016/0305-750X(93)90100-N, 1993.