the Creative Commons Attribution 4.0 License.
the Creative Commons Attribution 4.0 License.
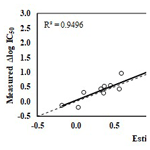
Increase in bacterial community induced tolerance to Cr in response to soil properties and Cr level in the soil
Claudia Campillo-Cora
Daniel Arenas-Lago
Manuel Arias-Estévez
David Fernández-Calviño
Chromium (Cr) soil pollution is a pressing global concern that demands thorough assessment. The pollution-induced community tolerance (PICT) methodology serves as a highly sensitive tool capable of directly assessing metal toxicity within microbial communities. In this study, 10 soils exhibiting a wide range of properties were subjected to Cr contamination, with concentrations ranging from 31.25 to 2000 mg Cr kg−1, in addition to the control. Bacterial growth, assessed using the [3H]-leucine incorporation technique, was used to determine whether bacterial communities developed tolerance to Cr, i.e. PICT to Cr in response to Cr additions to different soil types. The obtained results revealed that at concentrations of 1000 or 2000 mg Cr kg−1, certain bacterial communities showed inhibited growth, likely attributable to elevated Cr toxicity, while others continued to thrive. Interestingly, with Cr concentrations below 500 mg Cr kg−1, bacterial communities demonstrated two distinct responses depending on soil type: 7 of the 10 studied soils exhibited an increased bacterial community tolerance to Cr, while the remaining 3 soils did not develop such tolerance. Furthermore, the Cr level at which bacterial communities developed tolerance to Cr varies among soils, indicating varying levels of Cr toxicity between studied soils. The dissolved organic carbon (DOC) and the fraction of Cr extracted with distilled water (H2O-Cr) played an essential role in shaping the impact of Cr on microbial communities (R2=95.6 %). These factors (DOC and H2O-Cr) contribute to increased Cr toxicity in soil, i.e. during the selection phase of the PICT methodology.
- Article
(3143 KB) - Full-text XML
-
Supplement
(441 KB) - BibTeX
- EndNote
Chromium (Cr) is a highly toxic non-essential metal for microorganisms and plants that may naturally occur at high concentrations from parent materials, e.g. serpentine rocks (Adriano, 2001; Cervantes et al., 2001). The world average content of Cr in soils is 60 mg kg−1, but in soils developed from mafic and volcanic rocks, it can reach up to 10 000 mg kg−1 (Gonnelli and Renella, 2013). Cr contents of up to 2879 and 3865 mg kg−1 were reported for serpentine soils in Galicia (NW Spain) and Albania, respectively (Covelo et al., 2007; Shallari et al., 1998). Anthropogenic activities, e.g. the metallurgical industry, also lead to Cr accumulation in soils (Kabata-Pendias, 2011). Up to 195, 88, and 6228 mg kg−1 Cr were found in urban, agricultural, and industrial soils, respectively (Srinivasa Gowd et al., 2010; Wei and Yang, 2010). Speciation and adsorption on soil solid surfaces are the main processes controlling Cr toxicity in soils (Adriano, 2001; Shahid et al., 2017). Despite the various Cr oxidation states, Cr(III) and Cr(VI) are the most stable and common forms in soils. Cr(VI) is considered the most toxic form of Cr, while Cr (III) is less mobile and less toxic and presents mostly as precipitate (Kabata-Pendias, 2011). The adsorption of Cr on soil solid surfaces depends on several factors, e.g. soil pH, clay content, organic matter, or Fe hydroxides (Bolan and Thiagarajan, 2001; Bradl, 2004; Dias-Ferreira et al., 2015; Gonnelli and Renella, 2013; Kabata-Pendias, 2011).
In the assessment of metal pollution, the toxic metal effect on soil microorganisms should be considered because of their key role in maintaining soil ecosystem functions (Nannipieri et al., 2003). Lower microbial diversity, enzymatic activity, C mineralization, and microbial biomass were found in Cr-polluted soil in comparison to unpolluted soil (Dotaniya et al., 2017; He et al., 2016; Pradhan et al., 2019). The potential nitrification and microbial abundance were inhibited with the increase in Cr level in the soil (Zhang et al., 2022). Bacterial diversity was negatively correlated with total and available Cr, while microbial community structure was altered (Zhang et al., 2021). However, sometimes differentiating whether the microbial response is due to Cr toxicity or to soil property variation is a difficult task (Liu et al., 2019), in addition to the complex biogeochemical behaviour of Cr in soils (Ao et al., 2022). Therefore, a microbial indicator specifically related to Cr toxicity that reduces interference of other soil properties is needed to assess the Cr toxicity, such as the pollution-induced community tolerance (PICT) methodology. PICT is a sensitive tool that can be used as a direct indicator of metal toxicity in the microbial community (Blanck, 2002). The PICT methodology is based on the selective pressure that the metal exerts on a microbial community, which favoured the proliferation of more tolerant species over the more sensitive ones. Thus, the microbial community that was exposed to the pollutant should show higher tolerance than that of the unexposed reference microbial community (Blanck, 2002; Tlili et al., 2016). The PICT methodology has been successfully applied to assess Cr pollution in soils and sediments (Gong et al., 2002; Ipsilantis and Coyne, 2007; Ogilvie and Grant, 2008; Santás-Miguel et al., 2021; Shi et al., 2002a, b; Van Beelen et al., 2004). The microbial community tolerance should be quantified in a short-term assay by a sensitive endpoint, such as bacterial growth measured using [3H]-leucine incorporation (Berg et al., 2012; Boivin et al., 2006; Lekfeldt et al., 2014). Despite the high sensitivity and specificity, the PICT methodology might present some difficulties, mainly due to the influence of soil properties (Blanck, 2002; Lekfeldt et al., 2014). Shi et al. (2002b) found similar values of PICT to Cr and Pb both at low and high Cr (263 g kg−1) and Pb (10 000 mg kg−1) levels, respectively, suggesting that different soils affected Cr and Pb bioavailability. Similarly, Shi et al. (2002a) did not find bacterial community tolerance to Cr (or Pb), regardless of exposure history to Cr (or Pb), suggesting that several factors (organic matter, pH, redox potential) might influence metal availability. Boivin et al. (2006), Fernández-Calviño et al. (2012), and Fernández-Calviño and Bååth (2016) also reported different tolerance values to heavy metals in soils with similar values of metals but different soil properties. Soil properties may affect PICT development due to effects on metals speciation, adsorption, and bioavailability (Bradl, 2004; Shahid et al., 2017).
