the Creative Commons Attribution 4.0 License.
the Creative Commons Attribution 4.0 License.
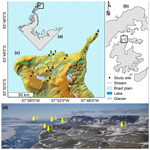
Soil organic matter interactions along the elevation gradient of the James Ross Island (Antarctica)
Vítězslav Vlček
David Juřička
Martin Valtera
Helena Dvořáčková
Vojtěch Štulc
Michaela Bednaříková
Jana Šimečková
Peter Váczi
Miroslav Pohanka
Pavel Kapler
Miloš Barták
Vojtěch Enev
Around half of the Earth's soil organic carbon (SOC) is presently stored in the Northern Hemisphere permafrost region. In polar permafrost regions, low temperatures particularly inhibit both the production and biodegradation of organic matter. Under such conditions, abiotic factors such as mesoclimate, pedogenic substrate or altitude are thought to be more important for soil development than biological factors. In Antarctica, biological factors are generally underestimated in soil development due to the rare occurrence of higher plants and the short time since deglaciation. In this study, we aim to assess the relationship between SOC and other soil properties related to the pedogenic factors or properties. Nine plots were investigated along the altitudinal gradient from 10 to 320 m in the deglaciated area of James Ross Island (Ulu Peninsula) using a parallel tea-bag decomposition experiment. SOC contents showed a positive correlation with the content of easily extractable glomalin-related soil protein (EE-GRSP; Spearman r=0.733, P=0.031) and the soil buffering capacity (expressed as ΔpH; Spearman r=0.817, P=0.011). The soil-available P was negatively correlated with altitude (Spearman , P=0.032), and the exchangeable Mg was negatively correlated with the rock fragment content (Spearman , P=0.050). No correlation was found between the available mineral nutrients (P, K, Ca and Mg) and SOC or GRSP. This may be a consequence of the inhibition of biologically mediated nutrient cycling in the soil. Therefore, the main factor influencing nutrient availability in these soils does not seem to the biotic environment; rather, the main impact appears to stem from the abiotic environment influencing the mesoclimate (altitude) or the level of weathering (rock content). Incubation in tea bags for 45 d resulted in the consumption and translocation of more labile polyphenolic and water-extractable organic matter, along with changes in the C content (increase of up to +0.53 % or decrease of up to −1.31 % C) and a decrease in the C:N ratio (from 12.5 to 7.1–10.2), probably due to microbial respiration and an increase in the abundance of nitrogen-binding microorganisms. Our findings suggest that one of the main variables influencing the SOC/GRSP content is not the altitude or coarse-fraction content (for which a correlation with SOC/GRSP was not found); rather, we suspect effects from other factors that are difficult to quantify, such as the availability of liquid water.
- Article
(3132 KB) - Full-text XML
-
Supplement
(3727 KB) - BibTeX
- EndNote
Oceans and underground fossil resources are the two largest carbon “pools” within the global carbon cycle, while topsoil, with an estimated store of more than 2000 petagrams of sequestered carbon (Pg C), is considered the third-greatest carbon sink. Most of this carbon (2200 Pg C) is in the form of soil organic matter (SOM), with a limited amount in the form of carbonates (Batjes, 1996). In addition, a further 600 Pg C is stored in the form of vegetation, while ca. 780 Pg C is stored in the atmosphere (Rackley, 2017). The oceans, fossil sources and the atmosphere contain vast stores of carbon, and soil, particularly soil organic matter (SOM), plays a crucial role in the carbon cycle. Soil carbon dynamics are mainly influenced by climatic factors and soil properties, which determine both the stability and decomposition rate of SOM (Aerts, 1997). Overall, the annual level of soil carbon flux, i.e. the amount of carbon emitted from the soil to the atmosphere due to microorganisms and root respiration, is estimated to be between 70 and 100 Pg C (Bond-Lamberty and Thomson, 2010). An important factor in this global carbon cycle flux is SOM mineralisation, which contributes ca. 58 Pg C annually to the total (Zech et al., 1997; Houghton, 2007). At the local scale, other factors will also play a role in the cycling of carbon, such as the nature of the organic matter, the local topography, and the composition of local vegetation and the microbial community (Makkonen et al., 2012; Bonanomi et al., 2013). Most of these factors, however, are directly or indirectly related to (micro)climatic conditions (Röder et al., 2016; Wilcke et al., 2008).
Currently, extensive research is being undertaken on the impact of climate change on terrestrial ecosystems, with permafrost areas seen as amongst the most vulnerable (Masson-Delmotte et al., 2006; Durán et al., 2021; Swati et al., 2021). The cold conditions prevalent at high latitudes are assumed to stunt the biological decomposition of organic matter, leading to the formation of soils rich in organic matter. This is especially true in Northern Hemisphere permafrost regions, where it is estimated that around half of the Earth's soil organic carbon (SOC) is presently stored (Tarnocai et al., 2009). Under Antarctic conditions, however, soil formation processes are thought to be affected more by climate, soil substrate and topography (e.g. altitude) than biological factors, due to both a general absence of higher vegetation and the relatively short period for pedogenesis (soil formation) since deglaciation. Consequently, soils in the Antarctic region tend to have a very low SOM content (Vlček et al., 2018; Pospíšilová et al., 2017; Zvěřina et al., 2012), with the rare occurrence of organic horizons (Campbell and Claridge, 1987).
Glomalin, or more commonly “glomalin-related soil protein” (GRSP; a crude extract containing many substances, including humic acids), is a hypothetical glycoprotein produced abundantly on the hyphae and spores of arbuscular mycorrhizal fungi (AMF; Glomeromycota) in the soil and roots and, as such, is an important component in SOM (Schüßler et al., 2001). Although there is some debate over its origin (e.g. see Gillespie et al., 2011; Holátko et al., 2021), GRSP is thought to be a sequential homologue of the chaperone protein group, involved in the folding of primary proteins, widespread across all domains of life, after their translation on ribosomes (Alaei et al., 2021). Arbuscular mycorrhiza is the most widespread form of mutualism between photobionts and fungi, represented in 70 %–90 % of vascular plant species (Basu et al., 2018; Błaszkowski, 2012; Fitter et al., 2008; Smith and Read, 2008). Fossil records indicate that arbuscular mycorrhiza has played an important role in terrestrial ecosystems for as long as 250–400 Myr (Harper et al., 2013; Redecker et al., 2000; Remy et al., 1994), with examples present in the earliest stages of the plant world's colonisation of terrestrial ecosystems (Blackwell, 2000; Pirozynski and Malloch, 1975; Simon et al., 1993).
The main factors determining the presence and growth of AMF appear to be soil properties and vegetation composition (Melo et al., 2019). Despite the extreme conditions found in Antarctica, non-lichenised fungi are found in the soil (Bridge and Newsham, 2009; Arenz et al., 2014) alongside lichen, which can produce allelochemicals that affect AMF (Martins et al., 2010; Tigre et al., 2012) by influencing mycorrhizal associations and acting as mutualism stimulators (Santiágo et al., 2018). Furthermore, some organic compounds produced by lichens, such as usnic and perlatolic acid, are also important carbon sources for the soil microbial community (Stark and Hyvärinen, 2003). Thus, it can be assumed that lichens and fungi are ecosystem drivers, especially in polar regions with few other organic compound producers. Alongside the fungi and lichens, mosses, the simplest terrestrial plants, also act as photobionts, thereby playing an important role in SOC dynamics and nutrient cycling, as well as maintaining soil structure, stability and water retention (Lovato et al., 1995; Smith and Read, 1997). Numerous previous studies have confirmed symbiotic relationships between AMF and mosses (e.g. Rayner, 2018; Kelley, 1950; Russell and Bulman, 2005).
