the Creative Commons Attribution 4.0 License.
the Creative Commons Attribution 4.0 License.
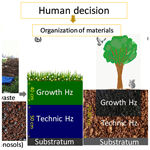
Using constructed soils for green infrastructure – challenges and limitations
Peter M. Groffman
Manuel Blouin
Sara Perl Egendorf
Alan Vergnes
Viacheslav Vasenev
Donna L. Cao
Daniel Walsh
Tatiana Morin
Geoffroy Séré
With the rise in urban population comes a demand for solutions to offset environmental problems caused by urbanization. Green infrastructure (GI) refers to engineered features that provide multiecological functions in urban spaces. Soils are a fundamental component of GI, playing key roles in supporting plant growth, infiltration, and biological activities that contribute to the maintenance of air and water quality. However, urban soils are often physically, chemically, or biologically unsuitable for use in GI features. Constructed Technosols (CTs), consisting of mixtures of organic and mineral waste, are man-made soils designed to meet specific requirements and have great potential for use in GI. This review covers (1) current methods to create CTs adapted for various GI designs and (2) published examples in which CTs have been used in GI. We address the main steps for building CTs, the materials and which formulae should be used to design functional CTs, and the technical constraints of using CTs for applications in parks and square lawns, tree-lined streets, green buffer for storm water management, urban farming, and reclaimed derelict land. The analysis suggests that the composition and structure of CTs should and can be adapted to available wastes and by-products and to future land use and environmental conditions. CTs have a high potential to provide multiple soil functions in diverse situations and to contribute to greening efforts in cities (and beyond) across the world.
- Article
(5496 KB) - Full-text XML
- BibTeX
- EndNote
1.1 Environmental issues in urban areas
Urban areas are exposed to multiple global- (e.g., climate change and biodiversity loss; Czech et al., 2000; McKinney, 2002; Shochat et al., 2006; Zalasiewicz et al., 2008) and local-scale (e.g., urban heat island, increased air pollution, and altered disturbance regimes; Bridgman et al., 1995; Lovett et al., 2000; Rao et al., 2014) environmental changes (Pickett et al., 2011). Urban development decreases forest and agricultural areas (Geneletti et al., 2017), which reduces ecosystem benefits (Foley et al., 2005) due to soil sealing and the alteration of soil properties (Pistocchi et al., 2015; Scalenghe and Marsan, 2009). Urban development fragments landscapes and leads to a loss of connectivity between habitats (Haddad et al., 2015; Madadi et al., 2017; Vergnes et al., 2012). These changes have marked effects on multiple ecosystem services linked to air and water quality (Cariolet et al., 2018; Doni et al., 2018; Jiang et al., 2018; Khan et al., 2018; Latif et al., 2018; Maurer et al., 2019), biodiversity (Guilland et al., 2018; McKinney, 2008; Pouyat et al., 2010), and human health and well-being (Das et al., 2018; Halonen et al., 2015; Säumel et al., 2012).
1.2 Green infrastructures: definition, benefits, and challenges
Green infrastructure has emerged as an important approach to urban environmental issues over the past 20 years. Green infrastructure is defined as engineered environmental design features built in interconnected natural and urban spaces to provide multiple ecological functions (Maes et al., 2015). Green infrastructure features can be completely man-made, such as green roofs and green walls, or can be based on an existing ecosystem with some intervention, such as parks, urban farms, and bioswales. Ecological functions associated with green infrastructure include habitat for biodiversity (Vergnes et al., 2012), improving air and water quality (Pugh et al., 2012), reducing storm water flows into sanitary sewer networks (Lucas and Sample, 2015), filtering noise, microclimate stabilization (O'Neill et al., 2018), food supply (Specht et al., 2014), improving psychological and physiological human health (Tzoulas et al., 2007), and strengthening social relationships by attracting people to outdoor spaces (Coley et al., 1997). Economic advantages include increased tourism, reduced energy use for indoor temperature regulation, lower wastewater treatment costs, and increased property values and tax revenue (Mell et al., 2016).
Due to its ability to supply a wide range of ecosystem benefits (Flores et al., 1998; Kazemi et al., 2011; Keeley et al., 2013; Lee and Maheswaran, 2011; Opdam et al., 2006; Sandström, 2002), green infrastructure has attracted significant attention in both developed (Brunner and Cozens, 2013; European Environment Agency, 2017; Davis et al., 2009; Hall, 2010; Kabisch and Haase, 2013; Lewis, 2005; Pauleit et al., 2005) and developing (Akmar et al., 2011; Byomkesh et al., 2012; Jim, 2005; Qureshi et al., 2010; Rafiee et al., 2009) countries and is now an important component of city planning around the world (Tan et al., 2013; Zhao et al., 2013; Zhou and Wang, 2011). Interest in the use of green infrastructure is likely to increase along with the ongoing expansion of cities.
There are numerous challenges associated with the development of green infrastructure (Haaland and van den Bosch, 2015; Jim, 1998). Building green infrastructure in densifying cities may pose challenges as there may be a conflict of interest among officials with regard to using the land for green spaces as opposed to residential, commercial, or service uses (Haaland and van den Bosch, 2015). Building a new green space requires a large of amount of financial and natural resources such as human labor, natural topsoils, and other imported materials. These costs are challenging because there is no easily measurable value for the economic benefits of green infrastructure (Haaland and van den Bosch, 2015).
Green infrastructures must provide suitable environmental conditions to foster the growth of vegetation. There is a need for sufficient sunlight and high-quality water for plants to grow, low foot traffic to avoid soil compaction, and an adequate surface area for tree growth. There is a potential for the high mortality of plants due to suboptimal levels of water, light, and nutrients, contamination, compaction, and other urban stressors.
1.3 Need for functional soils in urban areas
Soils in urban areas, especially nonsealed ones, are highly heterogeneous, varying from natural (e.g., relict forest soils) to fully artificial (De Kimpe and Morel, 2000; Huot et al., 2017). Many urban soils are derived from a combination of exogenous, anthropogenic (so-called “technic” materials), and natural geologic parent materials (Lehman, 2006). Urban soils have altered disturbance regimes from human activities (Godefroid and Koedam, 2007) and are frequently exposed to high volumes of storm water surface runoff (McGrane, 2016). As a consequence, urban soils frequently exhibit poor biological, physical, and chemical conditions that directly affect plant growth and reduce their ability to provide multiple ecological functions (De Kimpe and Morel, 2000; Morel et al., 2014).
Soils are a fundamental component of green infrastructure, playing key roles in supporting plant growth, infiltration of runoff, and microbial activities relevant to nutrient cycling and pollutant degradation (Deeb et al., 2018; Keeley et al., 2013). To ensure the development of vegetation and offset the low fertility of urban soils, large quantities of topsoil are imported from surrounding agricultural or forest areas (e.g., 3 million of m3 yr−1 in France; Rokia et al., 2014). Taking soil from rural areas for urban use is not sustainable as it is costly and is accompanied by environmental risks, e.g., release of carbon dioxide (Walsh et al., 2018, 2019). Soil is a complex ecosystem and a limited resource that forms slowly, e.g., the rate of soil production has been estimated to be between 20–100 m My−1 (i.e., 2–10 mm per century; Heimsath et al., 1997). Thus, soil cannot be considered to be a readily renewable resource that can be excavated and easily transported from rural to urban areas (Walsh et al., 2019). These concerns have stimulated interest in the development and use of constructed soils for multiple urban uses.
1.4 Existing solutions for providing functional soils in cities
Solutions to the challenges of greening cities depend on the degree and type of soil degradation, the affected area, and the size of the project. The most widely applied solution is the complete removal of the topsoil and replacement with natural, arable soil imported from nonurban areas (Dick et al., 2006; Dickinson, 2000). As noted above, it is expensive to transport soil, and the removal of the old soil creates a problematic waste product. This solution is particularly difficult to implement in developing countries (Bradshaw, 1997; Hüttl and Bradshaw, 2000).
Another, less common, solution to urban soil problems that has been applied in specific cases on smaller scales is using organic amendments present in the city, such as composts, sludge, industrial by-products, and biosolids, to improve topsoil characteristics (Larney and Angers, 2012). Adding organic wastes to restore the ecosystem functions has received great attention in different scientific studies (Basta et al., 2016; Gómez-Sagasti et al., 2018; Kumar et al., 1985; McGeehan, 2012). Moreover, inexpensive sources of waste and by-products have become increasingly available in recent years, allowing for the improvement of soil quality while reducing landfill material (Vetterlein and Hüttl, 1999). The main drawback of this solution is concern about the transfer of pollutants, especially Pb, from wastes to groundwater and runoff and subsequently to organisms (Battaglia et al., 2007; Calace et al., 2005). Few studies have addressed the long-term sustainability of reclamation by organic waste (Bacholle et al., 2006; Nemati et al., 2000; Vetterlein and Hüttl, 1999; Villar et al., 1997). Key concerns in this regard are that composting is not widely practiced or integrated in policies worldwide, and the amount of available organic waste varies widely within and between cities (Narkhede et al., 2010).
Adding phosphate-bearing amendments such as chemical fertilizers or fish bone waste has been found to stabilize lead in urban agriculture (Ruby et al., 1994). Phosphate amendments bond to unstable lead and form insoluble pyromorphite minerals (Ma et al., 1995; Paltseva et al., 2018a, b). The long-term stability of these minerals is uncertain, however, as they can change with soil chemical conditions. Moreover, adding phosphate can mobilize arsenic through competitive anion exchange (Creger and Peryea, 1994; Paltseva et al., 2018a). In addition, chemical fertilizers can have a variety of negative impacts on air and water quality in the surrounding environment.
Using native instead of nonnative plants has been proposed as a reclamation solution in urban landscapes (Ries et al., 2001) for storm water management (Bartens et al., 2008; Culbertson and Hutchinson, 2004; Lucas and Greenway, 2007; Selbig and Balster, 2010), land use sustainability (Dornbush, 2004; O'Dell et al., 2007), conservation of wildlife habitat (Fletcher and Koford, 2002), erosion control (Beyers, 2004), carbon sequestration (Isaacs et al., 2009), and soil remediation (Swedo et al., 2008) and stabilization. Planting native species has an indirect influence on soils by promoting biodiversity and creating resilient communities. However, there is no guarantee that native plants will grow in soils with significant anthropogenic impacts (Suding et al., 2004). Moreover, this solution has been practiced mostly to restore natural landscapes and to reverse species loss (Richardson et al., 2007).
1.5 Opportunities to construct new soils
The “new” idea of building constructed Technosols is based on combining the biotic and abiotic characteristics of diverse materials to produce specific functions and benefits (Fig. 1). Technosols are defined as deliberate mixtures of organic and mineral wastes and by-products constructed to meet specific requirements (Craul, 1999; Damas and Coulon, 2016; Rokia et al., 2014; Séré et al., 2008; Yilmaz et al., 2018). The process of constructing Technosols includes existing approaches such as recycling organic materials or including native plants. The use of easily available materials provides economic benefits (Walsh et al., 2019), has limited impact on natural resources, and makes use of waste materials. Constructed Technosols can be created as new buildings are constructed from excavation and demolition waste, saving on labor and transportation costs for both waste disposal and the importation of soils. Because the overall cost of constructed soils is lower, green spaces built with constructed Technosols can be more easily integrated with city planning. These advantages can be particularly important in cities or neighborhoods with fiscal constraints, thus alleviating some environmental justice issues.
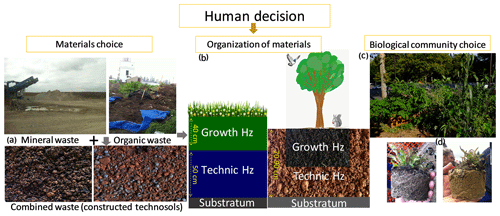
Figure 1Diagram presents abiotic and biotic components of constructed Technosols. (a) Example of waste mixture during Maha Deeb's Master's thesis. (b) Mixture organized in different layers, depending on the land use (SITERRE project). (c) Constructed Technosols for urban farming (carbon sponge project). (d) Constructed Technosols built by the youth club for research and development in the Lycée du Paysage et de l’Environnement (High School of Landscape and Environment) in Vaujours, France. Note: Hz – horizon.
In the sections below, we review (1) current methods for creating constructed Technosols (CTs) adapted for various green infrastructure designs and (2) published examples in which CTs have been used in green infrastructure.