We hypothesize that soil pollution with Cr induces the development of bacterial community tolerance to Cr, but the magnitude of the increases depends on soil physicochemical characteristics. Therefore, we aim to determine the induced bacterial community tolerance to Cr in response to the addition of different Cr levels to 10 soils with variable properties. We also aim to assess the importance of soil properties for the increase in bacterial community tolerance to Cr.
2.1 Soil samples
Soil samples were the same as used previously in Campillo-Cora et al. (2021a, 2020) to study Cr adsorption and fractionation in soils with different properties, mainly in terms of organic matter and pH. In brief, 10 remote forest locations in Galicia (NW Spain) were selected to avoid heavy metal pollution. Locations were also selected to obtain soil samples with a range of different physicochemical properties (Macías-Vázquez and Calvo de Anta, 2009). Superficial soil samples (0–20 cm) were taken using an Edelman probe and, once in the laboratory, were air-dried, homogenized, sieved (2 mm mesh), and stored until analysis.
2.2 Soil properties
A detailed description of the chemical analysis is given in Campillo-Cora et al. (2020) and in the Supplement. The properties of the 10 soils can be found in Tables S1 and S2. In brief, soil samples presented a wide range of textures (19 %–71 % sand, 13 %–67 % silt, 14 %–32 % clay). A wide range of soil pHW and pHK was found: 4.0–7.5 and 3.0–6.9, respectively. Similarly, organic matter (OM) oscillated between 10 %–29 %. A range from 2 to 29 cmolc kg−1in the manuscript) you are referring to data and was obtained for effective cation exchange capacity (eCEC). A large range was obtained for dissolved organic carbon (DOC): 0.14 to 0.70 g kg−1. Chromium total content varied from 7 up to 394 mg kg−1.
Adsorption constants determined from the Freundlich and Langmuir models (batch experiments) are presented in Table S3, obtained from Campillo-Cora et al. (2020). The different Cr fractions from extractions using distilled water, CaCl2, and diethylenetriamine pentaacetate (DTPA) are shown in Table S4, obtained from Campillo-Cora et al. (2021a).
2.3 Experimental design and bacterial community tolerance to Cr determination
Sieved soil samples were rewetted until reaching 60 %–80 % of water holding capacity (Meisner et al., 2013). To rewet, soil samples were spiked with seven Cr solutions (made from K2Cr2O7) and one of distilled water to obtain the following final Cr levels in soils: 2000, 1000, 500, 250, 125, 62.5, 31.25, and 0 mg Cr kg−1 soil. Each Cr solution was added separately and in triplicate, finally obtaining 240 microcosms (10 soils × 8 [Cr] × 3 replicates). These concentrations were selected as previously undertaken in Campillo-Cora (2020, 2021a), as they represent a broad exponential range of Cr contamination, which promotes the development of bacterial community tolerance to Cr, despite the considerable variability in soil properties. This facilitates subsequent comparisons of bacterial community tolerance to Cr results between the different soils studied. Once soil samples were spiked with Cr, microcosms were incubated in the dark at 22 ∘C for 2 months to ensure the reactivation of bacterial communities (Meisner et al., 2013).
After the incubation period, bacterial community tolerance to Cr was estimated through the PICT methodology (Blanck, 2002). The homogenization–centrifugation technique was performed to extract soil bacterial communities (Bååth, 1992). The bacterial community tolerance to Cr was determined as previously for Cu (Fernández-Calviño et al., 2011), with modifications based on suggestions by Lekfeldt et al. (2014). For this purpose, each microcosm was distributed in three 50 mL centrifuge tubes and MES buffer (4-morpholinoethanesulfonic acid, CAS no: 4432-31-9) was added at a ratio of 1:10 soil buffer (20 Mm pH 6) (Lekfeldt et al., 2014). The soil MES suspensions were mixed using a multi-vortex at maximum intensity for 3 min. This step was followed by low-speed centrifugation to remove most of the fungal biomass (1000 × g, 10 min) (Bååth, 1994; Bååth et al., 2001; Rousk and Bååth, 2011). Soil supernatants, i.e. bacterial suspensions, were filtered through glass wool and 1.5 mL aliquots were transferred into 2 mL micro-centrifugation tubes. A volume of 0.15 mL of different Cr concentrations (made from K2Cr2O7) was added to micro-centrifugation tubes, obtaining nine Cr concentrations (3.3 × 10−4 to 10−8 M) plus a blank (0.15 mL of distilled water). Then, the 3H-leucine incorporation method was used to estimate bacterial growth (Bååth et al., 2001). A volume of 0.2 µL [3H]Leu (37 MBq mL−1 and 5.74 TBq mmol−1. Amersham) with non-labelled Leu (19.8 µL) was added to each tube, resulting in 300 nM Leu in the bacterial suspensions. Bacterial suspensions were incubated for 8 h at 22 ∘C. Bacterial growth was stopped with 75 µL of 100 % trichloroacetic acid. The washing procedure and subsequent radioactivity measurement were carried out according to Bååth et al. (2001). Radioactivity was measured by liquid scintillation counting using a Tri-Carb 2810 TR (PerkinElmer, USA)
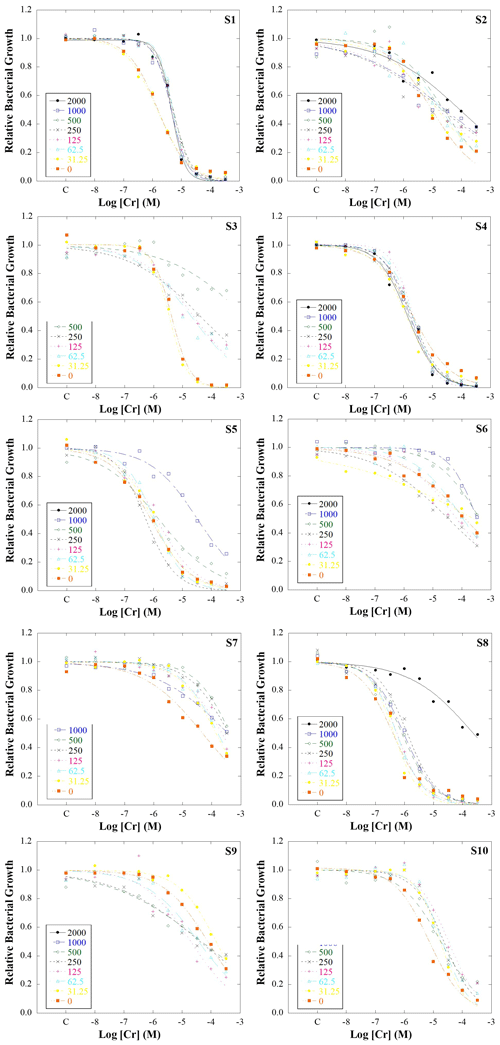
Figure 1Bacterial growth inhibition curves for bacterial suspensions extracted from 10 soils artificially polluted with a range of Cr concentrations: 2000, 1000, 500, 125, 62.5, 31.25, and 0 mg kg−1. Dots indicate real data measured, while the lines represent the fit of the data to the logistic model used. S1, S2, S3, S5, S6, S7, S8, S9, and S10 refer to studied soils 1, 2, 3, 4, 5, 6, 7, 8, 9, and 10, respectively.