In recent years, the Antarctic (Trinity) Peninsula has been undergoing unprecedented deglaciation due to significant local warming, which has been reported to be the most rapid on the planet over the last 50 years (Turner et al., 2005). From this perspective, the Antarctic Peninsula region represents a unique research location for early monitoring of the future development of soils in polar regions.
The main objectives of this study are as follows: (i) to determine the relationship between GRSP, SOC, SOM and environmental conditions (e.g. altitude, coarse-soil-fraction content and availability of liquid water); (ii) to assess the influence of environmental conditions on GRSP production and SOC content; and (iii) to monitor the initial stages of decomposition of a “standardised organic matter sample” (tea bag) using thermogravimetric analysis (TGA). With respect to aims (i) and (ii), we hypothesise that the highest GRSP and SOC content (and the lowest coarse-fraction content) will be found in soils located at the lowest elevations (H1). We further hypothesise that the GRSP content will be negatively correlated with the content of exchangeable phosphorous (P) (H2) and that SOC will be positively correlated with the GRSP content (H3). Regarding aim (iii), we hypothesise that a more rapid organic matter (OM) decomposition rate will be associated with increased soil SOC and GRSP content and that this will be indicative of environments with increased microbial activity (H4). Our results will contribute to improving our understanding of the processes underlying carbon deposition in (sub-)polar Antarctic areas under ongoing climate change.
2.1 Study area
In this study, we focus on soil formation processes near the Johann Gregor Mendel Czech Antarctic Station on James Ross Island, one of the largest islands off the north-eastern tip of the Antarctic Peninsula. The island has an area of ca. 2600 km2, 80 % of which is covered by permanent glaciers. We selected nine study sites on a 300 km2 deglaciated area on the Ulu Peninsula in the northern part of the island, an area covered with valleys and dome glaciers (Fig. 1). The climate is cold, with a 10-year mean annual temperature of −7.3 °C and −8 °C along the coastal area near the research station and at Bibby Hill (375 m a.s.l.) further inland (Matějka et al., 2021), respectively, with temperature inversions typically occurring during winter. According to Láska and Prošek (2013), elevation has little influence on temperature amplitude. Annual snow precipitation reaches 200 mm water equivalent (Strelin and Sone, 1998). Considering that the snow is subsequently redistributed by the wind, the occurrence of liquid water is more a matter of microrelief.
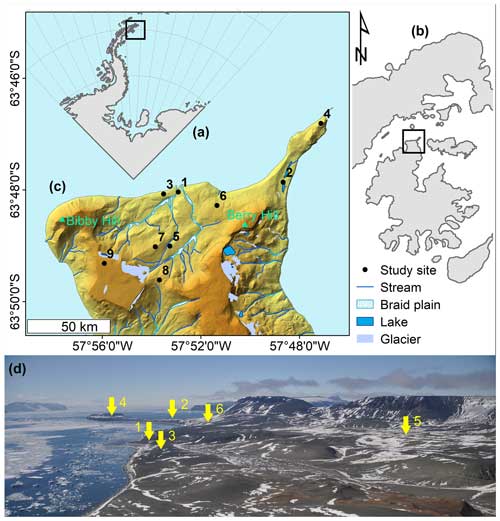
Figure 1Situational plan of (a) the northernmost tip of the Antarctic Peninsula, with (b) James Ross Island (zoomed in view of the square in panel a), (c) a digital terrain model (DTM) of the north coast of James Ross Island (Ulu Peninsula), and (d) a panoramic image of the map shown in panel (c) with study sites 1–6 indicated (yellow arrows; view from Bibby Hill). The map was created in ArcGIS Desktop v.10.8.1, the DTM and map layers were generated using the Czech Geological Survey ČGS 2009 dataset, and the situational plan was created in Inkscape 1.2.2. The photograph was sourced from an archive belonging to the first author.
2.2 Site description
The nine study sites were selected to cover a topographic gradient from lowest to highest elevation (Table 1). Site 1, known as the “Long-Term Research Plot” (see Barták et al., 2015), was located close to the Johann Gregor Mendel Czech Antarctic Station. The site consisted of two “plots”, one open to the elements (Plot 1a) and another in an open-topped enclosure (OTE; Plot 1b; Table 1). The land surface was covered by the marsh Bryum moss Bryum pseudotriquetrum Hedw., which formed thick carpets interspersed with patches of lichen, such as Rhizoplaca melanophthalma (DC.) Leuckert and Poelt and the elegant sunburst lichen Rusavskia elegans (Link) S.Y. Kondr. and Kärnefelt (Kondratyuk and Kärnefelt, 2003). The area was rich in microbial mats formed of Nostoc sp. colonies and cyanobacterial species (e.g. Microcoleus sp.), found in seepages. Site 2 was situated between the Big and Small Lachman lakes and consisted of two plots divided by soil moisture conditions (dryer Plot 2a and waterlogged Plot 2b). The vegetation here was dominated by the lichens R. elegans, Dermatocarpon polyphyllizum (Nyl.) Blomb. and Forssell, Hypnum revolutum (Mitt.) Lindb., Bryum argenteum Hedw. and Schistidium halinae Ochyra. The site had a liquid water supply during the short summer period, although this quickly froze as temperatures dropped. Site 3, situated near the research station, was also split into two, with one part close to a seal cadaver (Plot 3a) and the second further away in an unaffected area (Plot 3b); both sites had no vegetation cover. Site 4 was located on the side of the hill forming the Lachman Peninsula. Owing to its proximity to the shoreline and the annual availability of water from snowmelt, the site was classed as semi-moist to moist. Sites supplied with snowmelt water have a layer of vegetation comprised of mosses, such as H. revolutum, B. argenteum and S. halinae. Site 5 was located on a slope above a rivulet, dubbed “the Dirty Stream”, and had a surface covered with the mosses H. revolutum and B. argenteum. Owing to the annual availability of water from snowmelt from the western face of the Johnson Messa foothills, the site was classed as moderately moist. Site 6 was located at the foot of the northern slope of Berry Hill and received water from thawing snow fields on the hill. Mosses including H. revolutum, B. argenteum and S. halinae (Barták et al., 2015) were concentrated in the troughs of small periodic streams. Site 7 was located at the foot of the western slope of an un-named hill between “the Bohemian Stream” and “the Dirty Stream” and was classed as dry and without vegetation. Site 8 was located in the “Crame Col pass”, a flat plain between the Bibby Point massive and the Lachman Crags. Again, the site was classed as dry and without vegetation. Site 9 was located on the top of Berry Hill, with one sub-plot inside (Plot 9b) an OTE and one outside (Plot 9a). The land surface was comprised of early-developmental-stage organomineral substrate and was covered with patches of lichen (Usnea sphacelata and Umbilicaria decussata).
2.3 Soil properties
Composite soil samples (N=13), consisting of three individual samples arranged in a triangular pattern with a 5 m spacing, were collected from a depth of 0–5 cm at each site around the point where experiments on the decomposition of standardised organic matter were to be conducted. Soil samples were dried in an oven at 60 °C immediately after collection. The fraction smaller than 2 mm and the soil skeleton were subsequently separated. Only the fine-earth fraction was used for further analyses.
The soil reaction (pH) was determined in either distilled water or 1 M KCl at a ratio of 1:2.5 and measured on a 914 Metrohm pH/Conductometer. Available nutrients (P, K, Ca and Mg) were extracted using Mehlich III reagent (Mehlich, 1984) and then assessed using an Agilent 55B AA atomic absorption spectrometer (Agilent, CA, USA). All analyses were performed in triplicate. For a full description of the TGA and derivative thermogravimetric (DTG) analysis of soil (other elements – SOC and N), see Sect. 2.4.1. In this context, ΔpH refers to changes or differences in pH values (pH in water minus pH in KCl). The term ΔpH was used to indicate the difference in hydrogen ion concentration. However, a fixed ΔpH can have varying effects depending on the initial pH of the water. Therefore, it is recommended to calculate the corresponding change in the hydrogen ion concentration (Δ[H+]) in addition to ΔpH to fully understand the chemical implications of pH differences (Fassbender et al., 2021).