2.1 What is a constructed Technosol?
Anthropization is the process of soil formation in urban environments. The World Reference Base for Soil Resources (IUSS, 2015) defines Technosols as soils which contain greater than 20 % artificial materials by volume and are within the first 100 cm of the land surface. At the moment there is no universal definition of constructed Technosols. Séré et al. (2008) defined the construction of a Technosol as a process “using wastes and industrial by-products which are formulated and stacked in layers to build a new soil profile over in situ degraded substrates”. Prokofyeva et al. (2011), on the other hand, define constructed Technosols (“constructozems”) as bodies of soil with complicated stratification, greater than 40–50 cm in thickness, that are created for special purposes such as salt protection in semi- and severely dry areas (Smagin, 2012). In this review, we will define constructed Technosols as the result of the voluntary action of creating a “soil” (soil made by humans) using artefacts (i.e., technogenic materials, particular wastes, or seminatural materials including deep materials such as sediments or soil material from C horizons) and intentionally shaping them to provide a suitable environment for vegetation growth. The choice of the vegetation may be for aesthetic (greenery), protective (wind erosion and storm water), or productive (agriculture) roles (Fig. 1). This new soil could be created for use in multiple green infrastructure designs including squares and parks, accompaniments for public buildings (tree-lined streets, flower beds, verges, pocket spaces, and green roofs), accompaniments for traffic lanes (roads and railway lines), storm water management, urban farming, household yards, and abandoned land reclamation (Fig. 2).
2.2 Bibliographic search
Structured and semistructured searches were conducted using major scientific databases (namely, Scopus, Web of Knowledge, and Google Scholar) along with appropriate cross-referencing to obtain supporting literature. Additional searches were based on the authors' own knowledge of potentially relevant work and their experience with constructed Technosols. Structured searches were carried out with the following keywords (title, abstract, and keywords): constructed Technosols, engineered soils, Anthroposols, green space, parks, green infrastructure, green roof, storm water management, street trees, ecosystem services, densification, abandoned land, food security, soil functions, biodiversity, urban farming, recycling, landfill, and waste management.
The literature considered for the review was published in English, French, and Russian. Other texts that were difficult to translate or locate were excluded from this review.
A total of five land uses were chosen for this review based on the land use classification and evaluation provided by Panduro et al. (2013) that included the following eight land uses: parks, common area apartments, common area houses, sports fields, agriculture fields, green buffers, nature, and lakes. We merged the first three land uses into a single group, namely “parks and squares with lawns”, assuming that these would create similar conditions for the use of constructed Technosols. Sports fields were not included as they have already been discussed in the literature (Puhalla et al., 1999). Nature and lakes were excluded as the application of constructed Technosols is not needed in these land uses. Degraded land and tree-lined streets were added as complementary independent categories because they are commonly present in urban areas.
3.1 Construction of Technosols for different land uses in green infrastructure
3.1.1 Characteristics needed to fulfill green infrastructure requirements
Green infrastructure features require functional soils or adapted substrates in order to be fully operational. Characteristics that should be considered for the construction of Technosols include the following:
-
Adapted chemical fertility (Rokia et al., 2014) suitable for specific vegetation with specific features (e.g., trees, lawns, grasslands, and flower beds) is needed;
-
Sufficient soil depth for vegetation anchorage (Marié and Rossignol, 1997), e.g., trees with deep roots and high root system density, is required;
-
Compatible concentrations of contaminants related to the actual use of the public spaces (Egendorf et al., 2018) in terms of health risks (e.g., inhalation and soil ingestion) and land use (e.g., urban agriculture) are needed;
-
Compatible bearing capacity for trampling, parking, or vehicle traffic (Grabosky and Basset, 1998) is needed;
-
Permeable soils are required for water infiltration in order to limit floods (Liu et al., 2014);
-
Sufficient water storage capacity to support green lawns and ornamental plants with limited irrigation is needed (Smagin and Sadovnikova, 2015; Vasenev et al., 2017);
-
Low bulk density and shallow root system depth (Vijayaraghavan, 2016) are required for specific substrates that are used on buildings (e.g., green roof);
-
Minimal long-term maintenance, e.g., the addition of organic matter and frequent watering, is not necessary;
-
Stable soil structure is often desired to limit erosion and dust respiration (Deeb et al., 2017);
-
High hydraulic conductivity is required for land uses such as green roofs and storm water management systems;
-
Moderate ratios of mineral and organic content, generally refraining from the addition of more than 30 % organic matter is desired (Deeb, 2016a; Grosbellet, 2008; Vijayaraghavan, 2016) to avoid excessive loss of organic matter by oxidation (CO2 emissions) or leaching, which will change soil volume.
These characteristics vary depending on the intended uses and functions of the green infrastructure in question (Fig. 2; Table 1). For example, (i) tree-lined streets should have stronger physical support functions than green roofs, (ii) food production in community gardens requires parent materials with very low contamination, and (iii) organic contaminant decomposition and water infiltration are important for bioswales (green infrastructure for storm water management).
3.1.2 Use of waste materials
The generation of waste is a negative anthropogenic impact – especially in urban areas, which are estimated to produce 2.2 billion tons of solid waste per year by 2025 with a cost of USD 375.5 billion (Wilson and Velis, 2015). Efforts to minimize solid waste disposal in landfills and to encourage recycling are increasingly common (Krook et al., 2012). Constructed Technosols are a viable solution for waste management.
There is a huge diversity of wastes and by-products generated in cities. However, not all of them are considered relevant for the construction of Technosols (Table 2). Some researchers (Deeb et al., 2016a, b, 2017; Pruvost et al., 2018; Walsh et al., 2018, 2019) have focused on construction and demolition waste (CandD) mixed with organic waste because CandD debris generate high percentages (around 35 %) of the total solid waste worldwide (Hendriks and Pietersen, 2000). In Europe, CandD debris comprise 30 % of total waste (European Commission, 2020). Walsh et al. (2019) showed that an estimated 1.7×106 t of unused, uncontaminated native soil, consisting mainly of glacial sediments, is generated each year from building construction in New York City alone. Disposal of this clean material requires 60 000 truck transports and 8.7×106 km of travel distance, using 4.3×106 L of petroleum products, emitting 11 800 t of CO2, and costing over USD 60 million per year. Despite the large volumes of CandD waste produced, only small fractions are generally recycled (Gálvez-Martos et al., 2018).
Table 2List of wastes previously used for constructed Technosols in the literature and their main characteristics.
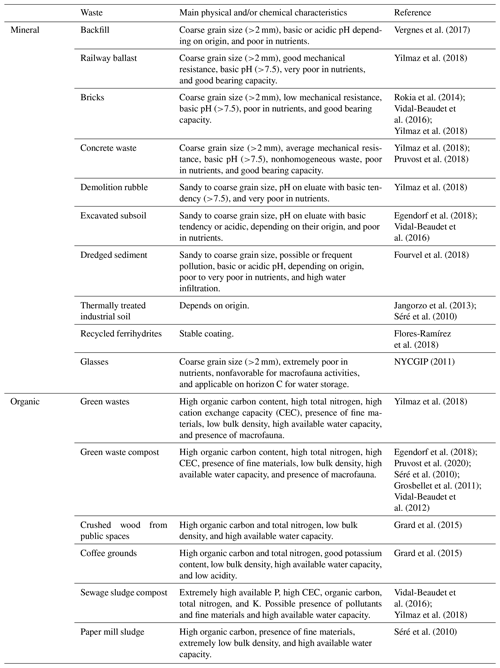
The SITERRE project (Damas and Coulon, 2016), one of the first and largest projects to create constructed Technosols for greening urban spaces, was based on the European catalogue of 836 urban waste types. The following three specific criteria were used to choose wastes for use in constructed Technosols:
-
Nontoxic: the waste should not be classified as dangerous for human health or living organisms.
-
Easy to handle: liquid and pasty wastes were not considered, and only granular solid materials could be used.
-
Suitable: the selected wastes have to generate minimal disturbances to the local population and also be compatible with the lifestyle in densely populated urban areas in terms of their appearance, i.e., organoleptic criteria such as smell or color.
After satisfying the fundamental criteria above, the selected waste should additionally comply with at least one of the following:
-
Fertility: the materials should contribute positively to the germination or development of vegetation.
-
Capacity: the materials should have a positive impact in terms of geotechnical properties, i.e., soil stability, plasticity, and cohesion.
Based on these five criteria, five mineral and six organic by-products were chosen (Damas and Coulon, 2016), including (i) materials from excavated acidic soil deep horizons, (ii) materials from excavated basic soil deep horizons, (iii) bricks, (iv) concrete, and unsorted demolition rubble, (v) track ballast (the material upon which railroad ties are laid), (vi) green wastes, (vii) sewage sludge, (viii) green waste compost, (ix) sewage sludge compost, (x) paper mill sludge, and (xi) street-sweeping wastes. Other researchers have investigated different waste materials including coffee grounds (Grard et al., 2015), backfill waste (Vergnes et al., 2017), thermally treated industrial soil (Séré et al., 2010), recycled ferrihydrites (Flores-Ramírez et al., 2018), and glass (NYCGIP, 2011).
A specific formula and/or mixture of waste ingredients must be determined to achieve the expected functions. A total of 25 combinations were made by Rokia et al. (2014) using the wastes selected in the SITERRE project, which were either binary mixtures at 5 different ratios (0∕100, 20∕80, 50∕50, 80∕20, and 100∕0; volume/volume) or ternary mixtures with 60 % v∕v coarse mineral material and 40 % v∕v mixtures of organic and mineral mixtures. While no single-stream waste material was adequate as a soil or horticultural substrate in isolation, the mixtures produced a range of chemical and physical properties. Both brick waste and excavated deep horizons mixed with compost produced physically and chemically fertile substrates, based on the chemical and physical characteristics of fertile soils – but not on their ability to sustain plant growth (Rokia et al., 2014). Mathematical models were developed to simulate the characteristics of mixtures from the characteristics of single materials (Rokia et al., 2014).
3.1.3 Technical constraints
There are constraints that need to be considered when planning to use constructed Technosols for developing green infrastructure. Some waste materials are extremely heterogeneous and thus difficult to characterize to ensure the safety of the environment and public health. In addition, different materials offer a wide range of physicochemical properties. For example, even though brick and organic matter showed optimal chemical characteristics for plant growth in the SITERRE project (Rokia et al., 2014), these materials showed low potential for aggregation compared to excavated deep horizon material combined with organic matter (Vidal-Beaudet et al., 2016). Fourvel et al. (2018) compared six types of degraded excavated sediments with heterogeneous characteristics from France and found that biomass production varied greatly among the sediment types.
Mixed waste materials need a specific time period to form a stable structure; thus, erosion, runoff, and compaction are risks in the early stages of soil formation. The establishment of plants with high-density root systems (Deeb et al., 2017) or building erosion-control barriers around the constructed Technosols could reduce these risks.
Fresh organic waste can be problematic as it can have a toxic effect on plant growth (Yilmaz et al., 2016), sometimes by creating anoxic conditions. Thoughtful choices, e.g., using mature compost or mixing in mineral material that drains well (such as sand) can avoid anoxic conditions. Even with these choices, the addition of organic material must be specific to its intended land use as amending organic matter on a regular basis may lead to the accumulation of heavy metals over time. To mitigate this limitation, additional organic matter should be avoided over time to improve Technosol quality and maintain the integrity of established soils. If organic waste must be added, organic matter with heavy metal contamination and pollutants may be mixed with other nontoxic waste ingredients in calculated proportions to lower the overall concentration into acceptable ranges. These ranges will vary according to the land use and local regulations. For example, total petroleum hydrocarbons should not exceed 5000 mg kg−1, as defined in the European Union (Pinedo et al., 2013). In New York City, heavy metals and organic contamination limits strictly depend on land use (NY-CRR, 2017).
Microplastics are another source of contamination that should be considered when building Technosols. Although the number of current studies is limited, plastic contamination may negatively affect plant growth, soil organisms, and human health through integration in the food chain (Horton et al., 2017). Studies show that the use of sewage sludge compost as fertilizer increases microplastic contamination in soils (Corradini et al., 2019). To prevent microplastic contamination, sewage sludge compost should be avoided in high quantities, should only be used in low ratios, and should be tested for microplastic contamination before application.
Finally, one of the most important limits is social rejection, especially since some organic waste (e.g., sewage) can have an objectionable odor. This problem can be avoided by applying such materials in deeper horizons. However, finding a solution for the general unpopularity of using waste for developing green spaces could be more challenging as it requires specific education, observation over time, cooperation between biophysical and social science disciplines, and active engagement with communities. It can be expected that the increasing reuse of materials considered to be waste will influence norms and policies regulating their production in a way which could ease their recycling in the future.
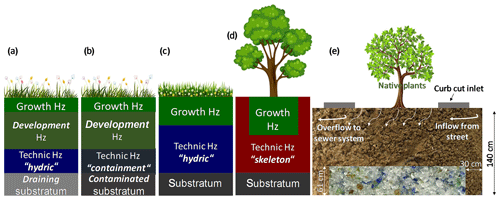
Figure 3Examples of the organization of horizons in constructed Technosols noted in the literature for (a–b) extensive grassland (Biotechnosol project), (c) parks and square lawns, (d) tree-lined streets (c and d from the SITERRE project), and (e) the storm water management system (DEPNY, 2010). Note: Hz – horizon.