2.4 Data analysis
2.4.1 Estimation of bacterial community tolerance to Cr (log IC50)
A dose–response curve was obtained for each soil microcosm. To compare the dose–response curves, i.e. inhibition curves, with each other, bacterial growth was expressed as relative bacterial growth. For each inhibition curve, generally, the four lowest concentrations of metal added to bacterial suspensions did not result in bacterial growth inhibition (Fig. 1). Thus, relative bacterial growth was calculated by dividing all bacterial growth data by the average of results from the four lowest added-metal concentrations (including blank), obtaining comparable dose–response curves. From each dose–response curve, log IC50 was determined as a tolerance index, i.e. Cr concentration resulting in 50 % inhibition of bacterial community growth. Higher log IC50 values mean higher bacterial community tolerance to Cr, and lower log IC50 values mean lower bacterial community tolerance to Cr. Log IC50 was calculated using the following logistic model (Fernández-Calviño et al., 2011):
where Y is the measured level of Leu incorporation, c is the bacterial growth rate without added Cr, b is a slope parameter indicating the inhibition rate, X is the logarithm of Cr added, and a is log IC50.
To detect whether bacterial community tolerance increased from different studied soils occurs, Δlog IC50 was determined as the difference between log IC50 value from each Cr level in soil (2000, 1000, 500, 250, 125, 62.5, or 31.25 mg Cr kg−1) and the control soil (0 mg Cr kg−1). A difference of 0.3 was taken as a reference value to determine whether bacterial community tolerance increased since it represents twice the Cr concentration in terms of added Cr to bacterial suspensions. If Δlog IC50 is higher than 0.3, we will consider an increase in bacterial community tolerance to Cr (Fernández-Calviño and Bååth, 2016, 2013).
2.4.2 Estimation of bacterial community tolerance increase to Cr (multiple linear regression analyses)
A multiple regression analysis, using the backward elimination method, was performed to obtain an equation that allows estimating the increase in bacterial community tolerance to Cr (Δlog IC50) from soil properties (Campillo-Cora et al., 2021b, 2022a, b). As the inhibition curves for some soils did not fit the logistic model (Eq. 1) for the highest Cr concentrations (1000 and 2000 mg kg−1), Δlog IC50 from 500 mg kg−1 was used for estimations. Once the equation was estimated, determining factors were verified: linearity, error independency, residue homoscedasticity, residual normality, autocorrelation, collinearity, and the presence of outliers. All statistics were performed using the IBM SPSS Statistics 25 software (IBM, USA).
3.1 Bacterial community tolerance to Cr in Cr-polluted soils with different properties
Figure 1 shows bacterial growth inhibition curves obtained for each microcosm. Generally, a sigmoid dose–response behaviour is observed in the inhibition curves, indicating that when the added Cr concentration to bacterial suspension was low, relative bacterial growth was close to 1, while it decreased when the Cr concentration increased. Most of the bacterial growth data fitted the logistic model, resulting in R2≥0.87 (Table S5). However, some data from 1000 and 2000 mg Cr kg−1 did not fit the logistic model; i.e. bacterial populations were not able to grow normally probably due to high Cr toxicity. In the case of 2000 mg kg−1, bacterial populations only grew normally in 4 of the 10 studied soils, while at 1000 mg kg−1 they grew normally in 7 soils. These differences in bacterial growth for the same Cr levels may indicate the influence of soil properties on Cr availability, as was previously suggested by Van Beelen et al. (2004). They found tolerant communities to Cr(III) in polluted soils with high Cr levels (2894 mg kg−1) but also reported that microbial communities from soils polluted with 3935 mg Cr kg−1 did not show tolerance to Cr(III), suggesting the influence of soil properties on metal toxicity. Therefore, in order to determine which properties influence Cr toxicity, the data of 1000 and 2000 mg Cr kg−1 were not considered in the following analysis.
Table 1Bacterial community tolerance (expressed as log IC50) to different levels of Cr pollution in the 10 studied soils (average ± SE).
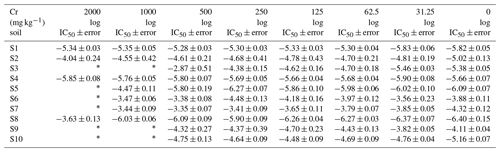
∗ Unadjusted data.