Glomalin (GRSP) determination
Easily extractable glomalin-related soil protein (EE-GRSP) was extracted from soil according to the procedure of Wright and Upadhyaya (1996). Briefly, 1 g of soil sample was added to 8 mL of 20 mM trisodium citrate solution (pH 7.0). The mixture was homogenised for 30 min on a GFL 3015 orbital shaker (Gesellschaft für Labortechnik mbH, Germany). The sample was then autoclaved for 60 min at 121 °C and, after cooling, was immediately centrifuged for 30 min at 3900 rpm on an MPW-223e centrifuge (MPW Med. Instruments, Poland). The supernatant was then frozen at −22 °C for later analysis using the method of Bradford (1976). Measurements were made in triplicate for each sample. Total glomalin (GRSP) was processed in the same manner, but instead of a 20 mM trisodium citrate solution, a 50 mM solution (pH 8.0) was used. All analyses were performed in triplicate.
2.4 Standardised organic matter (tea-bag) decomposition experiment
Two four-sided tetrahedral nylon bags containing 2 g (dry weight) of green tea (89 % Lipton Unilever green tea, EAN: 8714100770542; Keuskamp et al., 2013) were placed into pits 5 cm below the surface at each site during the 2019 summer season and then recovered with soil. The bags had a mesh size of 0.25 mm, allowing microorganisms to enter the bags (Keuskamp et al., 2013). After 45 d of exposure to the active permafrost layer, the tea bags were removed and then dried at 60 °C, reweighed, and transported back to the Czech Republic for further analysis. Note that, although tea bags were buried at all 13 sites, only half of the samples (7 of 13) were ultimately analysed as (i) some samples were destroyed by periodic freeze–thaw cycles at wetter locations, despite the test taking place in the Austral summer, and (ii) some samples were taken or moved by the south polar skua (Stercorarius maccormicki H. Saunders).
2.4.1 Thermogravimetric analysis (TGA)
Analysis of the relative content of elements in organic matter from the soil and tea samples was carried out using TGA analysis. First, 0.8–1.1 mg of the tea and 8–11 mg of soil was weighed into tin capsules, which were then packaged and combusted at 980 °C using oxygen (O2) injection as the combustion medium and helium as the carrier of the products formed. The relative nitrogen (N), carbon (C) and hydrogen (H) contents were evaluated based on gas chromatography, determined using an EA3000 CHNS-O analyser (EuroVector, Pavia, Italy), with sulfanilamide as a standard reference for analyser calibration. The O2 content was calculated from the residual combustible mass, and the data obtained were then adjusted for the moisture and ash content of the samples. All analyses were performed in triplicate. Sulfur (S) content proved to be below the detection limit (0.5 wt %) for all samples analysed.
Thermogravimetry (TG) was used to assess changes in the mass of the sample over time dependent on changes of temperature. For the soil samples, 5–15 mg of the sample was weighed onto a platinum sample pan and placed into a TA Q50 thermogravimetric analyser (TA Instruments, New Castle, DE, USA). The residual mass of the sample was then continuously recorded (±0.1 mass accuracy) as the sample was heated from ambient temperature to 950 °C at 10 °C min−1 in an oxidative atmosphere (air) or inert N2 atmosphere (both with a 50 mL min−1 flow rate), inducing a process of thermal degradation under pyrolysis. All measured thermograms were evaluated using the TA Universal Analysis 2000 software, in which the derivation of the TG curves was also performed. DTG analysis of the tea samples was undertaken in the same manner as the soil samples but using a TA Q5000 thermogravimetric analyser (TA Instruments, New Castle, DE, USA). Prior to measurement, all samples were homogenised by grinding them in an agate mortar. For both soil and tea, the moisture content was determined from the relative weight loss corresponding to the minimum DTG curve, with the residual mass at 950 °C representing the ash content (inorganic moieties). The SOM content was calculated according to the following relationship: . The data were obtained by thermogravimetric analysis using the oxidative atmosphere.
2.4.2 UV–Vis spectrophotometry
UV–Vis spectrophotometry was used to measure polyphenolic and water-extractable compounds from organic matter in tea samples, according to the modified Folin–Ciocalteho method of Gao et al. (2019), using a Hitachi U3600H dual-beam UV–Vis spectrometer (Hitachi, Tokyo, Japan) with a wavelength range of 650–850 nm and gallic acid as the calibration solution. The content of all water-extractable polyphenolic compounds was measured in triplicate for each sample.
2.5 Statistical analysis
The difference between potential and actual soil reaction (pH) was expressed as . The absolute difference between the potential and actual H+ concentration was calculated by taking the inverse logarithm of the pH values as follows: . Pairwise regression analysis was used to assess the significant difference between soil properties and selected environmental covariates in R v.4.2.2 (R Core Team, 2022). All regression models were considered statistically significant at p<0.05.
3.1 Soil nutrient content
Analysis of the nutrient content at each site revealed very low levels of exchangeable phosphorus (P), ranging from 0.0051 to 0.037 mg g−1; highly variable calcium (Ca) levels, ranging from 2.75 to 11.64 mg g−1; and magnesium (Mg) and potassium (K) levels ranging from 0.65 to 2.30 mg g−1 and from 3.57 to 2.20 mg g−1, respectively (Table 2). The pHwater and pHKCl values ranged from 6.48 to 8.17 (from slightly acidic to alkaline) and from 5.53 to 6.67 (from acidic to neutral), respectively (Table 2). The storage acidity (ΔpH) ranged from −0.74 to −1.79, the difference in hydrogen ion concentration (Δ[H+]) ranged from 10(−5.57) to 10(−6.71) and the coarse-fraction (>2.00 mm) content ranged from 3.8 % to 64.4 % of mass (Table 2).
Table 2Analysis of the available nutrient (P – phosphorous, K – potassium, Ca – calcium and Mg – magnesium; according to Mehlich, 1984) content at each study site, along with the coarse-fraction percentage. Site moisture conditions are denoted as follows: w (wet) – liquid water throughout the vegetation period, sd (semi-dry) – variable dry periods and d – dry site. pH values are expressed as follows: pHwater represents the pH in distilled deionised water, pHKCl represents the pH in potassium chloride solution, ΔpH represents the pH difference between pHwater and pHKCl, and inverse logarithm Δ[H+] represents changes in the hydrogen ion concentration.
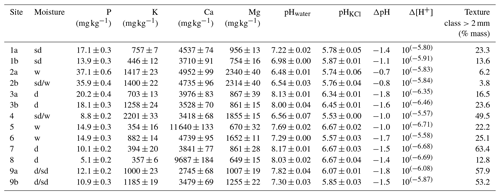
3.2 Relationship between soil organic-related fractions and environmental conditions
GRSP and EE-GRSP contents were generally low, ranging from 0.096 to 0.968 mg g−1 and from 0.072 to 0.752 mg g−1, respectively (Table 3). SOM content ranged from 2.79 to 15.04 wt %, while the SOC content ranged from 0.09 to 9.89 wt %, although, with few exceptions, most values were ≤1.5 wt % (Table 3).
Table 3Soil properties analysed via thermogravimetry. Soil organic-related fraction, showing glomalin fractions (EE-GRSP – easily extractable glomalin-related soil protein; GRSP – glomalin-related soil protein), soil organic matter (SOM), soil organic carbon (SOC) and total nitrogen (N; mean ± SE), and mass loss (wt %) during thermogravimetry over the following three temperature ranges: T1=200–430 °C, T2=430–590 °C and T3=590–750 °C.