3.1.4 Pedogenesis
The process of pedogenesis (soil formation), which is strongly controlled by local environmental conditions, dictates that the age of the Technosols is a key driver of their properties (Hui et al., 2017). Séré et al. (2010) demonstrated that artificial soils underwent pedogenic processes similar to natural soils (e.g., chemical weathering, soil structure evolution, and horizonation) and Deeb et al. (2016a) showed that after one cycle of humidification (1 week in laboratory conditions), constructed Technosols were able to develop hydrostructural behaviors similar to natural soils. After 5 months, the same Technosols showed a high ability to aggregate and, in some cases, enrich the carbon content of particular size fraction of aggregates (Deeb et al., 2017). High mineralization of organic matter may occur in the early stages of the pedogenesis of constructed Technosols, especially when a high quantity of organic matter is used (Grosbellet, 2008). Because the loss of organic matter will vary with environmental conditions, modifying the design of a constructed Technosol could be a solution. For example, in arid climates where the risk of hydromorphy is low, one can add a higher concentration of organic matter in subsurface deep horizons than in surface horizons so that organic matter will be protected from oxidation and will improve the water availability surrounding the root system. Establishing an active plant community as an internal source of organic matter and as a driver of multiple biotic and abiotic interactions is also important (Deeb et al., 2016b; Jangorzo et al., 2018, 2014; Pey et al., 2013a, 2014). A 3-year monitoring plan of carbon balance in Technosols from Moscow, Russia, showed substantial carbon release during the first months after construction, which was almost completely fixated in the next 3 years by carbon input from the root biomass growth (Shchepeleva et al., 2017, 2019). Given the specific growth requirements of different species, plant selection must be done with great care (Pruvost et al., 2018).
3.2 Construction of Technosols for specific land uses in green infrastructure
3.2.1 Parks and square lawns
The majority of studies of implementing parks or squares attempt to mimic natural soils by constructing Technosols with one or two distinct horizons. Horizon A (growing horizon) is generally designed to support germination and the initial growth of grasses; as a result, high amounts of organic wastes are often used as dominant ingredients. Horizon B (technical horizon) is commonly designed to provide high storm water retention and to prevent the leaching of organic carbon and nitrate (Fig. 3a).
Deeb et al. (2016a, b, c, 2017) defined the optimal waste mixtures to fulfill specific soil functions such as erosion control, carbon and water storage, structural stability, and biomass production for the growing horizon. These authors built constructed Technosols under specific climate conditions and mixed different amounts of compost (0 %, 10 %, 20 %, 30 %, 40 %, and 50 %; v∕v) with demolition waste to optimize the production of a grass, namely Lolium perenne. Depending on the desired function of the soils, different ratios of compost will complement certain soil functions more than others. For example, biomass production generally increases with increasing compost ratios. Structural stability was also found to increase with compost ratios but only in the presence of plants and macrofauna, the main drivers of soil structure. In terms of hydrostructural properties, the mixtures ranging from 20 % to 40 % compost exhibited similar characteristics. Therefore, it may be unnecessary to use excessive amounts of compost to achieve optimal available water content in macro- and micropores. The interaction between parent materials (compost ratio) and biota played a major role in water storage and available water, explaining 39 % of the observed variance of these functions.
Other organic parent materials, such as uncomposted green waste (tree and grass cuttings) or urban sewage sludge, have also been evaluated. Yilmaz et al. (2018) studied four constructed Technosols for the development of parks, squares, lawns, and trees in in situ lysimeters. The artificial soils were either planted with trees (Acer platanoides) or ryegrass (Lolium perenne L.). The authors studied specific soil functions such as soil structure arrangement and water maintenance through analyzing hydraulic conductivity, macro–microporosity, organic matter content, and water availability. The results demonstrated that, compared to natural soils, constructed Technosols provided high porosity, abundant water storage for plant use, and a high production of trees and ryegrass, likely due to the high organic matter content and soil pH of the Technosols. The study concluded that constructed Technosols have the capability to support vegetation growth for urban greening.
Our analysis of the results from previous studies supports the recommendation of using a soil mixture with 20 % to 30 % compost for parks and lawns to avoid mineralization and nutrient leaching. In addition, because macrofauna and macroflora have a positive effect on structural stability, porosity, and carbon storage, it is recommended to integrate biota, e.g., earthworms, as soon as possible when planning to build parks with constructed Technosols (Deeb et al., 2016c, 2017). Several ways exist for integrating biota; thus, one should conserve macrofauna in the rare cases where it is already present in materials. When absent, one could also favor its recolonization from populated areas by planning ecological corridors or by inoculation with soil blocks or ex situ bred populations (Blouin et al., 2013). Vergnes et al. (2017) surveyed the habitat colonization of parks built using a mixture of excavated deep horizons and natural topsoils and noted that parks that had an addition of topsoil had significantly more earthworms and ants compared to parks without the topsoil addition.
Pruvost et al. (2018), in a 4-year field experiment, tested the potential of excavated soil deep horizons alone or mixed with green waste compost and/or concrete waste to support the growth of six tree species for the development of parks. To assess the fertility of the recycled substrates, they measured tree growth, soil physicochemical characteristics, and soil macrofauna. Excavated deep horizons, independently and with other mixtures, showed promising results. The mixture with the highest tree mortality was excavated deep horizon and green waste compost. However, the addition of crushed concrete to this mixture counteracted the negative effects and decreased tree mortality. The mixture with 10 % green waste compost and 70 % crushed concrete had the most favorable outcome, with the highest tree survival, growth rate, and fastest soil macrofauna colonization. Acer campestre and Prunus avium were the tree species in the parks with no mortality and are likely the most adaptable. These results highlight the importance of balancing both the soil mixture and species choice (Fig. 1).
3.2.2 Tree-lined streets
Urban street trees frequently exhibit high mortality due to multiple factors (Jim, 1998; Rossignol, 1999) including limited nutrients (Li et al., 2013), contamination (Muir and McCune, 1988), poor soil physical properties (Lindsey and Bassuk, 1992), inadequate light (Jim, 1998), and insufficient space for roots (Dubik et al., 1990; Lindsey and Bassuk, 1992). Additional literature has documented a conflict between street trees and urban infrastructure (sidewalks, sewers, electricity cables, etc.; Lindsey and Bassuk, 1992). To avoid these conflicts, recommendations have been developed for suitable tree species (McPherson et al., 2016), their spatial distribution (Thomsen et al., 2016), and for providing sufficient surface area for root systems. Moreover, several researchers have documented how engineered (constructed) soils can reduce tree morality and conflicts with infrastructure (Rossignol, 1999). The main idea of these recommendations is to limit soil compaction and maximize water storage capacity and to avoid tree species that have a short life cycle and that damage surrounding infrastructure. For example, Daunay (1999) used horizons of different mixtures of natural and artificial materials including a 100–150 cm layer of 65 % ground stone and 35 % soil mixture, overlain by 30–50 cm of organic material. The ground stone mixture provided a firm foundation for plants with a low risk of compaction, creating a resilient soil structure with high infiltration capacity and no inhibition of root growth.
Damas and Coulon (2016) developed and tested a model soil for street trees (Fig. 3b) that includes a base layer skeleton horizon composed of a mixture of coarse mineral parent materials (i.e., track ballast, concrete waste, and demolition rubble). with a low content of organic materials, topped with a growing horizon layer of organic-rich materials for root growth. Cannavo et al. (2018) adopted a similar horizon order, using a layer of sand (0.15 m) as a base foundation for adequate drainage. For the skeleton horizon (1.85 m), they used three different mixtures. One contained fine mineral material, demolition waste, and green waste; the second consisted of fine mineral material track ballast, and sewage sludge; and the third used chalcedony and leaf litter. These were covered by a growing horizon (0.8 m) comprised of 60 % crushed brick waste and 40 % sewage sludge and green waste. After 3 years, the two skeleton horizon mixtures that contained fine mineral, demolition waste, track ballast, green waste, and sewage sludge showed greater plant development than the mixture with chalcedony and leaf litter alone (Cannavo et al., 2018), suggesting that the skeleton horizons are an important source of nutrients for plant growth. Other studies have documented the importance of the growing horizon, specifically the richness of the organic waste (Vidal-Beaudet et al., 2015) for tree rhizosphere development. While these constructed Technosols showed encouraging results for the growth and development of street-lining trees, it is important to note that these results were the outcome of innovative design where each detail, such as the waste mixture, order of layers, depth, tree species, etc., was meticulously considered as the soil was engineered.
3.2.3 Green buffers for storm water management
The soils in storm water management systems face similar, if not greater, anthropogenic stress than other urban soils. Storm water management systems are designed to filter storm water that contains contaminants, with small areas receiving runoff from much larger areas of impervious surfaces, creating physical, chemical, and biological stress for soils and plants. The main objective of storm water management systems is to absorb runoff and avoid floods by improving water entry into the soil profile and by enhancing water storage within the soil, which increases water retention for vegetation use. A range of biogeochemical processes affect greenhouse gas emissions, organic carbon storage, and the biofiltering of heavy metals and organic contaminants in these features (Deeb et al., 2018; McPhillips and Walter, 2015; McPhillips et al., 2018).
Given the high-stress conditions of storm water management systems, there is great potential for the use of constructed Technosols in these features to optimize water storage and infiltration by providing high porosity, permeable surfaces, and supporting microbial activity by planting vegetation with root systems that are resilient against physicochemical pressures. Constructed Technosols have been used in several green infrastructure designs for storm water management built by the Department of Environmental Protection in New York City (NYDEP, 2010), including enhanced tree pits (ETPs) and street-side infiltration swales (SSISs). Both ETPs and SSISs are rectangular-shaped bioswales built on sidewalks adjacent to the street. ETPs typically cover an area of 9 m2, with a 0.6 m constructed soil layer underlain by different depths of gravel, recycled glass, or storage chambers (Fig. 3c). SSISs have an average area of 19 m2 but do not have layers of gravel, recycled glass, or storage chambers. Water from the street enters the ETPs and SSISs through curb cuts and inlets into the storm water management system (NYCGIP, 2011). The design (surface area, order of layers, distance to soil from street, and shape) of the storm water management system affects the bacterial community and function (Joyner et al., 2019). Significant levels of denitrification (a water quality maintenance process that converts nitrate, an important agent of eutrophication, into nitrogen gas) have been observed in these green infrastructure systems (Deeb et al., 2018) and at other sites as well (Bettez and Groffman, 2012; Morse et al., 2017). Other studies have found high levels of microbial diversity and activity (Gill et al., 2017) and improved infiltration rates in these systems relative to urban soils (Alizadehtazi et al., 2016). The constructed storm water management soils studied by Deeb et al. (2018) had low levels of contamination by metals and total petroleum hydrocarbons. These results suggest that there is a high potential for the use of constructed Technosols in storm water systems. although further research, in a wider variety of settings (e.g., higher contaminant levels), is needed.
3.2.4 Urban farming
There is a significant body of work examining the possibilities and challenges of urban farming, but most research on this topic does not necessarily examine the soil that literally lays the ground for such endeavors. The most common attention given to urban agriculture soils pertains to the presence of inorganic and organic contaminants (Brown et al., 2016; Kessler, 2013; Marquez-Bravo et al., 2016; McBride et al., 2014; Mitchell et al., 2014; Sipter et al., 2008; Spliethoff et al., 2016). Both ongoing and historic activities related to industrial processes, the use of leaded paint and gasoline, and incineration have left a legacy of potentially toxic elements and compounds in urban soils that create risks for gardeners and others who come into contact with the soil (Chillrud et al., 1999; Laidlaw et al., 2017; Mielke et al., 1983; Root, 2015). While there are cases in which urban farmers use soils formed from naturally deposited parent materials, most proactive urban farmers deliberately avoid such practices in order to mitigate contaminant exposure and enhance fertility. Most urban farmers grow plants in a wide range of constructed Technosols that are difficult to classify (i.e., raised beds built by composting local organic waste mixed with potting soil). These are valuable safety practices given the research showing that community growing spaces are less contaminated than home gardens or yards (Cheng et al., 2015). These findings may be due to a variety of factors, including the use of imported soil materials and amendments that dilute or immobilize pollutants.
Researchers have examined the potential for constructed Technosols to support nonedible biomass (Rodrigues et al., 2019). Rokia et al. (2014) characterized the agronomic physical and chemical properties of a range of constructed Technosols built by using a combination of waste materials. However, these authors did not evaluate biological properties, especially yield.