The log IC50 values determined from inhibition curves using the logistic model (Eq. 1) are presented in Table 1. Bacterial community tolerance to Cr (log IC50) greatly varied between soils, even in the reference soils with no added Cr, log IC50 oscillated from −6.40 (S8) up to −3.88 (S6) (log units). The variation in bacterial community tolerance to Cr in the reference soils may be an indicator that the development of PICT is dependent on soil type. In addition, this bacterial community tolerance to Cr fluctuation in reference soils, together with the natural Cr content in soils (7–394 mg kg−1, Table S2), highlights the importance of selecting reference soils for PICT studies (Campillo-Cora et al., 2022a, 2021b). Likewise, when Cr was added to soils, bacterial community tolerance to Cr varied greatly between soils with the same Cr level. A range from −6.37 (S8) to −3.56 (S6) was determined for soils polluted with the lowest Cr level in soil (31.25 mg Cr kg−1), from −6.27 (S8) to −3.79 (S7) for 62.5 mg Cr kg−1, from −6.26 (S8) to −3.65 (S7) for 125 mg Cr kg−1, from −6.27 (S5) to −3.41 (S7) for 250 mg Cr kg−1, and from −6.09 (S8) to −2.87 (S3) for 500 mg kg−1.
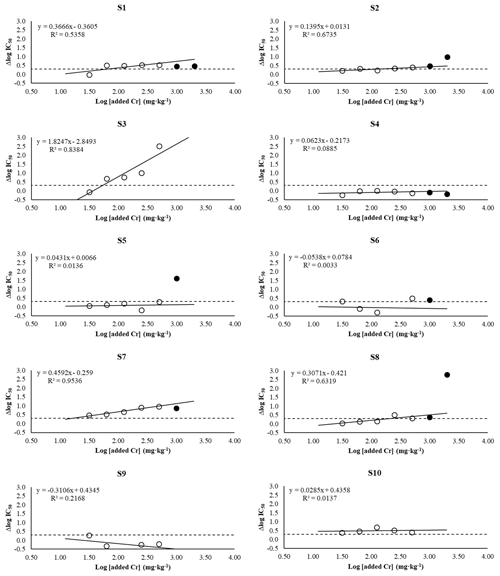
Figure 2Bacterial community tolerance variation (expressed as Δlog IC50 concerning unpolluted soil) to a range of added Cr to soil (on a logarithm scale). White dots represent data from Δlog IC50(31.25−0), Δlog IC50(62.5−0), Δlog IC50(125−0), Δlog IC50(250−0), and Δlog IC50(500−0). Black dots represent data from Δlog IC50(1000−0) and Δlog IC50(2000−0). Continuous lines represent the linear regression fit. The discontinuous line represents the value (0.3) from which it is considered that the bacterial community has developed tolerance. S1, S2, S3, S5, S6, S7, S8, S9, and S10 refer to studied soils 1, 2, 3, 4, 5, 6, 7, 8, 9, and 10, respectively.
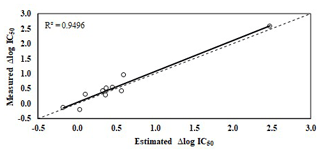
Figure 3Relationship between measured and estimated Δlog IC50 using the equation from Table 2. The stippled line indicated a 1:1 relationship.
Overall, bacterial communities showed two different responses to Cr addition to the soil (Fig. 2): (1) bacterial communities of S1, S2, S3, S6, S7, S8, and S10 developed tolerance in response to Cr additions, while (2) bacterial communities of S4, S5, and S9 did not develop tolerance following Cr addition to the soil. Based on the PICT hypothesis, the bacterial community is first exposed to the metal (i.e. selection phase of PICT), and if metal exerts toxicity, then the most sensitive organisms of the community will disappear, while the tolerant ones will be favoured. Therefore, whether the microbial community developed tolerance to Cr is a toxicity indicator. Later, the microbial community tolerance is quantified through a second exposition to Cr (i.e. detection phase of PICT) (Blanck, 2002; Tlili et al., 2016). Accordingly, Gong et al. (2002) and Ipsilantis and Coyne (2007) reported an increase in bacterial community tolerance to Cr with increasing Cr levels in soil and rhizosphere. Van Beelen et al. (2004) found that bacterial community tolerance to Cr(VI) increased with increasing Cr in pore water. Ogilvie and Grant (2008) determined a tendency for the bacterial community tolerance to Cr to increase when the Cr level increases in estuarine sediments. Our results showed that bacterial community tolerance to Cr increased with increasing Cr levels in soils only in 7 of the 10 soils studied (Fig. 2). However, our results showed that the Cr level in soil from which bacterial communities developed tolerance to Cr varied depending on the soil (Δlog IC50>0.3). Bacterial communities from S7 and S10 showed an increased tolerance at 31.25 mg Cr kg−1, bacterial communities from S1 and S3 showed it at 62.5 mg Cr kg−1, bacterial communities from S2 and S8 showed it at 250 mg Cr kg−1, and bacterial communities from S6 showed it at 500 mg Cr kg−1. In other words, Cr was more toxic for bacterial communities depending on soil type, following the sequence: S7, S10 > S1, S3 > S2, and S8 > S6. In other soils, our results show that microbial communities did not develop tolerance to Cr, even at high Cr levels. For example, bacterial communities of S6 did not show tolerance to Cr even at 2000 mg kg−1 (Fig. 2). Similarly, Shi et al. (2002a, b) and Ipsilantis and Coyne (2007) did not find tolerant microbial communities to Cr even at high Cr levels, from 447 up to 263 000 mg Cr kg−1. Therefore, considering that Cr pollution sometimes has no toxic effect on microbial communities and that, in other cases, microbial communities are affected by Cr from very low levels of Cr pollution, including soil properties in the assessment of Cr pollution is highly recommended, as for other heavy metals (Campillo-Cora et al., 2022b).
3.1.1 Estimation of the increase in bacterial community tolerance to Cr as a function of soil properties
The bacterial community tolerance to metals may be influenced by several soil properties, such as soil pH, clay content, or organic matter content (Ogilvie and Grant, 2008; Shi et al., 2002b). The effect of soil properties on bacterial community tolerance can occur in soil (selection phase of PICT) or in the determination phase of PICT. The effect of the soil properties in the selection phase occurs in the soil, i.e. the first time bacterial communities are exposed to the metal. For example, Fernández-Calviño and Bååth (2016) found that bacterial community tolerance to Cu was lower in vineyard soils with high pH in comparison to more acid soils, as Cu toxicity was reduced. On the other hand, the effect of soil properties may occur in the detection phase, i.e. confounding factors leading to altered tolerance measures (Lekfeldt et al., 2014). For example, Fernández-Calviño et al. (2011) reported that the measurement of PICT to Cu was altered because of the presence of the finer soil fraction in the bacterial suspensions when Cu concentrations were added. That is, the finer particles will bind part of the Cu added to bacterial suspensions, resulting in lower available Cu, so higher Cu concentrations will be necessary to inhibit the bacterial growth leading to apparent higher tolerance, i.e. an overestimated bacterial community tolerance to Cu.