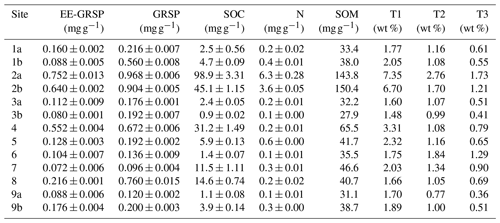
We recorded a very strong positive correlation between SOC and SOM (+0.93), a very strong positive correlation between GRSP and EE-GRSP (+0.91), and a strong correlation between EE-GRSP and SOC (+0.73). On the other hand, we recorded strong negative correlations between the coarse-fraction content and EE-GRSP (−0.67) and GRSP (−0.80), respectively, and between altitude and exchangeable P content (−0.71) (Fig. 2).
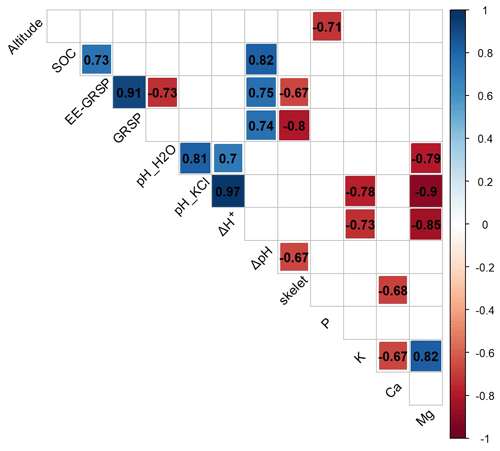
Figure 2Correlation diagram for selected environmental variables (altitude) and physical (skelet – coarse-fraction content), chemical (SOC – soil organic carbon, EE-GRSP – easily extractable glomalin-related soil protein and GRSP – glomalin-related soil protein) and physicochemical (pH, exchangeable ion: K – potassium, Ca – calcium, Mg – magnesium and P – phosphorus contents) properties in Antarctic topsoil. Only significant correlations at P<0.05 are shown.
3.3 Thermogravimetric and derivative thermogravimetric analysis of soil samples
The highest levels of soil loss (1.48–7.35 wt %) were recorded in the lowest temperature range from 200 to 430 °C, indicating that the majority of SOM fractions were relatively labile (Table 3). The mass loss in this temperature range was strongly correlated with both SOM (0.87) and SOC (0.73) content (Fig. 2). Relatively high mass loss (0.77–2.76 wt %) was also recorded at 430–590 °C, a range typically associated with the loss of mineral fractions (i.e. clays) and organic matter associated with the clay fraction. The lower levels of mass loss recorded at 590–750 °C (0.36–1.73 wt %) are typically representative of the least labile carbonates. Unexpectedly, the highest mass loss at Site 6 was recorded at 430–590 °C (1.84 wt %), where the N and SOM content values are 0.1 and 1.4 wt %, respectively (Table 3).
3.4 Thermogravimetric and derivative thermogravimetric analysis of standardised organic matter (tea) before and after decomposition
For all samples, DTG analyses of tea before decomposition showed a gradual decrease in mass as temperatures increased (Figs. 3 and 4), with the most rapid loss coinciding with three peaks at 204, 300 and 350 °C, indicating a loss of volatile compounds, hemicellulose and cellulose, respectively (Fig. 3). Peak mass loss during pyrolysis occurred at 300 °C, with a lesser peak occurring at 350 °C, although there was considerable overlap in the two peaks.
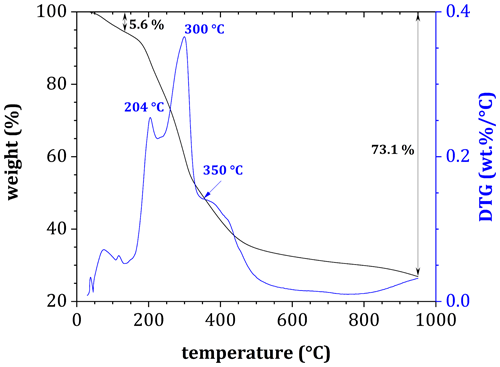
Figure 3Derivative thermogravimetric curve of a tea sample (before decomposition) during pyrolysis in an N2 atmosphere.
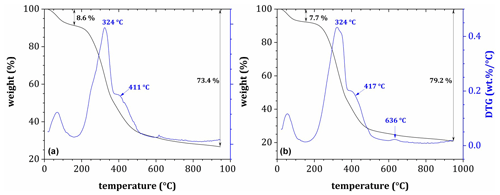
Figure 4Post-decomposition tea samples' derivative thermogravimetric curves (45 d exposure) during pyrolysis in an N2 atmosphere for (a) Site 3a (seal cadaver) and (b) Site 6.
DTG plots for post-decomposition samples (Fig. 4) showed two main mass loss peaks at 320–351 °C and 396–416 °C. In all cases, the first peak found in pre-decomposition samples around 204 °C was either missing or much less pronounced. On the other hand, a less pronounced peak appeared sporadically in post-decomposition samples over the range from 617 to 648 °C, which could be assigned to the highly stable substances such as lignin residues or humic substances in SOM. As such, post-decomposition OM showed higher thermal stability than the original samples, with the second and third mass loss peaks shifted slightly toward higher temperatures.
While N content increased at all sites post-decomposition (up to +2.6 wt %), C content remained relatively stable, with only a slight increase or decrease (−1.01 % to +1.3 %). As a result, the C:N ratio decreased from 12.5 to 7.1–10.2 post-decomposition, with the highest values recorded in the OTE at Site 9b. Overall, the C:N ratio tended to be lowest at lower-altitude sites and sites supplied with water (see Tables 1 and 4). In all cases, the abundance of polyphenolic and water-extractable compounds decreased post-decomposition, while ash content decreased slightly at OTE sites (Sites 1b, 9b) but increased elsewhere, with the greatest increase (+3.14 %) at Site 2. At all sites, the amount of OM decreased by 2 %–6 % post-decomposition (Table 4).
4.1 Interaction between environmental conditions and available nutrients in fine earth (hypotheses H1 and H2)
The lack of any correlation between available nutrients and OM in our results suggest that the main factor determining nutrient distribution in Antarctic deglaciated soils is not the presence of organic matter but rather environmental factors that accelerate mineral weathering processes. This is most likely due to the inhibition of biologically mediated nutrient cycling in the soil owing to extreme aridity and cold prevalent in Antarctica (McCraw, 1960; Campbell and Claridge, 1987). The important role of environmental factors, such as water availability and topography, is backed up by the positive correlation between available P and altitude (Fig. 2; thereby disproving Hypothesis H2). Unlike N, P lacks a biological fixation mechanism (Alewell et al., 2020). While sources of P are diverse, the primary source, particularly within Antarctic conditions, is via the weathering of apatite (Filippelli and Souch, 1999), i.e. the inorganic pool in which unweathered, mostly biologically unavailable material, typically dominates (Cross and Schlesinger, 2001). A second major source, particularly in coastal areas, could be seabird droppings; however, P values recorded in previous studies (e.g. Zhu et al., 2014) suggest that levels in seabird droppings are around 3 orders of magnitude lower than those recorded in this study. As such, the correlation between available P and altitude is more likely to be indicative of a decrease in weathering intensity along the altitudinal gradient, with the intensity of weathering affected by the presence of running liquid water.
We forecast that ongoing warming from global climate change observed since the second half of 20th century will lead to an expansion in soil biodiversity and vegetation cover along the Antarctic weathering gradient due to an increase in mineral weathering intensity as levels of liquid water increase (Turner et al., 2005). This will further strengthen the relationship between fungal and photoautotrophic organisms, i.e. AMF, whereby the fungi give plants access to mineral-weathered P and the plants provide products of photosynthesis (i.e. C) to fungi. According to Blackwell (2000) and Simon et al. (1993), this relationship has been in place since the earliest stages of terrestrial ecosystem colonisation.