Very few studies have performed field-scale trials and analyses of constructed Technosols in urban agriculture settings. Brandon and Price (2007) investigated the use of sediments dredged from rivers and lakes and outlined an approach for manufacturing soil. Such dredged sediments have been found to be appropriate for agricultural purposes (Darmody and Marlin, 2002). Similarly, Egendorf et al. (2018) used excavated deep horizon sediments from the New York City Clean Soil Bank (CSB) PUREsoil NYC program (Walsh et al., 2019) mixed with different percentages of compost (20 %, 33 %, and 50 %) to create constructed Technosols. These constructed Technosols were used for field-scale trials in urban farm settings and were found to be successful for urban agriculture (Egendorf et al., 2018). In these studies, the constructed Technosols exhibited low levels of contamination, which did not increase over the span of 1 year of exposure to the urban atmosphere. The results from these authors suggest that there is high potential for constructed Technosols to be used in urban agriculture.
Grard et al. (2018) highlighted the importance of constructed Technosol design (e.g., the order of substrate layers to specific biological activities; Fig. 4a) in green roofs. This author studied multiple plots filled with various urban wastes such as green waste compost, shredded wood, crushed tiles and bricks, used coffee grounds, and a biowaste compost. The different Technosols were evaluated in terms of food production, fertility, and water retention. Results showed that the constructed Technosols exhibited low contamination levels, were fertile, and could sustain high-quality food production for up to 5 years.
As mentioned above, urban farmers and growers are often constructing their own Technosols when they import a variety of substrates as growing media. Similar to natural soils, applying crop rotation to constructed Technosols may improve the physicochemical characteristics, stability, and crop production. In addition, choosing a variety of species with different root systems may improve soil stability and nutrient absorption. Community-based participatory research projects could help in collecting data about the effects of amendments and other urban farmer practices on ecosystem services.
3.2.5 Reclaimed derelict land
A major focus of urban sustainability programs has been brownfield sites and other types of degraded land. By definition, a brownfield site is a contaminated or degraded plot of land that formerly served as an industrial or commercial facility but is no longer in use or operational (US EPA, 1997). Revitalizing brownfield sites, which are commonly located in low-income neighborhoods, may not only beautify these neighborhoods but may also bring about economic opportunities that arise from developing commercial, industrial, or residential properties in place of the brownfield. Aside from economic opportunities, brownfield sites can also be recycled into green spaces or green infrastructures that will potentially introduce a wide variety of social, environmental, and health benefits. Although greening brownfields can potentially produce numerous benefits, they are challenging to develop due to safety and liability concerns and high planning, construction, and maintenance costs (De Sousa, 2003).
Soils are fundamental to brownfield redevelopment, and there is a high potential for the use of constructed Technosols in brownfield projects. For example, Séré et al. (2008) highlighted the importance of shaping a functional soil for restoring abandoned land by focusing on three main soil functions, namely water buffering and transformation, biomass production, and trace element cycles. These authors noted additional functions such as microbial activity related to the nitrogen cycle (Hafeez et al., 2012a, b), improving soil structure (Jangorzo et al., 2018, 2013, 2014), and macrofauna activity related to nutrient cycling (Pey et al., 2013a, 2014). The French Scientific Interest Group (GISFI; http://gisfi.univ-lorraine.fr/fr/, last access: 4 September 2020) conducted the first large-scale (100 m3) field experiment using waste comparing two types of constructed Technosols for the ecological reclamation of abandoned land (Fig. 4b) based on varying compositions and the depths of three different materials, namely green waste compost, paper by-products, and treated industrial sediment wastes (Séré et al., 2008). These constructed Technosols provided several soil functions, such as water filtration for removing contaminants and supplying nutrients to surrounding plants (Séré et al., 2008). Over time, they developed functional organo-mineral soil pedons (Séré et al., 2008) with active pedogenic process (Séré et al., 2010) and a wide range of biotic activities (Hafeez et al., 2012a; Pey et al., 2013b). These authors suggest that constructed Technosols have an important role to play in brownfield reclamation, but they make several recommendations that should be considered, such as the choice of waste, especially in the early stages (Séré, 2007; Séré et al., 2008), the importance of stimulating pedogenic process (Séré et al., 2008), and careful consideration of the targeted land use functions (Séré et al., 2008). Slukovskaya et al. (2019) demonstrated the advantages of Technosol construction for reclaiming heavily contaminated lands in the Russian subarctic zone. The implementation of carbonatite and serpentinite–magnesite wastes covered by hydroponic vermiculite not only allowed them to immobilize considerable amounts of Ni and Cu but also increased biomass production, carbon sequestration, and microbiological activity.
This review provides evidence that the construction of Technosols for the design of urban green infrastructure is a valuable alternative solution to the consumption of natural resources such as soil materials, wood chips, or peat coming from surrounding rural areas. Constructed Technosols can contribute to sustainable environments in urban contexts as they supply multiple functions and services in several land uses. Over the past 10 years, studies have confirmed the value of mixes that included organic material for soil functions. A dominant theme that has emerged over this time is mixing excavated deep horizons with organic waste due to the constant need to recycle and repurpose excavated deep horizon waste. Mixtures containing a small ratio of natural soils have also been shown to increase the colonization rate of macrofauna. A dominant conclusion that has emerged is that coupling the choice of waste mixture ratios and plants leads to a greater positive impact on soil functions than the choice of waste mixtures alone. Advantages of using Technosols include reduced economic costs associated with deposition of materials in landfill sites, lower remedial costs, reduced spending on fertilizer, lower public health risks due to healthier air quality from decreased transportation of dusty waste and urban-degraded soils, reduced soil and water pollution, increased vegetation which provides a cooler urban microclimate, lower greenhouse gas emissions, and greater food production in urban areas. However, this study confirmed that each element used to design constructed Technosols should be carefully considered. These elements include the ratio and the composition of waste, the order of horizons, environmental conditions, the choice of plant species, the implementation methods, and the critical need to foster pedogenic processes, especially during the first months following construction. There is a strong need for further research on how constructed Technosols can be used for multiple purposes in sites across the world. This research will contribute to urban sustainability and to fundamental knowledge on soil formation and function processes.
All data are published material available from various journals, which are provided in the reference list.
The research goals and aims of this paper are based on an original idea from MD, and GS helped to carry them out. MD prepared the paper, with contributions from all coauthors. PMG supervised MD and amended the whole text with numerous improvements. MB, SPE, AV, VV, DLC, DW, and TM all participated in editing the paper based on their respective expertise.
The authors declare that they have no conflict of interest.
The authors are thankful to Landry Collet for allowing the use of his pictures for publication (all photos in this paper are from Landry Collet, unless stated otherwise).
This research has been supported by the RUDN University's “5-100” project (grant no. 19-77-300-12).
This paper was edited by Cristine Carole Muggler and reviewed by two anonymous referees.
Akmar, A. N., Konijnendijk, C. C., Sreetheran, M., and Nilsson, K.: Greenspace planning and management in Klang valley, Peninsular Malaysia, Arboric. Urban. For., 37, 99–107, 2011.
Alizadehtazi, B., DiGiovanni, K., Foti, R., Morin, T., Shetty, N. H., Montalto, F. A., and Gurian, P. L.: Comparison of observed infiltration rates of different permeable urban surfaces using a cornell sprinkle infiltrometer, J. Hydrol. Eng., 21, 06016003, https://doi.org/10.1061/(ASCE)HE.1943-5584.0001374, 2016.
Bacholle, C., Leclerc, B., and Coppin, Y.: Utilisation de produits organiques en reconstitution de sol – Inventaire des pratiques en France – Etat de l'art des connaissances liées aux impacts de ces pratiques [Use of organic products in soil reconstitution – Inventory of practices in France – State of the art of knowledge related to the impacts of these practices], Agence de l'environnement et de la maîtrise de l'énergie (Ademe), Paris, Angers, Valbonne, Revue bimestrielle consacrée à l'actualité des travaux sur les matières organiques Echo-MO 59, 119 pp., 2006.
Bartens, J., Day, S. D., Harris, J. R., Dove, J. E., and Wynn, T. M.: Can urban tree roots improve infiltration through compacted subsoils for stormwater management?, J. Environ. Qual., 37, 2048–2057, https://doi.org/10.2134/jeq2008.0117, 2008.
Basta, N. T., Busalacchi, D. M., Hundal, L. S., Kumar, K., Dick, R. P., Lanno, R. P., Carlson, J., Cox, A. E., and Granato, T. C.: Restoring ecosystem function in degraded urban soil using biosolids, biosolids blend, and compost, J. Environ. Qual., 45, 74–83, https://doi.org/10.2134/jeq2015.01.0009, 2016.
Battaglia, A., Calace, N., Nardi, E., Petronio, B. M., and Pietroletti, M.: Reduction of Pb and Zn bioavailable forms in metal polluted soils due to paper mill sludge addition: Effects on Pb and Zn transferability to barley, Bioresour. Technol., 98, 2993–2999, https://doi.org/10.1016/j.biortech.2006.10.007, 2007.
Bettez, N. D. and Groffman, P. M.: Denitrification potential in stormwater control structures and natural riparian zones in an urban landscape, Environ. Sci. Technol., 46, 10909–10917, https://doi.org/10.1021/es301409z, 2012.
Beyers, J. L.: Postfire seeding for erosion control: Effectiveness and impacts on native plant communities, Conserv. Biol., 18. 947–956, https://doi.org/10.1111/j.1523-1739.2004.00523.x, 2004.
Blouin, M., Hodson, M. E., Delgado, E. A., Baker, G., Brussaard, L., Butt, K. R., Dai, J., Dendooven, L., Peres, G., Tondoh, J. E., Cluzeau D., and Brun J. J.: A review of earthworm impact on soil function and ecosystem services, Eur. J. Soil Sci., 64, 161–182, https://doi.org/10.1111/ejss.12025, 2013.
Bouzouidja, R., Séré, G., Claverie, R., Ouvrard, S., Nuttens, L., and Lacroix, D.: Green roof aging: Quantifying the impact of substrate evolution on hydraulic performances at the lab-scale, J. Hydrol., 564, 416–423, https://doi.org/10.1016/j.jhydrol.2018.07.032, 2018.
Bradshaw, A.: Restoration of mined lands – using natural processes, Ecol. Eng., 8, 255–269, https://doi.org/10.1016/S0925-8574(97)00022-0, 1997.
Brandon, D. L. and Price, R. A.: Summary of available guidance and best practices for determining suitability of dredged material for beneficial uses. Engineer Research and Development Center Vicksburg Ms Environmental Lab, available online: https://www.semanticscholar.org/paper/ (last access: 4 September 2020), 2007.
Bridgman, H. A., Warner, R. F., and Dodson, J. R.: Urban biophysical environments, Melbourne, Oxford University Press, 1–152, 1995.
Brown, S. L., Chaney, R. L., and Hettiarachchi, G. M.: Lead in urban soils: a real or perceived concern for urban agriculture?, J. Environ. Qual., 45, 26–36, https://doi.org/10.2134/jeq2015.07.0376, 2016.
Brunner, J. and Cozens, P.: Where Have All the Trees Gone?, Urban Consolidation and the Demise of Urban Vegetation: A Case Study from Western Australia, Plan. Pract. Res., 28, 231–255, https://doi.org/10.1080/02697459.2012.733525, 2013.
Byomkesh, T., Nakagoshi, N., and Dewan, A. M.: Urbanization and green space dynamics in Greater Dhaka, Bangladesh. Landsc. Ecol. Eng., 8, 45–58, https://doi.org/10.1007/s11355-010-0147-7, 2012.
Calace, N., Campisi, T., Iacondini, A., Leoni, M., Petronio, B. M., and Pietroletti, M.: Metal-contaminated soil remediation by means of paper mill sludges addition: chemical and ecotoxicological evaluation, Environ. Pollut., 136, 485–492, https://doi.org/10.1016/j.envpol.2004.12.014, 2005.
Cannavo, P., Guénon, R., Galopin, G., and Vidal-Beaudet, L.: Technosols made with various urban wastes showed contrasted performance for tree development during a 3-year experiment, Environ. Earth Sci., 77, 1–13, https://doi.org/10.1007/s12665-018-7848-x, 2018.
Cariolet, J.-M., Colombert, M., Vuillet, M., and Diab, Y.: Assessing the resilience of urban areas to traffic-related air pollution: Application in Greater Paris, Sci. Total. Environ., 615, 588–596, https://doi.org/10.1016/j.scitotenv.2017.09.334, 2018.
Cheng, Z., Paltseva, A., Li, I., Morin, T., Huot, H., Egendorf, S., Su, Z., Yolanda, R., Singh, K., Lee, L., Grinshtein, M., Green, K., Wai, W., Wazed, B., and Shaw, R.: Trace Metal Contamination in New York City Garden Soils, Soil Sci., 180, 167–174, https://doi.org/10.1097/SS.0000000000000126, 2015.
Chillrud, S. N., Bopp, R. F., Simpson, H. J., Ross, J. M., Shuster, E. L., Chaky, D. A., Walsh, D. C., Choy, C. C., Tolley, L.-R., and Yarme, A.: Twentieth century atmospheric metal fluxes into central park lake, New York City, Environ. Sci. Technol., 33, 657–662, https://doi.org/10.1021/es9807892, 1999.