The equation presented in Table 2 related the increase in bacterial community tolerance to Cr (Δlog IC50) with soil properties, explaining 95.6 % of the data variance (p<0.001). Only Δlog IC50 for 500 mg Cr kg−1 was used. The increase in bacterial community tolerance to Cr was estimated by using soil properties (p<0.05): DOC and extracted Cr using distilled water (H2O-Cr). Figure 3 shows estimated Δlog IC50 versus measured Δlog IC50, with a homogeneous distribution around the 1:1 line (R2=0.95).
DOC showed a significant positive relationship with Δlog IC50 (p<0.05; Table 2); i.e. when DOC increases, the bacterial community tolerance to Cr also increases. This DOC effect might be a confounding factor in the detection phase of PICT, as was previously reported for Cu (Campillo-Cora et al., 2021b; Lekfeldt et al., 2014). When bacterial communities are extracted from soil, DOC is extracted too. Later, when Cu is added to bacterial suspensions, Cu and DOC may bind together (Beesley et al., 2010), reducing Cu bioavailability and altering bacterial community tolerance to Cr (overestimation). Bérard et al. (2016) reported a similar effect for microbial community tolerance to Pb measurements. However, in a previous study (Campillo-Cora et al., 2023), we found that when dissolved organic matter (DOM) increases in bacterial suspensions, then bacterial community tolerance to Cr decreases; i.e. when DOM increases in bacterial suspensions, Cr becomes more toxic to bacteria. Hence, the DOC effect in Cr bioavailability in the detection phase should be discarded because of the positive relationship with Δlog IC50 (Table 2) and attributed to an effect in the selection phase in soil. In the soil, however, when DOC is present, Cr(VI) may be reduced to Cr(III); i.e. Cr toxicity decreases when DOC is present (Ao et al., 2022). If fact, the use of organic amendments to reduce Cr toxicity in soils is very common (Abou Jaoude et al., 2020; Mitchell et al., 2018; Yang et al., 2021). A hypothesis is that the presence of DOC in soil enhances the reduction of Cr(VI) to Cr(III) (Wittbrodt and Palmer, 1997), but during this process free radicals may also be formed (Kotaś and Stasicka, 2000), increasing general toxicity for bacterial communities (Campillo-Cora et al., 2023). In response to increased toxicity in soil, then, bacterial communities showed tolerance to Cr. Another hypothesis might be the ability of Cr(III) to coordinate various organic compounds, leading to the inhibition of some metalloenzyme systems (Kotaś and Stasicka, 2000), which might result in a more tolerant bacterial community.
Table 2The equation for estimating bacterial community tolerance increase to Cr (Δlog IC50(500−0)) was obtained by multiple regression analysis using all soil samples (n=10).
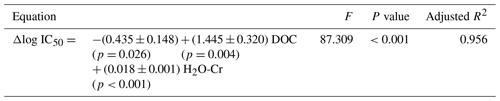
DOC is dissolved organic carbon (g kg−1); H2O-Cr is Cr extracted using H2O. Values associated with the independent variables are shown together with the standard errors (±). P values associated with each independent variable are shown below variables (in brackets).
The Cr fraction extracted with distilled water (H2O-Cr) showed a positive relationship with Δlog IC50 (p<0.001, Table 2). Usually, the soluble form of heavy metals represents the soil solution metal content, which is the most mobile and bioavailable form (Kabata-Pendias, 2011) and in the case of Cr in soils is usually Cr(VI) (Ao et al., 2022). Thus, H2O-Cr exerts its effect in soil, during the selection phase. H2O-Cr content in soil increases as the added Cr level in soils increases (Campillo-Cora et al., 2021a). If Cr exerts toxicity, the most sensitive bacterial species would be removed, while the tolerant ones would survive, resulting in a community more tolerant to Cr. Later, in the detection phase, when bacterial growth is measured and Cr is added to bacterial suspensions, tolerant bacteria allow greater Cr concentrations, leading to a higher tolerant community. Van Beelen et al. (2004) found a significant increase in microbial community tolerance to Cr(VI) with Cr(VI) pore-water concentration. Similarly, Fernández-Calviño and Bååth (2016) reported a positive relationship between bacterial community tolerance increase (Δlog IC50) to Cu versus a water-soluble Cu concentrations logarithm (R2=0.79). Kunito et al. (1999) also determined a positive correlation between IC50 values and soluble–exchangeable Cu (r=0.76), while total Cu did not show any significant relationship (r=0.013, p>0.05).
3.2 Concluding remarks
In the present study, we aimed to improve the PICT methodology for the assessment of soil pollution, using bacterial growth as the endpoint. Dissolved organic carbon (DOC) and the fraction of Cr extracted with distilled water (H2O-Cr) were the main factors controlling the Cr effect on microbial communities, determined by the increase in bacterial community tolerance to Cr. The main selection pressure of Cr on the microbial community presumably occurs in soil, i.e. the selection phase of PICT. In the case of DOC, Cr became more toxic to bacterial communities as DOC increased in soil, leading to an increase in bacterial community tolerance to Cr in response to toxicity. Secondly, H2O-Cr is related to the toxic and active form of Cr, probably Cr(VI), and the higher the H2O-Cr content in the soil, the higher the tolerance to Cr developed by bacterial communities. The outcomes of this study may be helpful for normalizing Cr toxicity thresholds for soil with different properties. In addition, overestimations or underestimations of Cr toxicity based on total or bioavailable Cr content may be avoided, since soil properties should be considered during risk assessment.
Data will be made available on request.
The supplement related to this article is available online at: https://doi.org/10.5194/soil-9-561-2023-supplement.
Conceptualization: MAE and DFC; Methodology: MAE and DFC; Formal analysis: CCC and DFC; Investigation: CCC, DAL and DFC; Writing – original draft preparation: CCC and DFC; writing – review and editing: CCC, DAL and MAE; funding acquisition: MAE and DFC; Data curation: CCC and DAL; Supervision: MAE and DFC; project administration: DFC.