4.2 Relationship between soil organic carbon and easily extractable glomalin-related soil protein (Hypothesis H3)
We further hypothesise that SOC will be positively correlated with GRSP content (H3). Under Antarctic conditions, the main sources of SOC are mosses (carbon = 40 %–50 %; Green et al., 2008; Vingiani et al., 2004), lichens (genus Usnea; carbon = 25 %; Shukla et al., 2019) and microorganisms associated with AMF (GRSP), with AMF hyphae containing around 50 % carbon (Zhu and Miller, 2003) and glomalin (associated with AMF) containing levels range from 28 % to 45 % (Huang et al., 2011). In our case, however, we believe that the main source of SOC was from glomalin (GRSP), as suggested by (i) the strong correlation (r=0.73) between EE-GRSP and SOC (thus confirming Hypothesis H3) and (ii) the relatively low (54 % on average) SOC in SOM (Table 3). In comparison, van Bemmelen (1889) reported a typical carbon content of 58 % and UN FAO (2019) reported a common carbon content of between 43 % and 58 % SOC in SOM. This would suggest that the absence of any relationship between the glomalin fraction (GRSP) and mass loss over the first temperature interval during TGA (where OM fraction mass loss is expected) could have been caused by the lower carbon content in both glomalin fractions, i.e. lower than the commonly reported 58 % C in OM (van Bemmelen, 1889).
The glomalin fraction content reported in this study (up to 0.97 mg g−1 GRSP; Table 2) was considerably higher than that reported in a previous study undertaken on James Ross Island, i.e. 0.01–0.15 mg g−1 (Pohanka and Vlček, 2018). This was most likely due to the study site in the prior work (Pohanka and Vlček, 2018) being situated close to the Whisky Glacier (Davis Dome), where the environmental conditions were unsuitable for vegetation. Surprisingly, the glomalin (GRSP) values in this study were actually higher than those recorded for agricultural soils, i.e. 0.30–0.70 mg g−1 (Wright and Anderson, 2000; Wuest et al., 2005). The high glomalin values (GRSP) recorded in our study were the result of the slow mineralisation of OM and its subsequent long-term accumulation.
Glomalin (GRSP) clearly plays an important role in Antarctic soil genesis, notably due to its influence on the buffering capacity. Correlations between ΔpH and SOC (0.82), ΔpH and GRSP (0.74), and ΔpH and EE-GRSP (0.75) all tend to indicate that fungi and their metabolites are contributing to the soil's buffering capacity, although the absence of any correlation between Δ[H+] and SOC and between EE-GRSP and GRSP does introduce some uncertainty.
As mentioned by Vlček et al. (2018), an SOC content of ≥2 % is not unusual in Antarctica; however, it should be noted that Vlček et al. (2018) measured SOC using the modified Walkley–Black method (Walkley and Black, 1934), which can underestimate SOC values. Walkley (1947), for example, stated that Walkley–Black recovery factors can range from 60 % to 86 %, while Grewal et al. (1991) reported recovery factors of 75 % to 85 %. In the present study, TGA and DTG values were comparable with those of Siewert (2004), who reported values ranging from 0.7 % to 3.2 % for 28 sites without vegetation on King George Island in the South Shetland Islands.
4.3 Changes in the decomposition of standardised organic matter indicated by thermogravimetric analysis (Hypothesis H4)
We hypothesise that a more rapid OM decomposition rate will be associated with increased soil SOC and GRSP content and that this will be indicative of environments with increased microbial activity (H4). As expected, OM decomposition was intrinsically linked with microbial activity (Fig. 4; Hypothesis H4). There was an apparent decrease in the abundance of polyphenolic and water-extractable compounds in the post-decomposition tea sample, indicating that the most thermally labile OM fractions (corresponding to a TGA peak at 204 °C in the original sample) had all been “lost” during decomposition. Furthermore, the partial overlap between the TGA and DTG curves in the incubated samples may indicate the formation of a complex organic compounds from labile fractions (which are characterised by a slightly bounded thermal stability).
Unexpectedly, we observed little change in total C post-decomposition, but we do see a noticeable decrease in the C:N ratio, suggesting that the tea sample had been colonised by N-fixing microorganisms (Utaile et al., 2021; Tóth et al., 2017; Kouchi et al., 2004). This could also help explain the relatively low C loss across sites, with any losses during mineralisation being compensated for by OM produced from microbial biomass (Paul, 2014; Keuskamp et al., 2013). This phenomenon is typical of the initial phase of mineralisation, where balance in the system has yet to be established (Golchin et al., 2018).
The study indicates that the nutrient distribution in Antarctic deglaciated soils is primarily influenced by environmental factors, such as topography, rather than the presence of OM. Altitude determines water availability, serving as the primary factor that accelerates the weathering of rock minerals. This was supported by the lack of any connections between available nutrients and OM, most likely resulting from the inhibition of biologically mediated nutrient cycling in the soil due to the extreme climate. Global warming is expected to enhance soil biodiversity and vegetation cover along the Antarctic weathering gradient by increasing the mineral weathering intensity and water availability. This study confirms a positive correlation between SOC and the GRSP content, identifying glomalin produced by microorganisms as a primary source of SOC in Antarctic soils. The higher GRSP content observed in this work suggests slow mineralisation and long-term accumulation of organic matter, highlighting glomalin's role in Antarctic soil development. Decomposition tests show a decrease in the most labile OM fractions (polyphenols and water-extractable compounds) and a shift in the C : N ratio, suggesting colonisation by nitrogen-fixing microorganisms, which helps balance carbon loss during the initial phases of mineralisation.
The source of thermogravimetry (TGA) data can be found in the Supplement below.
The supplement related to this article is available online at: https://doi.org/10.5194/soil-10-813-2024-supplement.
Conceptualisation was performed by VV, DJ and MV; methodology by VV and DJ; formal analysis by MV and HD; field investigation by MB, PV and MB; writing, including preparation of initial draft, by VV, DJ and MV; writing, including review and editing, by VV, JŠ, PV and VE; visualisation by VV and DJ; supervision by VV; and fundraising by MP. Laboratory analysis and evaluation were carried out as follows: thermogravimetric analysis by VE, glomalin by MP, and soil properties by VV and JŠ.
The contact author has declared that none of the authors has any competing interests.
Publisher's note: Copernicus Publications remains neutral with regard to jurisdictional claims made in the text, published maps, institutional affiliations, or any other geographical representation in this paper. While Copernicus Publications makes every effort to include appropriate place names, the final responsibility lies with the authors.
This article is part of the special issue “Earth surface shaping by biota (Esurf/BG/SOIL/ESD/ESSD inter-journal SI)”. It is not associated with a conference.
The authors would like to thank all of the members of the Masaryk University who worked on the Czech Antarctic Research Programme for data provision and for fieldwork support. This work was supported by the Ministry of Defence of the Czech Republic “Long Term Organization Development Plan 1011” – Healthcare Challenges of WMD II of the Military Faculty of Medicine Hradec Kralove, University of Defence, Czech Republic (project no. DZRO-FVZ22-ZHN II).
This paper was edited by Boris Jansen and reviewed by two anonymous referees.
Aerts, R.: Climate, leaf litter chemistry and leaf litter decomposition in terrestrial ecosystems: A triangular relationship, Oikos, 79, 439–449, https://doi.org/10.2307/3546886, 1997.
Alaei, L., Ashengroph, M., and Moosavi-Movahedi, A. A.: The concept of protein folding/unfolding and its impacts on human health, Adv. Protein Chem. Str., 126, 227–278, https://doi.org/10.1016/bs.apcsb.2021.01.007, 2021.