Coley, R. L., Sullivan, W. C., and Kuo, F. E.: Where does community grow? The social context created by nature in urban public housing, Environ. Behav., 29, 468–494, https://doi.org/10.1177/001391659702900402, 1997.
Corradini, F., Meza, P., Eguiluz, R., Casado, F., Huerta-Lwanga, E., and Geissen, V.: Evidence of microplastic accumulation in agricultural soils from sewage sludge disposal, Sci. Total Environ., 671, 411–420, https://doi.org/10.1016/j.scitotenv.2019.03.368, 2019.
Craul, P. J.: Urban Soils: Applications and Practices, New York, John Wiley and Sons, 85–115, 1999.
Creger, T. L. and Peryea, F. J.: Phosphate Fertilizer Enhances Arsenic Uptake by Apricot Liners Grown in Lead-arsenate-enriched Soil, HortScience, 29, 88–92, https://doi.org/10.21273/HORTSCI.29.2.88, 1994.
Culbertson, T. L. and Hutchinson S. L.: Assessing Bioretention Cell Function in a Midwest Continental Climate, Ottawa, Canada 1–4 August 2004, American Society of Agricultural and Biological Engineers, Meeting Paper No. 047051, ASAE, St Joseph, Mich., 7814–7852, 047051, https://doi.org/10.13031/2013.17124, 2004.
Czech, B., Krausman, P. R., and Devers, P. K.: Economic Associations among Causes of Species Endangerment in the United States Associations among causes of species endangerment in the United States reflect the integration of economic sectors, supporting the theory and evidence that economic growth proceeds at the competitive exclusion of nonhuman species in the aggregate, BioScience, 50, 593–601, https://doi.org/10.1641/0006-3568(2000)050[0593:EAACOS]2.0.CO;2, 2000.
Damas, O. and Coulon, A.: Créer des sols fertiles: du déchet à la végétalisation urbaine, Create fertile soils: from waste to urban greening, Paris, Editions Le Moniteur, 335 pp., 2016.
Darmody, R. G. and Marlin, J. C.: Sediments and sediment-derived soils in Illinois: Pedological and agronomic assessment, Environ. Monit. Assess., 77, 209–227, https://doi.org/10.1023/A:1015880004383, 2002.
Das, I., Pedit, J., Handa, S., and Jagger, P.: Household air pollution (HAP), microenvironment and child health: Strategies for mitigating HAP exposure in urban Rwanda, Environ. Res. Lett., 13, 045011, https://doi.org/10.1008/1748-9326/aab047, 2018.
Daunay, M.: La création de sols de plantation en mélange terre-pierres pour les arbres d'alignement, à travers l'exemple d'une réalisation faite par Viapark, à Nanterre, The creation of planting soil mixed land-stones to roadside trees, through the example of an achievement made by Viapark in Nanterre, Le sol, support de nos plantations, Livre blanc du colloque de l'UNEP, 2 Juin 1999, Paris, 44–47, 1998.
Davis, A. P., Hunt, W. F., Traver, R. G., and Clar, M.: Bioretention Technology: Overview of Current Practice and Future Needs, J. Environ. Eng., 135, 109–117, https://doi.org/10.1061/(ASCE)0733-9372(2009)135:3(109), 2009.
DEBNY (New York City Department of Environmental Protection): available at: http://www.developmentexcellence.com/tools/docs/NYC%20Green%20Infrastructure%20Plan.pdf, last access: 28 September 2010.
Deeb, M., Grimaldi, M., lerch, T. Z., Pando, A., Podwojewski, P., and Blouin, M.: Influence of organic matter content on hydro-structural properties of constructed Technosols, Pedosphere, 26, 486–498, https://doi.org/10.1016/S1002-0160(15)60059-5, 2016a.
Deeb, M., Grimaldi, M., Lerch, T. Z., Pando, A., Gigon, A., and Blouin, M.: Interactions between organisms and parent materials of a constructed Technosol shape its hydrostructural properties, SOIL, 2, 163–174, https://doi.org/10.5194/soil-2-163-2016, 2016b.
Deeb, M.: Influence des plantes, des vers de terre et de la matière organique sur la structure de technosols construits, The effect of earthworms, plants and organic matters on structure of Constructed Technosols, Ph.D. thesis, UPEC, Université Paris-Est Créteil – UMR IEES – Institut d'Ecologie et des Sciences de l'Environnement de Paris, Créteil, France, 192 pp., 2016c.
Deeb, M., Desjardins, T., Podwojewski, P., Pando, A., Blouin, M., and Lerch, T. Z.: Interactive effects of compost, plants and earthworms on the aggregations of constructed Technosols, Geoderma, 305, 305–313, https://doi.org/10.1016/j.geoderma.2017.06.014, 2017.
Deeb, M., Groffman, P. M., Joyner, J. L., Lozefski, G., Paltseva, A., Lin, B., Mania, K., Cao, D. L., McLaughlin, J., Muth, T., Prithiviraj, B., Kerwin, J., and Cheng, Z.: Soil and microbial properties of green infrastructure stormwater management systems, Ecol. Eng., 125, 68–75, https://doi.org/10.1016/j.ecoleng.2018.10.017, 2018.
De Kimpe, C. R. and Morel, J. L.: Urban soil management: A growing concern, Soil Sci., 165, 31–40, 2000.
De Sousa, C. A.: Turning brownfields into green space in the City of Toronto, Landsc. Urban Plan., 62, 181–198, https://doi.org/10.1016/S0169-2046(02)00149-4, 2003.
Dick, D. P., Knicker, H., Ávila, L. G., Inda Jr., A. V., Giasson, E., and Bissani, C. A.: Organic matter in constructed soils from a coal mining area in southern Brazil, Org. Geochem., 37, 1537–1545, https://doi.org/10.1016/j.orggeochem.2006.06.017, 2006.
Dickinson, N. M.: Strategies for sustainable woodland on contaminated soils, Chemosphere, 41, 259–263, https://doi.org/10.1016/S0045-6535(99)00419-1, 2000.
Doni, A., Murthy, C., and Kurian, M. Z.: Survey on multi sensor based air and water quality monitoring using IoT, Indian J. Sci. Res., 17, 147–153, 2018.
Dornbush, M. E.: Plant community change following fifty-years of management at Kalsow Prairie Preserve, Iowa, USA Am. Midl. Nat., 151, 241–250, 2004.
Dubik, S. P., Krizek, D. T., and Stimart, D. P.: Influence of root zone restriction on mineral element concentration, water potential, chlorophyll concentration, and partitioning of assimilate in spreading euonymus (E. Kiautschovica Loes. “Sieboldiana”), J. Plant Nutr., 13, 677–699, https://doi.org/10.1080/01904169009364109, 1990.
Egendorf, S. P., Cheng, Z., Deeb, M., Flores, V., Paltseva, A., Walsh, D., Groffman, P., and Mielke, H. W.: Constructed soils for mitigating lead (Pb) exposure and promoting urban community gardening: The New York City Clean Soil Bank pilot study, Landsc. Urban Plan., 175, 184–194, https://doi.org/10.1016/j.landurbplan.2018.03.012, 2018.
European Environment Agency: Urban green infrastructure, available at: https://www.eea.europa.eu/themes/sustainability-transitions/urban-environment/urban-green-infrastructure (last access: 4 September 2020), 2017.
European Commission: Construction and Demolition Waste, available at: https://ec.europa.eu/environment/waste/construction_demolition.htm (last access: 16 May 2020), 2020.
Fletcher, R. J. and Koford, R. R.: Habitat and landscape associations of breeding birds in native and restored grasslands, J. Wildl. Manage., 66, 1011–1022, https://doi.org/10.2307/3802933, 2002.
Flores, A., Pickett, S. T., Zipperer, W. C., Pouyat, R. V., and Pirani, R.: Adopting a modern ecological view of the metropolitan landscape: the case of a greenspace system for the New York City region, Landsc. Urban Plan., 39, 295–308, https://doi.org/10.1016/S0169-2046(97)00084-4, 1998.
Flores-Ramírez, E., Dominik, P., and Kaupenjohann, M.: Coating a dredged sand with recycled ferrihydrites to create a functional material for plant substrate, J. Soils Sediments, 18, 534–545, https://doi.org/10.1007/s11368-017-1772-7, 2018.
Foley, J. A., DeFries, R., Asner, G. P., Barford, C., Bonan, G., Carpenter, S. R., Chapin, F. S., Coe, M. T., Daily, G. C., Gibbs, H. K., Helkowski, J. H., Holloway, T., Howard, E. A., Kucharik, C. J., Monfreda, C., Patz, J. A., Prentice, C., Ramankutty, N., and Snyder, P. K.: Global Consequences of Land Use, Science, 309, 570–574, https://doi.org/10.1126/science.1111772, 2005.
Fourvel, G. J., Vidal-Beaudet, L., Le Bocq, A., Thery, F., Brochier, V., and Cannavo, P.: Fertility of Technosols constructed with dam sediments for urban greening and land reclamation, J. Soils Sediments, 19, 3178–3192, https://doi.org/10.1007/s11368-018-2077-1, 2018.
Gálvez-Martos, J.-L., Styles, D., Schoenberger, H., and Zeschmar-Lahl, B.: Construction and demolition waste best management practice in Europe, Resources, Conservation and Recycling, 136, 166–178, https://doi.org/10.1016/j.resconrec.2018.04.016 , 2018.
Geneletti, D., Biasiolli, A., and Morrison-Saunders, A.: Land take and the effectiveness of project screening in Environmental Impact Assessment: Findings from an empirical study, Environ. Impact Assess., 67, 117–123, https://doi.org/10.1016/j.eiar.2017.08.008, 2017.
Gill, A. S., Lee, A., and McGuire, K. L.: Phylogenetic and functional diversity of total (DNA) and expressed (RNA) bacterial communities in urban green infrastructure bioswale soils, Appl. Environ. Microbiol., 83, AEM.00287-17, https://doi.org/10.1128/AEM.00287-17, 2017.
Godefroid, S. and Koedam, N.: Urban plant species patterns are highly driven by density and function of built-up areas, Landsc. Ecol., 22, 1227–1239, https://doi.org/10.1007/s10980-007-9102-x, 2007.
Gómez-Sagasti, M. T., Hernández, A., Artetxe, U., Garbisu, C., and Becerril, J. M.: How Valuable Are Organic Amendments as Tools for the Phytomanagement of Degraded Soils? The Knowns, Known Unknowns, and Unknowns, Front. Sustain. Food Syst., 2, 1–16, https://doi.org/10.3389/fsufs.2018.00068, 2018.
Grabosky, J. and Basset, N.: A new urban tree soil to safely increase rooting volumes under sidewalks, Arboric J., 187–201, 1995.
Grard, B., Bel, N., Marchal, N., Madre, N., Castell, J.-F., Cambier, P., Houot, S., Manouchehri, N., Besancon, S., Michel, J.C., Chenu, C., Frascaria-Lacoste, N., and Aubry, C.: Recycling urban waste as possible use for rooftop vegetable garden, Recycling urban waste as possible use for rooftop vegetable garden, Future of food: Journal on Food, Agriculture and Society, 3, 21–34, 2015.
Grard, B. J.-P., Chenu, C., Manouchehri, N., Houot, S., Frascaria-Lacoste, N., and Aubry, C.: Rooftop farming on urban waste provides many ecosystem services, Agron. Sustain. Dev., 38, 1–12, https://doi.org/10.1007/s13593-017-0474-2, 2018.
Grosbellet, C.: Evolution et effet sur la structuration du sol de la matière organique apporté en grande quantité, Evolution and effects of great quantities of organic matter on soil structuration, Ph.D. thesis, Université d'Angers, 237 pp., available at: http://www.theses.fr/2008ANGE0057 (4 September 2020), 2008.
Guilland, C., Maron, P. A., Damas, O., and Ranjard, L.: Biodiversity of urban soils for sustainable cities, Environ. Chem. Lett., 16, 1267–1282, https://doi.org/10.1007/s10311-018-0751-6, 2018.
Haaland, C. and van den Bosch, C. K.: Challenges and strategies for urban green-space planning in cities undergoing densification: A review, Urban For. Urban Gree., 14, 760–771, https://doi.org/10.1016/j.ufug.2015.07.009, 2015.
Haddad, N. M., Brudvig, L. A., Clobert, J., Davies, K. F., Gonzalez, A., Holt, R. D., Lovejoy, T. E., Sexton, J. O., Austin, M. P., and Collins, C. D.: Habitat fragmentation and its lasting impact on Earth's ecosystems, Sci. Adv., 1, e1500052, https://doi.org/10.1126/sciadv.1500052, 2015.