The contact author has declared that none of the authors has any competing interests.
Publisher's note: Copernicus Publications remains neutral with regard to jurisdictional claims made in the text, published maps, institutional affiliations, or any other geographical representation in this paper. While Copernicus Publications makes every effort to include appropriate place names, the final responsibility lies with the authors.
David Fernández-Calviño holds a Ramón y Cajal contract (RYC-2016-20411) financed by the Spanish Ministry of Economy, Industry and Competitiveness. Daniel Arenas-Lago thanks the Ministerio de Ciencia e Innovación of Spain and the University of Vigo for the postdoc grant Juan de la Cierva Incorporación 2019 (IJC2019-042235-I). Claudia Campillo-Cora holds a Predoctoral fellowship financed by Xunta de Galicia (ED481A-2020/084). Funding for the open-access charge was provided by Universidade de Vigo/CISUG.
This research has been supported by the Ministerio de Economía y Competitividad (grant no. CTM2015-73422-JIN).
This paper was edited by Lisa Ciadamidaro and reviewed by two anonymous referees.
Abou Jaoude, L., Castaldi, P., Nassif, N., Pinna, M. V., and Garau, G.: Biochar and compost as gentle remediation options for the recovery of trace elements-contaminated soils, Sci. Total Environ., 711, 134511, https://doi.org/10.1016/j.scitotenv.2019.134511, 2020.
Adriano, D. C.: Trace Elements in Terrestrial Environments, 2 Edn., Springer, New York, https://doi.org/10.1007/978-0-387-21510-5, 2001.
Ao, M., Chen, X., Deng, T., Sun, S., Tang, Y., Morel, J. L., Qiu, R., and Wang, S.: Chromium biogeochemical behaviour in soil-plant systems and remediation strategies: A critical review, J. Hazard. Mater., 424, 127233, https://doi.org/10.1016/j.jhazmat.2021.127233, 2022.
Bååth, E.: Thymidine incorporation into macromolecules of bacteria extracted from soil by homogenization-centrifugation, Soil Biol. Biochem., 24, 1157–1165, https://doi.org/10.1016/0038-0717(92)90066-7, 1992.
Bååth, E.: Thymidine and leucine incorporation in soil bacteria with different cell size, Microb. Ecol., 27, 267–278, https://doi.org/10.1007/BF00182410, 1994.
Bååth, E., Pettersson, M., and Söderberg, K. H.: Adaptation of a rapid and economical microcentrifugation method to measure thymidine and leucine incorporation by soil bacteria, Soil Biol. Biochem., 33, 1571–1574, https://doi.org/10.1016/S0038-0717(01)00073-6, 2001.
Beesley, L., Moreno-Jiménez, E., and Gomez-Eyles, J. L.: Effects of biochar and greenwaste compost amendments on mobility, bioavailability and toxicity of inorganic and organic contaminants in a multi-element polluted soil, Environ. Pollut., 158, 2282–2287, https://doi.org/10.1016/J.ENVPOL.2010.02.003, 2010.
Bérard, A., Capowiez, L., Mombo, S., Schreck, E., Dumat, C., Deola, F., and Capowiez, Y.: Soil microbial respiration and PICT responses to an industrial and historic lead pollution: a field study, Environ. Sci. Pollut. Res., 23, 4271–4281, https://doi.org/10.1007/s11356-015-5089-z, 2016.
Berg, J., Brandt, K. K., Al-Soud, W. A., Holm, P. E., Hansen, L. H., Sørensen, S. J., and Nybroe, O.: Selection for Cu-tolerant bacterial communities with altered composition, but unaltered richness, via long-term cu exposure, Appl. Environ. Microbiol., 78, 7438–7446, https://doi.org/10.1128/AEM.01071-12, 2012.
Blanck, H.: A critical review of procedures and approaches used for assessing pollution-induced community tolerance (PICT) in biotic communities, Hum. Ecol. Risk Assess., 8, 1003–1034, https://doi.org/10.1080/1080-700291905792, 2002.
Boivin, M. E. Y., Greve, G. D., Kools, S. A. E., van der Wurff, A. W. G., Leeflang, P., Smit, E., Breure, A. M., Rutgers, M., and van Straalen, N. M.: Discriminating between effects of metals and natural variables in terrestrial bacterial communities, Appl. Soil Ecol., 34, 103–113, https://doi.org/10.1016/j.apsoil.2006.03.009, 2006.
Bolan, N. S. and Thiagarajan, S.: Retention and plant availability of chromium in soils as affected by lime and organic matter amendments, Aust. J. Soil Res., 39, 1091–1103, https://doi.org/10.1071/SR00090, 2001.
Bradl, H. B.: Adsorption of heavy metal ions on soils and soils constituents, J. Colloid Interface Sci., 277, 1–18, https://doi.org/10.1016/j.jcis.2004.04.005, 2004.
Campillo-Cora, C., Conde-Cid, M., Arias-Estévez, M., Fernández-Calviño, D., and Alonso-Vega, F.: Specific adsorption of heavy metals in soils: Individual and competitive experiments, Agronomy, 10, 1113, https://doi.org/10.3390/agronomy10081113, 2020.
Campillo-Cora, C., González-Feijoo, R., Arias-Estévez, M., and Fernández-Calviño, D.: Dissolved organic matter as a confounding factor in the determination of pollution-induced community tolerance (PICT) of bacterial communities to heavy metals using the leucine incorporation method, Geoderma, 430, 116335, https://doi.org/10.1016/j.geoderma.2023.116335, 2023
Campillo-Cora, C., Rodríguez-González, L., Arias-Estévez, M., Fernández-Calviño, D., and Soto-Gómez, D.: Influence of physicochemical properties and parent material on chromium fractionation in soils, Processes, 9, 1073, https://doi.org/10.3390/pr9061073, 2021a.
Campillo-Cora, C., Soto-Gómez, D., Arias-Estévez, M., Bååth, E., and Fernández-Calviño, D.: Estimation of baseline levels of bacterial community tolerance to Cr, Ni, Pb, and Zn in unpolluted soils, a background for PICT (pollution-induced community tolerance) determination, Biol. Fertil. Soils, 58, 49–61, https://doi.org/10.1007/s00374-021-01604-x, 2022a.