Alewell, C., Ringeval, B., Ballabio, C., Robinson, D. A., Panagos, P., and Borrelli, P.: Global phosphorus shortage will be aggravated by soil erosion, Nat. Commun., 11, 4546, https://doi.org/10.1038/s41467-020-18326-7, 2020.
Arenz, B. E., Blanchette, R. A., and Farrell, R. L.: Fungal diversity in Antarctic soils, in: Antarctic Terrestrial Microbiology, edited by: Cowan, D. A., Springer-Verlag, Berlin Heiderberg, 35–53, https://doi.org/10.1007/978-3-642-45213-0_3, 2014.
Barták, M., Váczi, P., Stachoň, Z., and Kubešová, S.: Vegetation mapping of moss-dominated areas of northern part of James Ross Island (Antarctica) and a suggestion of protective measures, Czech Polar Rep., 5, 75–87, https://doi.org/10.5817/CPR2015-1-8, 2015.
Basu, S., Rabara, R. C., and Negi, S.: AMF: The future prospect for sustainable agriculture, Physiol. Mol. Plant P., 102, 36–45, https://doi.org/10.1016/j.pmpp.2017.11.007, 2018.
Batjes, N. H.: Total carbon and nitrogen in the soils of the world, Eur. J. Soil Sci., 47, 151–163, https://doi.org/10.1111/j.1365-2389.1996.tb01386.x, 1996.
Blackwell, M.: Evolution. Terrestrial life – fungal from the start?, Science, 289, 1884–1885, https://doi.org/10.1126/science.289.5486.1884, 2000.
Błaszkowski, J.: Glomeromycota, W. Szafer Institute of Botany, Polish Academy of Sciences, Kraków, 303 pp., ISBN 978-83-89648-82-2, 2012.
Bonanomi, G., Incerti, G., Giannino, F., Mingo, A., Lanzotti, V., and Mazzoleni, S.: Litter quality assessed by solid state 13C NMR spectroscopy predicts decay rate better than and LigninN ratios, Soil Biol. Biochem., 56, 40–48, https://doi.org/10.1016/j.soilbio.2012.03.003, 2013.
Bond-Lamberty, B. and Thomson, A.: A global database of soil respiration data, Biogeosciences, 7, 1915–1926, https://doi.org/10.5194/bg-7-1915-2010, 2010.
Bradford, M. M.: A rapid and sensitive method for the quantitation of microgram quantities of protein utilizing the principle of protein-dye binding, Anal. Biochem., 72, 248–254, https://doi.org/10.1016/0003-2697(76)90527-3, 1976.
Bridge, P. D. and Newsham, K. K.: Soil fungal community composition at Mars Oasis, a southern maritime Antarctic site, assessed by PCR amplification and cloning, Fungal Ecol., 2, 66–74, https://doi.org/10.1016/j.funeco.2008.10.008, 2009.
Campbell, I. B. and Claridge, G. G. C.: Antarctica: Soils, Weathering Processes and Environment. Developments in Soil Science 16, Elsevier Science, 367 pp., ISBN 9780444427847, 1987.
Cross, A. F. and Schlesinger, W. H.: Biological and geochemical controls on phosphorus fractions in semiarid soils, Biogeochemistry, 52, 155–172, https://doi.org/10.1023/A:1006437504494, 2001.
Czech Geological Survey: James Ross Island – Northern Part. Topographic map 1 : 25 000, CGS, Praha, ISBN 978-80-7075-734-5, 2009.
Durán, J., Rodríguez, A., Heiðmarsson, S., Lehmann, J. R. K., del Moral, Á., Garrido-Benavent, I., and De los Ríos, A.: Cryptogamic cover determines soil attributes and functioning in polar terrestrial ecosystems, Sci. Total Environ., 762, 143169, https://doi.org/10.1016/j.scitotenv.2020.143169, 2021.
FAO: Measuring and modelling soil carbon stock and stock changes in livestock production systems. Guidelines for assessment (Version 1), Livestock Environmental Assessment and Performance (LEAP) Partnership, FAO, Rome, 170 pp., ISBN 978-92-5-131408-1, 2019.
Fassbender, A. J., Orr, J. C., and Dickson, A. G.: Technical note: Interpreting pH changes, Biogeosciences, 18, 1407–1415, https://doi.org/10.5194/bg-18-1407-2021, 2021.
Filippelli, G. M. and Souch, C.: Effects of climate and landscape development on the terrestrial phosphorus cycle, Geology, 27, 171–174, https://doi.org/10.1130/0091-7613(1999)027<0171:EOCALD>2.3.CO;2, 1999.
Fitter, A. H., Heinemeyer, A., and Staddon, P. L.: The impact of elevated CO2 and global climate change on arbuscular mycorrhizas: a mycocentric approach, New Phytol., 147, 179–187, https://doi.org/10.1046/j.1469-8137.2000.00680.x, 2008.
Gao, M. R., Xu, Q. D., He, Q., Sun, Q., and Zeng, W.-C.: A theoretical and experimental study: the influence of different standards on the determination of total phenol content in the Folin–Ciocalteu assay, J. Food Meas. Charact., 13, 1349–1356, https://doi.org/10.1007/s11694-019-00050-6, 2019.
Gillespie, A. W., Farrell, R. E., Walley, F. L., Ross, A. R., Leinweber, P., Eckhardt, K. U., Regier, T. Z., and Blyth, R. I.: Glomalin-related soil protein contains non-mycorrhizal-related heat-stable proteins, lipids and humic materials, Soil. Biol. Biochem., 43, 766–777, https://doi.org/10.1016/j.soilbio.2010.12.010, 2011.
Golchin, A., Baldock, J. A., and Oades, J. M.: A model linking organic matter decomposition, chemistry, and aggregate dynamics, in: Soil processes and the carbon cycle, edited by: Lal, R., Kimble, J. M., Follet, F., and Stewart, B. A., CRC Press, 245–266, https://doi.org/10.1201/9780203739273, 2018.
Green, T. G. A., Nash, T. H., and Lange, O. L.: Physiological ecology of carbon dioxide Exchange, in: Lichen Biology, edited by: Nash III, T. H., Cambridge University Press, 152–181, https://doi.org/10.1017/CBO9780511790478.010, 2008.
Grewal, K. S., Buchan, G. D., and Sherlock, R. R.: Comparison of three methods of organic carbon determination in some New Zealand soils, Eur. J. Soil Sci., 42, 251–257, https://doi.org/10.1111/j.1365-2389.1991.tb00406.x, 1991.
Harper, C. J., Taylor, T. N., Krings, M., and Taylor, E. L.: Mycorrhizal symbiosis in the Paleozoic seed fern Glossopteris from Antarctica, Rev. Palaeobot. Palyno., 192, 22–31, https://doi.org/10.1016/j.revpalbo.2013.01.002, 2013.
Holátko, J., Brtnický, M., Kučerík, J., Kotianová, M., Elbl, J., Kintl, A., Kynický, J., Benada, O., Datta, R., and Jansa, J.: Glomalin – Truths, myths, and the future of this elusive soil glycoprotein, Soil Biol. Biochem., 153, 108116, https://doi.org/10.1016/j.soilbio.2020.108116, 2021.
Houghton, R. A.: Balancing the global carbon budget, Annu. Rev. Earth Pl. Sc., 35, 313–347, https://doi.org/10.1146/annurev.earth.35.031306.140057, 2007.
Huang, Y., Wang, D. W., Cai, J. L., and Zheng, W. S.: Review of glomalin-related soil protein and its environmental function in the rhizosphere, Chin. J. Plant Ecol., 35, 232–236, https://www.plant-ecology.com/EN/10.3724/SP.J.1258.2011.00232 (last access: 14 November 2024), 2011.