Hafeez, F., Spor, A., Breuil, M.-C., Schwartz, C., Martin-Laurent, F., and Philippot, L.: Distribution of bacteria and nitrogen-cycling microbial communities along constructed Technosol depth-profiles, J. Hazard. Mater., 231/232, 88–97, https://doi.org/10.1016/j.jhazmat.2012.06.041, 2012a.
Hafeez, F., Martin-Laurent, F., Béguet, J., Bru, D., Cortet, J., Schwartz, C., Morel, J.-L., and Philippot, L.: Taxonomic and functional characterization of microbial communities in Technosols constructed for remediation of a contaminated industrial wasteland, J. Soils Sediments, 12, 1396–1406, https://doi.org/10.1007/s11368-012-0563-4, 2012b.
Hall, T.: Goodbye to the Backyard? – The Minimisation of Private Open Space in the Australian Outer-Suburban Estate, Urban Pol. Res., 28, 411–433, https://doi.org/10.1080/08111146.2010.496715, 2010.
Halonen, J. I., Hansell, A. L., Gulliver, J., Morley, D., Blangiardo, M., Fecht, D., Toledano, M. B., Beevers, S. D., Anderson, H. R., and Kelly, F. J.: Road traffic noise is associated with increased cardiovascular morbidity and mortality and all-cause mortality in London, Eur. Heart J., 36, 2653–2661, https://doi.org/10.1093/eurheartj/ehv216, 2015.
Heimsath, A. M., Dietrich, W. E., Nishiizumi, K., and Finkel, R. C.: The soil production function and landscape equilibrium, Nature, 388, 358–361, 1997.
Hendriks, C. F. and Pietersen, H. S.: Report 22: Sustainable Raw Materials: Construction and Demolition Waste – State-of-the-Art Report of RILEM Technical Committee 165-SRM (RILEM Publications), 2000.
Horton, A. A., Walton, A., Spurgeon, D. J., Lahive, E., and Svendsen, C.: Microplastics in freshwater and terrestrial environments: Evaluating the current understanding to identify the knowledge gaps and future research priorities, Sci. Total Environ., 586, 127–141, https://doi.org/10.1016/j.scitotenv.2017.01.190, 2017.
Hui, N., Jumpponen, A., Francini, G., Kotze, D. J., Liu, X., Romantschuk, M., Strömmer, R., and Setälä, H.: Soil microbial communities are shaped by vegetation type and park age in cities under cold climate, Environ. Microbiol., 19, 1281–1295, https://doi.org/10.1111/1462-2920.13660, 2017.
Huot, H., Joyner, J., Córdoba, A., Shaw, R. K., Wilson, M. A., Walker, R., Muth, T. R., and Cheng, Z.: Characterizing urban soils in New York City: profile properties and bacterial communities, J. Soils Sediments, 17, 393–407, https://doi.org/10.1007/s11368-016-1552-9, 2017.
Hüttl, R. F. and Bradshaw, A.: Aspect of Reclamation Ecology, Landsc. Urban Plan., 51, 73–74, https://doi.org/10.1016/S0169-2046(00)00120-1, 2000.
Isaacs, R., Tuell, J., Fiedler, A., Gardiner, M., and Landis, D.: Maximizing arthropod-mediated ecosystem services in agricultural landscapes: the role of native plants, Front. Ecol. Environ., 7, 196–203, https://doi.org/10.1890/080035, 2009.
IUSS Working Group WRB: World Reference Base for Soil Resources 2014, update 2015 International soil classification system for naming soils and creating legends for soil maps, World Soil Resources Reports No. 106, FAO, Rome, p. 87, 2015.
Jangorzo, N., Watteau, F., and Schwartz, C.: Ranking of wetting–drying, plant, and fauna factors involved in the structure dynamics of a young constructed Technosol, J. Soil. Sediments, 18, 2995, https://doi.org/10.1007/s11368-018-1968-5, 2018.
Jangorzo, N. S., Watteau, F., and Schwartz, C.: Evolution of the pore structure of constructed Technosols during early pedogenesis quantified by image analysis, Geoderma, 207/208, 180–192, https://doi.org/10.1016/j.geoderma.2013.05.016, 2013.
Jangorzo, N. S., Watteau, F., Hajos, D., and Schwartz, C.: Nondestructive monitoring of the effect of biological activity on the pedogenesis of a Technosol, J. Soil. Sediments, 15, 1705–1715, https://doi.org/10.1007/s11368-014-1008-z, 2014.
Jiang, Y., Zhuang, G., Wang, Q., Huang, K., Deng, C., Yu, G., Xu, C., Fu, Q., Lin, Y., and Fu, J. S.: Impact of mixed anthropogenic and natural emissions on air quality and eco-environment – the major water-soluble components in aerosols from northwest to offshore isle, Air Qual. Atmos. Hlth., 11, 521–534, https://doi.org/10.1007/s11869-018-0557-5, 2018.
Jim, C. Y.: Urban soil characteristics and limitations for landscape planting in Hong Kong, Landsc. Urban Plan., 40, 235–249, https://doi.org/10.1016/S0169-2046(97)00117-5, 1998.
Jim, C. Y.: Monitoring the performance and decline of heritage trees in urban Hong Kong, J. Environ. Manage., 74, 161–172, https://doi.org/10.1016/j.jenvman.2004.08.014, 2005.
Joyner, J. L., Kerwin, J., Deeb, M., Lozefski, G., Prithiviraj, B., Paltseva, A., McLaughlin, J., Groffman, P., Cheng, Z., and Muth, T. R.: Green Infrastructure Design Influences Communities of Urban Soil Bacteria, Front. Microbiol., 10, 1–14, https://doi.org/10.3389/fmicb.2019.00982, 2019.
Kabisch, N. and Haase, D.: Green spaces of European cities revisited for 1990–2006, Landsc. Urban Plan., 110, 113–122, https://doi.org/10.1016/j.landurbplan.2012.10.017, 2013.
Kazemi, F., Beecham, S., and Gibbs, J.: Streetscape biodiversity and the role of bioretention swales in an Australian urban environment, Landsc. Urban Plan., 101, 139–148, https://doi.org/10.1016/j.landurbplan.2011.02.006, 2011.
Keeley, M., Koburger, A., Dolowitz, D. P., Medearis, D., Nickel, D., and Shuster, W.: Perspectives on the use of green infrastructure for stormwater management in Cleveland and Milwaukee, Environ. Manage., 51, 1093–1108, https://doi.org/10.1007/s00267-013-0032-x, 2013.
Kessler, R.: Urban gardening: managing the risks of contaminated soil (National Institute of Environmental Health Sciences), Environ. Health Perspect., 121, A326–A333, https://doi.org/10.1289/ehp.121-A326, 2013.
Khan, J., Ketzel, M., Kakosimos, K., Sørensen, M., and Jensen, S. S.: Road traffic air and noise pollution exposure assessment – A review of tools and techniques, Sci. Total Environ., 634, 661–676, https://doi.org/10.1016/j.scitotenv.2018.03.374, 2018.
Krook, J., Svensson, N., and Eklund, M.: Landfill mining: A critical review of two decades of research, Waste Management, 32, 513–520, https://doi.org/10.1016/j.wasman.2011.10.015, 2012.
Kumar, S., Malik, R., and Dahiya, I.: Influence of different organic wastes upon water retention, transmission and contact characteristics of a sandy soil, Soil Res., 23, 131–136, https://doi.org/10.1071/SR9850131, 1985.
Laidlaw, M. A., Filippelli, G. M., Brown, S., Paz-Ferreiro, J., Reichman, S. M., Netherway, P., Truskewycz, A., Ball, A. S., and Mielke, H. W.: Case studies and evidence-based approaches to addressing urban soil lead contamination, Appl. Geochem., 83, 14–30, https://doi.org/10.1016/j.apgeochem.2017.02.015, 2017.
Larney, F. J. and Angers, D. A.: The role of organic amendments in soil reclamation: A review, Can. J. Soil Sci., 92, 19–38, https://doi.org/10.4141/cjss2010-064, 2012.
Latif, M. T., Othman, M., Idris, N., Juneng, L., Abdullah, A. M., Hamzah, W. P., Khan, M. F., Nik Sulaiman, N. M., Jewaratnam, J., Aghamohammadi, N., Sahani, M., Xiang, J. C., Ahmad, F., Amil, N., Darus, M., Varkkey, H., Tangang, F., and Jaafar, A. B.: Impact of regional haze towards air quality in Malaysia: A review, Atmos. Environ., 177, 28–44, https://doi.org/10.1016/j.atmosenv.2018.01.002, 2018.
Lee, A. C. K. and Maheswaran, R.: The health benefits of urban green spaces: a review of the evidence, J. Public Health, 33, 212–222, https://doi.org/10.1093/pubmed/fdq068, 2011.
Lehman, A.: Technosols and other proposals on urban soils for the WRB (World Reference Base for Soil Resources), Int. Agrophys., 20, 129–134, 2006.
Lewis, J.: The Potential Fate of Leftover Green Space Areas in Loughborough-Garendon, Arboric. J., 29, 43–54, https://doi.org/10.1080/03071375.2005.9747441, 2005.
Li, Z., Zhang, G., Liu, Y., Wan, K., Zhang, R., and Chen, F.: Soil nutrient assessment for urban ecosystems in Hubei, China. PloS One, 8, e75856, https://doi.org/10.1371/journal.pone.0075856, 2013.
Lindsey, P. and Bassuk, N.: Redesigning the urban forest from the ground below: A new approach to specifying adequate soil volumes for street trees, Arboric. J., 16, 25–39, https://doi.org/10.1080/03071375.1992.9746896, 1992.
Liu, W., Chen, W., and Peng, C.: Assessing the effectiveness of green infrastructures on urban flooding reduction: A community scale study, Ecol. Model., 291, 6–14, https://doi.org/10.1016/j.ecolmodel.2014.07.012, 2014.
Lovett, G. M., Traynor, M. M., Pouyat, R. V., Carreiro, M. M., Zhu, W.-X., and Baxter, J. W.: Atmospheric Deposition to Oak Forests along an Urban−Rural Gradient, Environ. Sci. Technol., 34, 4294–4300, https://doi.org/10.1021/es001077q, 2000.
Lucas, W. and Greenway, M.: Phosphorus retention performance in vegetated and non-vegetated bioretention mesocosms using recycled effluent, Rainwater and Urban Design 2007, edited by: Barton, A. C. T., Engineers Australia, 696–703, 2007.
Lucas, W. C. and Sample, D. J.: Reducing combined sewer overflows by using outlet controls for Green Stormwater Infrastructure: Case study in Richmond, Virginia, J. Hydrol., 520, 473–488, https://doi.org/10.1016/j.jhydrol.2014.10.029, 2015.
Ma, Q. Y., Logan, T. J., and Traina, S. J.: Lead Immobilization from Aqueous Solutions and Contaminated Soils Using Phosphate Rocks, Environ. Sci. Technol., 29, 1118–1126, https://doi.org/10.1021/es00004a034, 1995.
Madadi, H., Moradi, H., Soffianian, A., Salmanmahiny, A., Senn, J., and Geneletti, D.: Degradation of natural habitats by roads: Comparing land-take and noise effect zone, Environ. Impact Asses., 65, 147–155, https://doi.org/10.1016/j.eiar.2017.05.003, 2017.
Maes, J., Barbosa, A., Baranzelli, C., Zulian, G., Batista e Silva, F., Vandecasteele, I., Hiederer, R., Liquete, C., Paracchini, M.L., Mubareka, S., Crisioni, C. J., Castillo, C. P., and Lavalle, C.: More green infrastructure is required to maintain ecosystem services under current trends in land-use change in Europe, Landsc. Ecol., 30, 517–534, https://doi.org/10.1007/s10980-014-0083-2, 2015.
Marié, X. and Rossignol, J. P.: Les “Anthroposols reconstitués” pour les espaces verts, “Anthroposols reconstituted” for green spaces, Int. ISHS Symp, “La Santé de l'arbre urbain” 22–26 September 1997, Paris, Acta Horticulturae, no. 496, 361-368, 1997.
Marquez-Bravo, L. G., Briggs, D., Shayler, H., McBride, M., Lopp, D., Stone, E., Ferenz, G., Bogdan, K. G., Mitchell, R. G., and Spliethoff, H. M.: Concentrations of polycyclic aromatic hydrocarbons in New York City community garden soils: Potential sources and influential factors, Environ. Toxicol. Chem., 35, 357–367, https://doi.org/10.1002/etc.3215, 2016.
Maurer, M., Klemm, O., Lokys, H. L., and Lin, N.-H.: Trends of Fog and Visibility in Taiwan: Climate Change or Air Quality Improvement?, Aerosol Air Qual. Res., 19, 896–910, https://doi.org/10.4209/aaqr.2018.04.0152, 2019.