Campillo-Cora, C., Soto-Gómez, D., Arias-Estévez, M., Bååth, E., and Fernández-Calviño, D.: Bacterial community tolerance to Cu in soils with geochemical baseline concentrations (GBCs) of heavy metals.: Importance for pollution induced community tolerance (PICT) determinations using the leucine incorporation method, Soil Biol. Biochem., 155, 108157, https://doi.org/10.1016/j.soilbio.2021.108157, 2021b.
Campillo-Cora, C., González-Feijoo, R., Arias-Estévez, M., and Fernández-Calviño, D.: Influence of soil properties on the development of bacterial community tolerance to Cu, Ni, Pb and Zn. Environ. Res., 214, 113920, https://doi.org/10.1016/J.ENVRES.2022.113920, 2022b.
Cervantes, C., Campos-García, J., Devars, S., Gutiérrez-Corona, F., Loza-Tavera, H., Torres-Guzmán, J. C., and Moreno-Sánchez, R.: Interactions of chromium with microorganisms and plants, FEMS Microbiol. Rev., 25, 335–347, https://doi.org/10.1016/S0168-6445(01)00057-2, 2001.
Covelo, E. F., Vega, F. A., and Andrade, M. L.: Heavy metal sorption and desorption capacity of soils containing endogenous contaminants, J. Hazard. Mater., 143, 419–430, https://doi.org/10.1016/j.jhazmat.2006.09.047, 2007.
Dias-Ferreira, C., Kirkelund, G. M., and Ottosen, L. M.: Ammonium citrate as enhancement for electrodialytic soil remediation and investigation of soil solution during the process, Chemosphere, 119, 889–895, https://doi.org/10.1016/j.chemosphere.2014.08.064, 2015.
Dotaniya, M. L., Rajendiran, S., Meena, V. D., Saha, J. K., Coumar, M. V., Kundu, S., and Patra, A. K.: Influence of Chromium Contamination on Carbon Mineralization and Enzymatic Activities in Vertisol, Agric. Res., 6, 91–96, https://doi.org/10.1007/S40003-016-0242-6/TABLES/5, 2017.
Fernández-Calviño, D., Arias-Estévez, M., Díaz-Raviña, M., and Bååth, E.: Assessing the effects of Cu and pH on microorganisms in highly acidic vineyard soils, Eur. J. Soil Sci., 63, 571–578, https://doi.org/10.1111/j.1365-2389.2012.01489.x, 2012.
Fernández-Calviño, D., Arias-Estévez, M., Díaz-Raviña, M., and Bååth, E.: Bacterial pollution induced community tolerance (PICT) to Cu and interactions with pH in long-term polluted vineyard soils, Soil Biol. Biochem., 43, 2324–2331, https://doi.org/10.1016/j.soilbio.2011.08.001, 2011.
Fernández-Calviño, D. and Bååth, E.: Co-selection for antibiotic tolerance in Cu-polluted soil is detected at higher Cu-concentrations than increased Cu-tolerance, Soil Biol. Biochem., 57, 953–956, https://doi.org/10.1016/j.soilbio.2012.08.017, 2013.
Fernández-Calviño, D. and Bååth, E.: Interaction between pH and Cu toxicity on fungal and bacterial performance in soil, Soil Biol. Biochem., 96, 20–29, https://doi.org/10.1016/j.soilbio.2016.01.010, 2016.
Gong, P., Siciliano, S. D., Srivastava, S., Greer, C. W., and Sunahara, G. I.: Assessment of pollution-induced microbial community tolerance to heavy metals in soil using ammonia-oxidizing bacteria and biolog assay, Hum. Ecol. Risk Assess., 8, 1067–1081, https://doi.org/10.1080/1080-700291905828, 2002.
Gonnelli, C. and Renella, G.: Heavy Metals in Soils, 3 Edn., Springer, Dordrecht, Netherlands, https://doi.org/10.1007/978-94-007-4470-7, 2013.
He, Z., Hu, Y., Yin, Z., Hu, Y., and Zhong, H.: Microbial Diversity of Chromium-Contaminated Soils and Characterization of Six Chromium-Removing Bacteria, Environ. Manage., 57, 1319–1328, https://doi.org/10.1007/s00267-016-0675-5, 2016.
Ipsilantis, I. and Coyne, M. S.: Soil microbial community response to hexavalent chromium in planted and unplanted soil, J. Environ. Qual., 36, 638–645, https://doi.org/10.2134/jeq2005.0438, 2007.
Kabata-Pendias, A.: Trace Elements in Soils and Plants, 4 Edn., Boca Raton, CRC Press, Taylor & Francis Group, New York, https://doi.org/10.1201/b10158, 2011.
Kotaś, J. and Stasicka, Z.: Chromium occurrence in the environment and methods of its speciation, Environ. Pollut., 107, 263–283, https://doi.org/10.1016/S0269-7491(99)00168-2, 2000.
Kunito, T., Saeki, K., Oyaizu, H., and Matsumoto, S.: Influences of copper forms on the toxicity to microorganisms in soils, Ecotoxicol. Environ. Saf., 44, 174–181, https://doi.org/10.1006/eesa.1999.1820, 1999.
Lekfeldt, J. D. S., Magid, J., Holm, P. E., Nybroe, O., and Brandt, K. K.: Evaluation of the leucine incorporation technique for detection of pollution-induced community tolerance to copper in a long-term agricultural field trial with urban waste fertilizers, Environ. Pollut., 194, 78–85, https://doi.org/10.1016/j.envpol.2014.07.013, 2014.
Liu, B., Su, G., Yang, Y., Yao, Y., Huang, Y., Hu, L., Zhong, H., and He, Z.: Vertical distribution of microbial communities in chromium-contaminated soil and isolation of Cr(Y)-Reducing strains, Ecotoxicol. Environ. Saf., 180, 242–251, https://doi.org/10.1016/j.ecoenv.2019.05.023, 2019.