Kelley, A. P.: Mycotrophy in plants. Chronica Botanica, Waltham, MA, 223 pp., ISBN-13 978-1121005679, 1950.
Keuskamp, J. A., Dingemans, B. J. J., Lehtinen, T., Sarneel, J. M., and Hefting, M. M.: Tea Bag Index: a novel approach to collect uniform decomposition data across ecosystems, Method. Ecol. Evol., 4, 1070–1075, https://doi.org/10.1111/2041-210X.12097, 2013.
Kondratyuk, S. Y. and Kärnefelt, I.: Revision of three natural groups of xanthorioid lichens (Teloschistaceae, Ascomycota), Ukrainskiy Botanichnyi Zhurnal, 60, 427–437, 2003.
Kouchi, H., Shimomura, K., Hata, S., Hirota, A., Wu, G. J., Kumagai, H., Tajima, S., Suganuma, N., Suzuki, A., Aoki, T., Hayashi, M., Yokoyama, T., Ohyama, T., Asamizu, E., Kuwata, Ch., Shibata, D., and Tabata, S.: Large-scale analysis of gene expression profiles during early stages of root nodule formation in a model legume, Lotus japonicus, DNA Res., 11, 263–274, https://doi.org/10.1093/dnares/11.4.263, 2004.
Láska, K. and Prošek, P.: Klima a klimatický výzkum ostrova James Ross, in: Antarktida, edited by: Prošek, P., Academia, 348 pp., ISBN 978-80-200-2140-3, 2013.
Lovato, P. E., Schuepp, H., Trouvelot, A., and Gianinazzi, S.: Application of arbuscular mycorrhizal fungi (AMF) in orchid and ornamental plants, in: Mycorrhiza, edited by: Varma, A. and Hock, B., Springer, Berlin Heidelberg New York, 443–468, https://doi.org/10.1007/978-3-662-03779-9_19, 1995.
Makkonen, M., Berg, M. P., Handa, I. T., Hättenschwiler, S., van Ruijven, J., van Bodegom, P. M., and Aerts, R.: Highly consistent effects of plant litter identity and functional traits on decomposition across a latitudinal gradient, Ecol. Lett., 15, 1033–1041, https://doi.org/10.1111/j.1461-0248.2012.01826.x, 2012.
Martins, M. C. B., de Lima, M. J. G., Silva, F. P., Azevedo-Ximenes, E., da Silva, N. H., and Pereira, E. C.: Cladia aggregata (lichen) from Brazilian Northeast: chemical characterization and antimicrobial activity, Braz. Arch. Biol. Techn., 53, 115–122, https://doi.org/10.1590/S1516-89132010000100015, 2010.
Masson-Delmotte, V., Kageyama, M., Braconnot, P., Charbit, S., Krinner, G., Ritz, C., Guilyardi, E., Jouzel, J., Abe-Ouchi, A., Crucifix, M., Gladstone, R. M., Hewitt, C. D., Kitoh, A., LeGrande, A. N., Marti, O., Merkel, U., Motoi, T., Ohgaito, R., Otto-Bliesner, B., Peltier, W. R., Ross, I., Valdes, P. J., Vettoretti, G., Weber, S. L., Wolk, F., and Yu, Y.: Past and future polar amplification of climate change: climate model intercomparisons and ice-core constraints, Clim. Dynam., 26, 513–529, https://doi.org/10.1007/s00382-005-0081-9, 2006.
Matějka, M., Láska, K., Jeklová, K., and Hošek, J.: High-Resolution Numerical Modelling of Near-Surface Atmospheric Fields in the Complex Terrain of James Ross Island, Antarctic Peninsula, Atmosphere-Basel, 12, 360, https://doi.org/10.3390/atmos12030360, 2021.
McCraw, J. D.: Soils of the Ross Dependency, Antarctica. A preliminary note, N. Z. Soc. Soil Sci. Proc., 4, 30–35, 1960.
Mehlich, A.: Mehlich 3 soil test extractant: A modification of the Mehlich 2 extractant, Commun. Soil Sci. Plan., 15, 1409–1416, https://doi.org/10.1080/00103628409367568, 1984.
Melo, C. D., Walker, C., Krüger, C., Borges, P. A. V., Luna, S., Mendoca, D., Fonseca, H. M. A. C., and Machado, A. C.: Environmental factors driving arbuscular mycorrhizal fungal communities associated with endemic woody plant Picconiaazorica on native forest of Azores, Ann. Microbiol., 69, 1309–1327, https://doi.org/10.1007/s13213-019-01535-x, 2019.
Mlčoch, B., Nývlt, D., and Mixa, P.: Geological map of James Ross Island Northern Part 1 : 25 000, Czech Geol. Survey, Prague, ISBN 978-80-7075-996-7, 2020.
Paul, E. (Ed.): Soil microbiology, ecology and biochemistry, Academic Press, 604 pp., ISBN 9780124159556, 2014.
Pirozynski, K. A. and Malloch, D. W.: The origin of land plants: a matter of mycotropism, Biosystems, 6, 153–164, https://doi.org/10.1016/0303-2647(75)90023-4, 1975.
Pohanka, M. and Vlček, V.: Assay of glomalin using a quartz crystal microbalance biosensor, Electroanalysis, 30, 453–458, https://doi.org/10.1002/elan.201700772, 2018.
Pospíšilová, L., Vlček, V., Hybler, V., and Uhlík, P.: Carbon content and macroelement in cryosols, Humic Substances Research, 13, 13–17, 2017.
R Core Team: R: A Language and Environment for Statistical Computing, R Foundation for Statistical Computing, Vienna, Austria, https://www.R-project.org/ (last access: 15 December 2022), 2022.
Rackley, S. A.: Carbon capture and storage, Butterworth-Heinemann, 698 pp., ISBN 9780128120415, 2017.
Rayner, M. C.: Mycorrhiza – An Account of Non-Pathogenic Infection by Fungi in Vascular Plants and Bryophytes, White Press, ISBN-10: 152870763X, ISBN-13: 978-1528707633, 2018.
Redecker, D., Kodner, R., and Graham, L. E.: Glomalean fungi from the Ordovician, Science, 289, 1920–1921, https://doi.org/10.1126/science.289.5486.1920, 2000.
Remy, W., Taylor, T. N., Hass, H., and Kerp, H.: Four hundred-million-year-old vesicular arbuscular mycorrhizae, P. Natl. Acad. Sci. USA, 91, 11841–11843, https://doi.org/10.1073/pnas.91.25.11841, 1994.
Röder, J., Detsch, F., Otte, I., Appelhans, T., Nauss, T., Peters, M. K., and Brandl, R.: Heterogeneous patterns of abundance of epigeic arthropod taxa along a major elevation gradient, Biotropica, 49, 217–228, https://doi.org/10.1111/btp.12403, 2016.
Russell, J. and Bulman, S.: The liverwort Marchantia foliacea forms a specialized symbiosis with arbuscular mycorrhizal fungi in the genus Glomus, New Phytol., 165, 567–579, https://doi.org/10.1111/j.1469-8137.2004.01251.x, 2005.
Santiago, R., Silva, N. H., Silva, F. P., Martins, M. C. B., de Vasconcelos, T. L., Yano-Melo, A. M., and Pereira, E. C.: Interactions of the lichen Cladonia salzmannii Nyl. with soil, microbiota, mycorrhizae and Genipa Americana, J. Soil Sci. Plant Nut., 18, 833–850, https://doi.org/10.4067/S0718-95162018005002402, 2018.
Schüßler, A., Schwarzott, D., and Walker, Ch.: A new fungal phylum, the Glomeromycota: phylogeny and evolution, Mycol. Res., 105, 1413–1421, https://doi.org/10.1017/S0953756201005196, 2001.