McBride, M. B., Shayler, H. A., Spliethoff, H. M., Mitchell, R. G., Marquez-Bravo, L. G., Ferenz, G. S., Russell-Anelli, J. M., Casey, L., and Bachman, S.: Concentrations of lead, cadmium and barium in urban garden-grown vegetables: the impact of soil variables, Environ. Pollut., 194, 254–261, https://doi.org/10.1016/j.envpol.2014.07.036, 2014.
McGeehan, S.L.: Impact of Waste Materials and Organic Amendments on Soil Properties and Vegetative Performance, Appl. Environ. Soil. Sci., 907831, https://doi.org/10.1155/2012/907831, 2012.
McGrane, S. J.: Impacts of urbanisation on hydrological and water quality dynamics, and urban water management: a review, Hydrolog. Sci. J., 61, 2295–2311, https://doi.org/10.1080/02626667.2015.1128084, 2016.
McKinney, M. L.: Urbanization, Biodiversity, and Conservation The impacts of urbanization on native species are poorly studied, but educating a highly urbanized human population about these impacts can greatly improve species conservation in all ecosystems, Bioscience, 52, 883–890, https://doi.org/10.1641/0006-3568(2002)052[0883:UBAC]2.0.CO;2, 2002.
McKinney, M. L.: Effects of urbanization on species richness: a review of plants and animals, Urban Ecosyst., 11, 161–176, https://doi.org/10.1007/s11252-007-0045-4, 2008.
McPherson, E. G., van Doorn, N., and de Goede, J.: Structure, function and value of street trees in California, USA, Urban. For. Urban. Green, 17, 104–115, https://doi.org/10.1016/j.ufug.2016.03.013, 2016.
McPhillips, L. and Walter, M. T.: Hydrologic conditions drive denitrification and greenhouse gas emissions in stormwater detention basins, Ecol. Eng., 85, 67–75, https://doi.org/10.1016/j.ecoleng.2015.10.018, 2015.
McPhillips, L., Goodale, C., and Walter, M. T.: Nutrient Leaching and Greenhouse Gas Emissions in Grassed Detention and Bioretention Stormwater Basins, Journal of Sustainable Water in the Built Environment, 4, 04017014, https://doi.org/10.1061/JSWBAY.0000837, 2018.
Mell, I. C., Henneberry, J., Hehl-Lange, S., and Keskin, B.: To green or not to green: Establishing the economic value of green infrastructure investments in The Wicker, Sheffield, Urban. For. Urban. Gree., 18, 257–267, https://doi.org/10.1016/j.ufug.2016.06.015, 2016.
Mielke, H. W., Anderson, J. C., Berry, K. J., Mielke, P. W., Chaney, R. L., and Leech, M.: Lead concentrations in inner-city soils as a factor in the child lead problem, Am. J. Public Health, 73, 1366–1369, 1983.
Mitchell, R. G., Spliethoff, H. M., Ribaudo, L. N., Lopp, D. M., Shayler, H.A., Marquez-Bravo, L. G., Lambert, V. T., Ferenz, G. S., Russell-Anelli, J. M., and Stone, E. B.: Lead (Pb) and other metals in New York City community garden soils: factors influencing contaminant distributions, Environ. Pollut., 187, 162–169, https://doi.org/10.1016/j.envpol.2014.01.007, 2014.
Morel, J. L., Chenu, C., and Lorenz, K.: Ecosystem services provided by soils of urban, industrial, traffic, mining, and military areas (SUITMAs), J. Soils Sediments, 15, 1–8, https://doi.org/10.1007/s11368-014-0926-0, 2014.
Morse, N. R., McPhillips, L. E., Shapleigh, J. P., and Walter, M. T.: The Role of Denitrification in Stormwater Detention Basin Treatment of Nitrogen, Environ. Sci. Technol., 51, 7928–7935, https://doi.org/10.1021/acs.est.7b01813, 2017.
Muir, P. S. and McCune, B.: Lichens, tree growth, and foliar symptoms of air pollution: are the stories consistent?, J. Environ. Qual., 17, 361–370, https://doi.org/10.2134/jeq1988.00472425001700030004x, 1988.
Narkhede, S. D., Jadhav, R. N., Khatik, V. A., Ingle, S. T., and Attarde, S. B.: Composting and its applicability in developing countries, Int. J. Agr. Sci., 6, 343–349, 2010.
Nemati, M. R., Caron, J., and Gallichand, J.: Stability of Structural Form during Infiltration Laboratory Measurements on the Effect of De-inking Sludge, Soil Sci. Soc. Am. J., 64, 543–552, https://doi.org/10.2136/sssaj2000.642543x, 2000.
New York Codes, Rules and Regulations (NY-CRR), Remedial program soil cleanup objectives, available at: https://www.dec.ny.gov/docs/remediation_hudson_pdf/part375.pdf (last access: 4 September 2020), 2017.
NYC Green Infrastructure Plan (NYCGIP): Preliminary Pilot Monitoring Results, available at: https://www1.nyc.gov/assets/dep/ (last access: 4 September 2020) 2011.
O'Dell, R., Young, S., and Claassen, V.: Native roadside perennial grasses persist a decade after planting in the Sacramento Valley, Calif. Agr., 61, 79–84, https://doi.org/10.3733/ca.v061n02p79, 2000.
O'Neill, B. C., M. Done, J., Gettelman, A., Lawrence, P., Lehner, F., Lamarque, J.-F., Lin, L., J. Monaghan, A., Oleson, K., Ren, X., Sanderson, B. M., Tebaldi, C., Weitzel, M., Xu, Y., Anderson, B., Fix, M. J., and Levis, S.: The benefits of reduced Anthropogenic climate change (BRACE): a synthesis, Clim. Change, 146, 287–301, https://doi.org/10.1007/s10584-017-2009-x, 2018.
Opdam, P., Steingröver, E., and van Rooij, S.: Ecological networks: A spatial concept for multi-actor planning of sustainable landscapes, Landsc. Urban Plan., 75, 322–332, https://doi.org/10.1016/j.landurbplan.2005.02.015, 2006.
Paltseva, A., Cheng, Z., Deeb, M., Groffman, P. M., Shaw, R. K., and Maddaloni, M.: Accumulation of arsenic and lead in garden-grown vegetables: Factors and mitigation strategies, Sci. Total. Environ., 640, 273–283, https://doi.org/10.1016/j.scitotenv.2018.05.296, 2018a.
Paltseva, A., Cheng, Z., Deeb, M., Groffman, P. M., and Maddaloni, M.: Variability of Bioaccessible Lead in Urban Garden Soils, Soil Sci., 183 123–131, https://doi.org/10.1097/SS.0000000000000232, 2018b.
Panduro, T. E. and Veie, K. L.: Classification and valuation of urban green spaces – A hedonic house price valuation, Landsc. Urban Plan., 120, 119–128, https://doi.org/10.1016/j.landurbplan.2013.08.009, 2013.
Pauleit, S., Ennos, R., and Golding, Y.: Modeling the environmental impacts of urban land use and land cover change – a study in Merseyside, UK, Landsc. Urban Plan., 71, 295–310, https://doi.org/10.1016/j.landurbplan.2004.03.009, 2005.
Pey, B., Cortet, J., Watteau, F., Cheynier, K., and Schwartz, C.: Structure of earthworm burrows related to organic matter of a constructed Technosol, Geoderma, 202, 103–111, https://doi.org/10.1016/j.geoderma.2013.03.010, 2013a.
Pey, B., Cortet, J., Watteau, F., Cheynier, K., and Schwartz, C. Structure of earthworm burrows related to organic matter of a constructed Technosol, Geoderma, 202, 103–111, https://doi.org/10.1016/j.geoderma.2013.03.010, 2013b.
Pey, B., Cortet, J., Capowiez, Y., Nahmani, J., Watteau, F., and Schwartz, C.: Technosol composition affects Lumbricus terrestris surface cast composition and production, Ecol. Eng., 67, 238–247, https://doi.org/10.1016/j.ecoleng.2014.03.039, 2014.
Pickett, S. T. A., Cadenasso, M. L., Grove, J. M., Boone, C. G., Groffman, P. M., Irwin, E., Kaushal, S. S., Marshall, V., McGrath, B. P., Nilon, C. H., Pouyat, R. V., Szlavecz, K., Troy, A., and Warren, P.: Urban ecological systems: Scientific foundations and a decade of progress, J. Environ. Manage., 92, 331–362, https://doi.org/10.1016/j.jenvman.2010.08.022, 2011.
Pinedo, J., Ibáñez, R., Lijzen, J. P. A., Irabien, Á.: Assessment of soil pollution based on total petroleum hydrocarbons and individual oil substances, J. Environ. Manage., 130, 72–79, https://doi.org/10.1016/j.jenvman.2013.08.048, 2013.
Pistocchi, A., Calzolari, C., Malucelli, F., and Ungaro, F.: Soil sealing and flood risks in the plains of Emilia-Romagna, Italy, J. Hydrol. Reg. Stud., 4, 398–409, https://doi.org/10.1016/j.ejrh.2015.06.021, 2015.
Pouyat, R. V., Szlavecz, K., Yesilonis, I. D., Groffman, P. M., and Schwarz, K.: Chemical, physical and biological characteristics of urban soils, chap. 7, Aitkenhead-Peterson Jacqueline Volder Astrid Eds Urban Ecosystems Ecology, Agronomy. Monogr., 55 Madison WI: American Society of Agronomy, Crop Science Society of America, Soil Science, 119–152, https://doi.org/10.2134/agronmonogr55.c7, 2010.
Prokofyeva, T. V., Martynenko, I. A., Ivannikov, F. A.: Classification of Moscow soils and parent materials and its possible inclusion in the classification system of Russian soils, Euras. Soil Sci., 44, 561–571, https://doi.org/10.1134/S1064229311050127, 2011.
Pruvost, C.: Potentiel de la Biodiversité dans la construction de Technosols à partir de déchets urbains, Potential of Biodiversity in the construction of Technosols from urban waste, Ph.D. thesis, Université Paris-Est; École doctorale Sciences, Ingénierie et Environnement Laboratoire: IEES Paris – Institut d'Ecologie et des Sciences de l'Environnement de Paris (laboratoire), 173 pp., http://www.theses.fr/2018PESC1161 (last access: 4 September 2020), 2018.
Pruvost, C., Mathieu, J., Nunan, N., Gigon, A., Pando, A., Lerch, T. Z., and Blouin, M.: Tree growth and macrofauna colonization in Technosols constructed from recycled urban wastes, Ecol. Eng., 153, 105886, https://doi.org/10.1016/j.ecoleng.2020.105886, 2020.
Pugh, T. A. M., MacKenzie, A. R., Whyatt, J. D., and Hewitt, C. N.: Effectiveness of green infrastructure for improvement of air quality in urban street canyons, Environ. Sci. Technol., 46, 7692–7699, https://doi.org/10.1021/es300826w, 2012.
Puhalla, J., Krans, J., and Goatley, M.: Sports fields: A manual for design, construction and maintenance, John Wiley and Sons, Hoboken, NJ, 1–443, 1999.
Qureshi, S., Hasan Kazmi, S. J., and Breuste, J. H.: Ecological disturbances due to high cutback in the green infrastructure of Karachi: Analyses of public perception about associated health problems, Urban For. Urban Gree., 9, 187–198, https://doi.org/10.1016/j.ufug.2009.08.003, 2010.
Rafiee, R., Salman Mahiny, A., and Khorasani, N.: Assessment of changes in urban green spaces of Mashad city using satellite data, Int. J. Appl. Earth Obs., 11, 431–438, https://doi.org/10.1016/j.jag.2009.08.005, 2009.
Rao, P., Hutyra, L. R., Raciti, S. M., and Templer, P. H.: Atmospheric nitrogen inputs and losses along an urbanization gradient from Boston to Harvard Forest, MA, Biogeochemistry, 121, 229–245, https://doi.org/10.1007/s10533-013-9861-1, 2014.
Richardson, D. M., Holmes, P. M., Esler, K. J., Galatowitsch, S. M., Stromberg, J. C., Kirkman, S. P., Pyšek, P., and Hobbs, R. J.: Riparian vegetation: degradation, alien plant invasions, and restoration prospects, Divers. Distrib., 13, 126–139, https://doi.org/10.1111/j.1366-9516.2006.00314.x, 2007.
Ries, L., Debinski, D. M., and Wieland, M. L.: Conservation value of roadside prairie restoration to Butterfly Communities, Conserv. Biol., 15, 401–411, https://doi.org/10.1046/j.1523-1739.2001.015002401.x, 2001.
Rodrigues, J., Gérard, A., Séré, G., Morel, J.-L., Guimont, S., Simonnot, M.-O., and Pons, M.-N.: Life cycle impacts of soil construction, an innovative approach to reclaim brownfields and produce nonedible biomass, J. Clean. Prod., 211, 36–43, https://doi.org/10.1016/j.jclepro.2018.11.152, 2019.