Macías-Vázquez, F. and Calvo de Anta, R.: Niveles Genéricos de Referencia de metales pesados y otros elementos traza en suelos de Galicia, Consellería de Medio Ambiente e Desenvolvemento Sostible, Santiago de Compostela, Spain, ISBN 978-84-453-4664-8, 2009.
Meisner, A., Bååth, E., and Rousk, J.: Microbial growth responses upon rewetting soil dried for four days or one year, Soil Biol. Biochem., 66, 188–192, https://doi.org/10.1016/j.soilbio.2013.07.014, 2013.
Mitchell, K., Trakal, L., Sillerova, H., Avelar-González, F. J., Guerrero-Barrera, A. L., Hough, R., and Beesley, L.: Mobility of As, Cr and Cu in a contaminated grassland soil in response to diverse organic amendments; a sequential column leaching experiment, J. Appl. Geochem., 88, 95–102, https://doi.org/10.1016/j.apgeochem.2017.05.020, 2018.
Nannipieri, P., Ascher, J., Ceccherini, M. T., Landi, L., Pietramellara, G., and Renella, G.: Microbial diversity and soil functions, Eur. J. Soil Sci., 54, 655–670, https://doi.org/10.1046/j.1351-0754.2003.0556.x, 2003.
Ogilvie, L. A. and Grant, A.: Linking pollution induced community tolerance (PICT) and microbial community structure in chronically metal polluted estuarine sediments, Mar. Environ. Res., 65, 187–198, https://doi.org/10.1016/j.marenvres.2007.10.002, 2008.
Pradhan, S. K., Kumar, U., Singh, N. R., and Thatoi, H.: Functional diversity and metabolic profile of microbial community of mine soils with different levels of chromium contamination, Int. J. Environ. Health Res., 30, 461–473, https://doi.org/10.1080/09603123.2019.1601686, 2019.
Rousk, J. and Bååth, E.: Growth of saprotrophic fungi and bacteria in soil, FEMS Microbiol. Ecol., 78, 17–30, https://doi.org/10.1111/j.1574-6941.2011.01106.x, 2011.
Santás-Miguel, V., Núñez-Delgado, A., Álvarez-Rodríguez, E., Díaz-Raviña, M., Arias-Estévez, M., and Fernández-Calviño, D.: Tolerance of soil bacterial community to tetracycline antibiotics induced by As, Cd, Zn, Cu, Ni, Cr, and Pb pollution, SOIL, 8, 437–449, https://doi.org/10.5194/soil-8-437-2022, 2022.
Shahid, M., Shamshad, S., Rafiq, M., Khalid, S., Bibi, I., Niazi, N. K., Dumat, C., and Rashid, M. I.: Chromium speciation, bioavailability, uptake, toxicity and detoxification in soil-plant system: A review, Chemosphere, 178, 513–533, https://doi.org/10.1016/j.chemosphere.2017.03.074, 2017.
Shallari, S., Schwartz, C., Hasko, A., and Morel, J. L.: Heavy metals in soils and plants of serpentine and industrial sites of Albania, Sci. Total Environ., 209, 133–142, https://doi.org/10.1016/S0048-9697(97)00312-4, 1998.
Shi, W., Becker, J., Bischoff, M., Turco, R. F., and Konopka, A. E.: Association of microbial community composition and activity with lead, chromium, and hydrocarbon contamination, Appl. Environ. Microbiol., 68, 3859–3866, https://doi.org/10.1128/AEM.68.8.3859-3866, 2002a.
Shi, W., Bischoff, M., Turco, R., and Konopka, A.: Long-term effects of chromium and lead upon the activity of soil microbial communities, Appl. Soil Ecol., 21, 169–177, https://doi.org/10.1016/S0929-1393(02)00062-8, 2002b.
Srinivasa Gowd, S., Ramakrishna Reddy, M., and Govil, P. K.: Assessment of heavy metal contamination in soils at Jajmau (Kanpur) and Unnao industrial areas of the Ganga Plain, Uttar Pradesh, India, J. Hazard. Mater., 174, 113–121, https://doi.org/10.1016/j.jhazmat.2009.09.024, 2010.
Tlili, A., Berard, A., Blanck, H., Bouchez, A., Cássio, F., Eriksson, K. M., Morin, S., Montuelle, B., Navarro, E., Pascoal, C., Pesce, S., Schmitt-Jansen, M., and Behra, R.: Pollution-induced community tolerance (PICT): towards an ecologically relevant risk assessment of chemicals in aquatic systems, Freshw. Biol., 61, 2141–2151, https://doi.org/10.1111/fwb.12558, 2016.
Van Beelen, P., Wouterse, M., Posthuma, L., and Rutgers, M.: Location-specific ecotoxicological risk assessment of metal-polluted soils, Environ. Toxicol. Chem., 23, 2769–2779, https://doi.org/10.1897/03-568, 2004.
Wei, B. and Yang, L.: A review of heavy metal contaminations in urban soils, urban road dusts and agricultural soils from China, Microchem. J., 94, 99–107, https://doi.org/10.1016/j.microc.2009.09.014, 2010.
Wittbrodt, P. R. and Palmer, C. D.: Reduction of Cr(VI) by soil humic acids, Europ. J. Soil Sci., 48, 151–162, https://doi.org/10.1111/j.1365-2389.1997.tb00194.x, 1997.
Yang, Z., Zhang, X., Jiang, Z., Li, Q., Huang, P., Zheng, C., Liao, Q., and Yang, W.: Reductive materials for remediation of hexavalent chromium contaminated soil – A review, Sci. Total Environ., 773, 145654, https://doi.org/10.1016/J.SCITOTENV.2021.145654, 2021.
Zhang, X., Gai, X., Zhong, Z., Bian, F., Yang, C., Li, Y., and Wen, X.: Understanding variations in soil properties and microbial communities in bamboo plantation soils along a chromium pollution gradient, Ecotoxicol. Environ. Saf., 222, 112507, https://doi.org/10.1016/J.ECOENV.2021.112507, 2021.
Zhang, X., Zhang, X., Li, L., Fu, G., Liu, X., Xing, S., Feng, H., and Chen, B.: The toxicity of hexavalent chromium to soil microbial processes concerning soil properties and aging time, Environ. Res., 204, 111941, https://doi.org/10.1016/J.ENVRES.2021.111941, 2022.