Shukla, V., Bajpai, R., Pandey, U., and Upreti, D. K.: Do Lichens have the Ability to Remove Arsenic from Water?, Int. J. Plant Environ., 5, 47–49, https://doi.org/10.18811/ijpen.v5i01.8, 2019.
Siewert, Ch.: Rapid screening of soil properties using thermogravimetry, Soil Sci. Soc. Am. J., 68, 1656–1661, https://doi.org/10.2136/sssaj2004.1656, 2004.
Simon, L., Bousquet, J., Levesque, R. C., and Lalonde, M.: Origin and diversification of endomycorrhizal fungi and coincidence with vascular land plants, Nature, 363, 67–69, https://doi.org/10.1038/363067a0, 1993.
Smith, S. E. and Read, D. J.: Mycorrhizal Symbiosis. San Diego, CA, Academic Press, https://doi.org/10.1016/B978-0-12-652840-4.X5000-1, 1997.
Smith, S. E. and Read, D. J.: Mycorrhizal Symbiosis, Academic Press, San Diego, USA, 800 pp., https://doi.org/10.1016/B978-0-12-370526-6.X5001-6, 2008.
Stark, S. and Hyvärinen, M.: Are phenolics leaching from the lichen Cladina stellaris sources of energy rather than allelophatic agents for soil microorganisms?, Soil Biol. Biochem., 35, 1381–1385, https://doi.org/10.1016/S0038-0717(03)00217-7, 2003.
Strelin, J. A. and Sone, T.: Rock glaciers on James Ross Island, Antarctica, in: Permafrost, 7th International Conference Proceedings, Yellowknife (Canada), edited by: Lewkowicz, A. G. and Allard, M., Collection Nordicana University Laval, Quebec, 1027–1032, 23–27 June 1998, OCLC No 40770716, ISBN: 9782920197572, 2920197576, 1998.
Swati, J., Bajpai, A., and Narain Johri, B.: Extremophilic fungi at the interface of climate change, in: Fungi Bio-Prospects in Sustainable Agriculture, Environment and Nano-technology, edited by: Kumar, V. S., Shah, M. P., Parmar, S., and Kumar, A., Academic Press, 1–22, ISBN 9780128219256, https://doi.org/10.1016/B978-0-12-821925-6.00001-0, 2021.
Tarnocai, C., Canadell, J. G., Schuur, E. A. G., Kuhry, P., Mazhitova, G., and Zimov, S.: Soil organic carbon pools in the northern circumpolar permafrost region, Global Biogeochem. Cy., 23, GB2023, https://doi.org/10.1029/2008GB003327, 2009.
Tigre, R. C., Silva, N. H., Santos, M. G., Honda, N. K., Falcao, E. P. S., and Pereira, E. C.: Allelopathic and bioherbicidal potential of Cladonia verticillaris on the germination and growth of Lactuca sativa, Ecotoxicol. Environ. Safety, 84, 125–132, https://doi.org/10.1016/j.ecoenv.2012.06.026, 2012.
Tóth, Z., Táncsics, A., Kriszt, B., Kröel-Dulay, G., Ónodi, G., and Hornung, E.: Extreme effects of drought on composition of the soil bacterial community and decomposition of plant tissue, Eur. J. Soil Sci., 68, 504–513, https://doi.org/10.1111/ejss.12429, 2017.
Turner, J., Colwell, S. R., Marshall, G. J., Lachlan-Cope, T. A., Carleton, A. M., Jones, P. D., Lagun, V., Reid, P. A., and Iagovkina, S.: Antarctic climate change during the last 50 years, Int. J. Climatol., 25, 279–294, https://doi.org/10.1002/joc.1130, 2005.
Utaile, Y. U., Honnay, O., Muys, B., Cheche, S. S., and Helsen, K.: Effect of Dichrostachys cinerea encroachment on plant species diversity, functional traits and litter decomposition in an East‐African savannah ecosystem, J. Veg. Sci., 32, e12949, https://doi.org/10.1111/jvs.12949, 2021.
van Bemmelen, J. M.: Über die Bestimmungen des Wassers, den Humus, des Schwefels, der in den colloidalen Silikaten gebunden Kieselsäuren, des Mangans, u.s.w. im Ackerboden, Landwirtschaftliche Versuch Station, 37, 279–290, 1889.
Vingiani, S., Adamo, P., and Giordano, S.: Sulphur, nitrogen and carbon content of Sphagnum capillifolium and Pseudevernia furfuracea exposed in bags in the Naples urban area, Environ. Pollut., 129, 145–158, https://doi.org/10.1016/j.envpol.2003.09.016, 2004.
Vlček, V., Pospíšilová, L., and Uhlík, P.: Mineralogy and chemical composition of Cryosols and Andosols in Antarctica, Soil Water Res., 13, 61–73, https://doi.org/10.17221/231/2016-SWR, 2018.
Walkley, A.: A Critical Examination of a Rapid Method for Determining Organic Carbon in Soils: Effect of Variations in Digestion Conditions and of Inorganic Soil Constituents, Soil Sci., 63, 251–264, https://doi.org/10.1097/00010694-194704000-00001, 1947.
Walkley, A. and Black, I. A.: An examination of the Degtjareff method for determining soil organic matter, and a proposed modification of the chromic acid titration method, Soil Sci., 37, 29–38, https://doi.org/10.1097/00010694-193401000-00003, 1934.
Wilcke, W., Oelmann, Y., Schmitt, A., Valarezo, C., Zech, W., and Homeier, J.: Soil properties and tree growth along an altitudinal transect in Ecuadorian tropical montane forest, J. Plant Nutr. Soil Sc., 171, 220–230, https://doi.org/10.1002/jpln.200625210, 2008.
Wright, S. F. and Anderson, R. L.: Aggregate stability and glomalin in alternative crop rotations for the central Great Plains, Biol. Fert. Soils, 31, 249–253, https://doi.org/10.1007/s003740050653, 2000.
Wright, S. F. and Upadhyaya, A.: Extraction of an abundant and unusual protein from soil and comparison with hyphal protein of arbuscular mycorrhizal fungi, Soil Sci., 161, 575–586, https://doi.org/10.1097/00010694-199609000-00003, 1996.
Wuest, S. B., Caesar-TonThat, T. C., Wright, S. F., and Williams, J. D.: Organic matter addition, N, and residue burning effects on infiltration, biological, and physical properties of an intensively tilled silt–loam soil, Soil Till. Res., 84, 154–167, https://doi.org/10.1016/j.still.2004.11.008, 2005.
Zech, W., Senesi, N., Guggenberger, G., Kaiser, K., Lehmann, J., Miano, T., Miltner, A., and Schroth, G.: Factors controlling humification and mineralization of soil organic matter in the tropics, Geoderma, 79, 117–161, https://doi.org/10.1016/S0016-7061(97)00040-2, 1997.
Zhu, R., Wang, Q., Wang, C., Hou, L., and Ma, D.: Penguins significantly increased phosphine formation and phosphorus contribution in maritime Antarctic soils, Sci. Rep.-UK, 4, 7055, https://doi.org/10.1038/srep07055, 2014.
Zhu, Y.-G. and Miller, R. M.: Carbon cycling by arbuscular mycorrhizal fungi in soil-plant systems, Trends Plant Sci., 8, 407–409, https://doi.org/10.1016/s1360-1385(03)00184-5, 2003.
Zvěřina, O., Coufalík, P., Vaculovič, T., Kuta, J., Zeman, J., and Komárek, J.: Macro-and microelements in soil profile of the moss-covered area in James Ross Island, Antarctica, Czech Polar Rep., 2, 1–7, https://doi.org/10.5817/CPR2012-1-1, 2012.