Rokia, S., Séré, G., Schwartz, C., Deeb, M., Fournier, F., Nehls, T., Damas, O., and Vidal-Beaudet, L.: Modelling agronomic properties of Technosols constructed with urban wastes, Waste Manage., 34, 2155–2162, https://doi.org/10.1016/j.wasman.2013.12.016, 2014.
Root, R. A.: Analysis of a Study of Lead Wheel Weight Deposition and Abrasion in New Jersey, Water Air Soil Poll., 226, 381–389, https://doi.org/10.1007/s11270-015-2646-5, 2015.
Rossignol, J.-P.: Caractérisation des sols reconstitués, Le sol, support de nos plantations [Characterization of reconstituted soils, The soil, support of our plantations], Livre blanc du colloque de l'UNEP, 2 Juin 1999, Paris, 36–39, 1999.
Ruby, M. V., Davis, A., and Nicholson, A.: In situ formation of lead phosphates in soils as a method to immobilize lead, Environ. Sci. Technol., 28, 646–654, https://doi.org/10.1021/es00053a018, 1994.
Sandström, U. G.: Green infrastructure planning in urban Sweden, Plan. Pract. Res., 17, 373–385, https://doi.org/10.1080/02697450216356, 2002.
Säumel, I., Kotsyuk, I., Hölscher, M., Lenkereit, C., Weber, F., and Kowarik, I.: How healthy is urban horticulture in high traffic areas? Trace metal concentrations in vegetable crops from plantings within inner city neighbourhoods in Berlin, Germany, Environ. Pollut., 165, 124–132, https://doi.org/10.1016/j.envpol.2012.02.019, 2012.
Scalenghe, R. and Marsan, F. A.: The anthropogenic sealing of soils in urban areas, Landsc. Urban Plan., 90, 1–10, https://doi.org/10.1016/j.landurbplan.2008.10.011, 2009.
Selbig, W. R. and Balster, N.: Evaluation of Turf-Grass and Prairie-Vegetated Rain Gardens in a Clay and Sand Soil, Madison, Wisconsin, Water Years 2004-08 (U.S. Geological Survey), Scientific Investigations Report 2010-5077, https://doi.org/10.3133/sir20105077, 2010.
Séré, G.: Fonctionnement et évolution pédogénétique de Technosols issus d'un procédé de construction de sol, Functioning and pedogenic evolution of Technosols to come from a process of soil construction, Ph.D. thesis, Institut National Polytechnique de Lorraine, France, 227 pp., 2007.
Séré, G., Schwartz, C., Ouvrard, S., Sauvage, C., Renat, J.-C., and Morel, J. L.: Soil construction: A step for ecological reclamation of derelict lands, J. Soils Sediments, 8, 130–136, https://doi.org/10.1065/jss2008.03.277, 2008.
Séré, G., Schwartz, C., Ouvrard, S., Renat, J.-C., Watteau, F., Villemin, G., and Morel, J. L.: Early pedogenic evolution of constructed Technosols, J. Soils Sediments, 10, 1246–1254, https://doi.org/10.1007/s11368-010-0206-6, 2010.
Shchepeleva, A. S., Vasenev, V. I., Mazirov, I. M., Vasenev, I. I., Prokhorov, I. S., and Gosse, D. D.: Changes of soil organic carbon stocks and CO2 emissions at the early stages of urban turf grasses' development, Urban Ecosyst., 20, 309–321, https://doi.org/10.1007/s11252-016-0594-5, 2017.
Shchepeleva, A. S., Vizirskaya, M. M., Vasenev, V. I., and Vasenev, I. I.: Analysis of carbon stocks and fluxes of urban lawn ecosystems in Moscow megapolis, Springer Geography, 80–88, https://doi.org/10.1007/978-3-319-89602-1_11, 2019.
Shochat, E., Warren, P. S., Faeth, S. H., McIntyre, N. E., and Hope, D.: From patterns to emerging processes in mechanistic urban ecology, Trends Ecol. Evol., 21, 186–191, https://doi.org/10.1016/j.tree.2005.11.019, 2006.
Sipter, E., Rózsa, E., Gruiz, K., Tátrai, E., and Morvai, V.: Site-specific risk assessment in contaminated vegetable gardens, Chemosphere, 71, 1301–1307, https://doi.org/10.1016/j.chemosphere.2007.11.039, 2008.
Slukovskaya, M. V., Vasenev, V. I., Ivashchenko, K. V., Morev, D. V., Drogobuzhskaya, S. V., Ivanova, L. A., and Kremenetskaya, I. P.: Technosols on mining wastes in the subarctic: Efficiency of remediation under Cu-Ni atmospheric pollution, Int. Soil Water Conserv. Res., 7, 297–307, https://doi.org/10.1016/j.iswcr.2019.04.002, 2019.
Smagin, A. V.: Theory and practice of soil engineering, Moscow State University Press, Moscow, 544 pp., 2012 (in Russian).
Smagin, A. V. and Sadovnikova, N. B.: Creation of soil-like constructions, Eurasian Soil Sci., 48, 981–990, https://doi.org/10.1134/S1064229315090100, 2015.
Specht, K., Siebert, R., Hartmann, I., Freisinger, U. B., Sawicka, M., Werner, A., Thomaier, S., Henckel, D., Walk, H., and Dierich, A.: Urban agriculture of the future: an overview of sustainability aspects of food production in and on buildings, Agr. Hum. Values, 31, 33–51, https://doi.org/10.1007/s10460-013-9448-4, 2014.
Spliethoff, H. M., Mitchell, R. G., Shayler, H., Marquez-Bravo, L. G., Russell-Anelli, J., Ferenz, G., and McBride, M.: Estimated lead (Pb) exposures for a population of urban community gardeners, Environ. Geochem. Hlth., 38, 955–971, https://doi.org/10.1007/s10653-016-9790-8, 2016.
Suding, K. N., Gross, K. L., and Houseman, G. R.: Alternative states and positive feedbacks in restoration ecology, Trends Ecol. Evol., 19, 46–53, https://doi.org/10.1016/j.tree.2003.10.005, 2004.
Swedo, B. L., Glinka, C., Rollo, D. R., and Reynolds, H. L.: Soil bacterial community structure under exotic versus native understory forbs in a woodland remnant in Indiana, Proc. Indiana Acad. Sci., 117, 7–15, 2008.
Tan, P. Y., Wang, J., and Sia, A.: Perspectives on five decades of the urban greening of Singapore, Cities, 32, 24–32, https://doi.org/10.1016/j.cities.2013.02.001, 2013.
Thomsen, P., Bühler, O., and Kristoffersen, P.: Diversity of street tree populations in larger Danish municipalities, Urban For. Urban Gree., 15, 200–210, https://doi.org/10.1016/j.ufug.2015.12.006, 2016.
Tzoulas, K., Korpela, K., Venn, S., Yli-Pelkonen, V., Kaźmierczak, A., Niemela, J., and James, P.: Promoting ecosystem and human health in urban areas using Green Infrastructure: A literature review, Landsc. Urban Plan., 81, 167–178, https://doi.org/10.1016/j.landurbplan.2007.02.001, 2007.
US EPA: Brownfields Definition, US EPA, Brownfields Homepage, available at: https://www.epa.gov/brownfields/overview-epas-brownfields-program (last access: 4 September 2020), 1997.
Vasenev, V.: Analysis of microbial respiration and carbon pools for functional-ecological assessment of constructed Technosols in the Moscow region, Ph.D. thesis, Soil Science Faculty, Lomonosov Moscow State University, 2011.
Vasenev, V. I., Smagin, A. V., Ananyeva, N. D., Ivashchenko, K. V., Gavrilenko, E. G., Prokofeva, T. V., Paltseva, A., Stoorvogel, J. J., Gosse, D. D., and Valentini, R.: Urban soil's functions: Monitoring, assessment, and management, Adaptive Soil Management: From Theory to Practices, Springer, Singapore, 359–409, https://doi.org/10.1007/978-981-10-3638-5_18, 2017.
Vergnes, A., Le Viol, I., and Clergeau, P.: Green corridors in urban landscapes affect the arthropod communities of domestic gardens, Biol. Conserv., 145, 171–178, https://doi.org/10.1016/j.biocon.2011.11.002, 2012.
Vergnes, A., Blouin, M., Muratet, A., Lerch, T. Z., Mendez-Millan, M., Rouelle-Castrec, M., and Dubs, F.: Initial conditions during Technosol implementation shape earthworms and ants diversity, Landsc. Urban Plan., 159, 32–41, https://doi.org/10.1016/j.landurbplan.2016.10.002, 2017.
Vetterlein, D. and Hüttl, R. F.: Can applied organic matter fulfil similar functions as soil organic matter? Risk-benefit analysis for organic matter application as a potential strategy for rehabilitation of disturbed ecosystems, Plant Soil, 213, 1–10, https://doi.org/10.1023/A:1004681506901, 1999.
Vidal-Beaudet, L., Forget-Caubel, V., and Grosbellet, C.: Favour street tree root growth with high supplies of organic matter induces changes in urban soil properties, Acta Hortic., 1099, 943–950, https://doi.org/10.17660/ActaHortic.2015.1099.120, 2015.
Vidal-Beaudet, L., Rokia, S., Nehls, T., and Schwartz, C.: Aggregation and availability of phosphorus in a Technosol constructed from urban wastes, J. Soils Sediments, 18, 456–466, https://doi.org/10.1007/s11368-016-1469-3, 2016.
Vijayaraghavan, K.: Green roofs: A critical review on the role of components, benefits, limitations and trends, Renew. Sust. Energy Rev., 57, 740–752, https://doi.org/10.1016/j.rser.2015.12.119, 2016.
Villar, M. C., González-Prieto, S. J., and Carballas, T.: Evaluation of three organic wastes for reclaiming burnt soils: Improvement in the recovery of vegetation cover and soil fertility in pot experiments, Biol. Fert. Soils, 26, 122–129, https://doi.org/10.1007/s003740050354, 1997.
Walsh, D., Glass, K., Morris, S., Zhang, H., McRae, I., Anderson, N., Alfieri, A., Egendorf, S. P., Holberton, S., Owrang, S., and Cheng, Z.: Sediment exchange to mitigate pollutant exposure in urban soil, J. Environ. Manage., 214, 354–361, https://doi.org/10.1016/j.jenvman.2018.03.013, 2018.
Walsh, D., McRae, I., Zirngibl, R., Chawla, S., Zhang, H., Alfieri, A., Moore, H., Bailey, C., Brooks, A., Ostock, T., Pong, S., Hard, T., Sullivan, C., and Wilding, J.: Generation rate and fate of surplus soil extracted in New York City, Sci. Total Environ., 650, 3093–3100, https://doi.org/10.1016/j.scitotenv.2018.09.284, 2019.
Wilson, D. C. and Velis, C. A.: Waste management – still a global challenge in the 21st century: An evidence-based call for action, Waste Manage. Res., 33, 1049–1051, https://doi.org/10.1177/0734242X15616055, 2015.
Yilmaz, D., Sabre, M., Lassabatère, L., Dal, M., and Rodriguez, F.: Storm water retention and actual evapotranspiration performances of experimental green roofs in French oceanic climate, Eur. J. Environ. Civ. En., 20, 344–362, https://doi.org/10.1080/19648189.2015.1036128, 2016.
Yilmaz, D., Cannavo, P., Séré, G., Vidal-Beaudet, L., Legret, M., Damas, O., and Peyneau, P.-E.: Physical properties of structural soils containing waste materials to achieve urban greening, J. Soils Sediments, 18, 442–455, https://doi.org/10.1007/s11368-016-1524-0, 2018.
Zalasiewicz, J., Williams, M., Smith, A., Barry, T. L., Coe, A. L., Bown, P. R., Brenchley, P., Cantrill, D., Gale, A., Gibbard, P., Gregory, F. J., Hounslow, M. W., Kerr, A. C., Pearson, P., Knox, R., Powell, J. R., Waters, C., Marshall, J. K., Oates, M., Rawson, P., and Stone, P.: Are we now living in the Anthropocene, GSA Today, 18, 4–8, https://doi.org/10.1130/GSAT01802A.1, 2008.
Zhao, J., Chen, S., Jiang, B., Ren, Y., Wang, H., Vause, J., and Yu, H.: Temporal trend of green space coverage in China and its relationship with urbanization over the last two decades, Sci. Total Environ., 442, 455–465, https://doi.org/10.1016/j.scitotenv.2012.10.014, 2013.
Zhou, X. and Wang, Y.-C.: Spatial–temporal dynamics of urban green space in response to rapid urbanization and greening policies, Landsc. Urban Plan., 100, 268–277, https://doi.org/10.1016/j.landurbplan.2010.12.013, 2011.