the Creative Commons Attribution 4.0 License.
the Creative Commons Attribution 4.0 License.
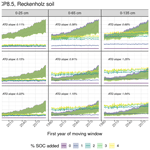
Sequestering carbon in the subsoil benefits crop transpiration at the onset of drought
Maria Eliza Turek
Attila Nemes
Annelie Holzkämper
Increasing soil organic carbon is promoted as a negative emission technology for the agricultural sector with a potential co-benefit for climate adaptation due to increased soil water retention. Field-scale hydrological models are powerful tools for evaluating how the agricultural systems would respond to the changing climate in upcoming years and decades, for predicting impacts, and for looking for measures that would help decrease drought-driven crop stress under current and future climatic conditions. We quantified how different levels of soil organic carbon (SOC) additions at varied soil depths are expected to influence drought-induced transpiration reduction (Treddry) in maize cultivated in Switzerland. Parameterization of the model based on a pedotransfer function (PTF) was validated against soil moisture data from a long-term lysimeter experiment with a typical Swiss soil, and the model was subsequently applied under climate forcing between 1981 until 2099, representative of three distinct climatic sites of Switzerland. We used the same PTF to indirectly assess the effects of SOC additions at different depths on soil hydraulic properties. We found a threshold in both the added amount of SOC (2 % added) and the depth of sequestering that SOC (top 65 cm), beyond which any additional benefit appears to be substantially reduced. However, adding at least 2 % SOC down to at least 65 cm depth can reduce Treddry in maize, i.e. increase transpiration annually but mostly at the onset of summer drought, by almost 40 mm. We argue that SOC increases in subsoils can play a supporting role in mitigating drought impacts in rain-fed cropping in Switzerland.
- Article
(2121 KB) - Full-text XML
-
Supplement
(2085 KB) - BibTeX
- EndNote
Over the last few decades, scientific studies have increasingly emphasized the need and explored potentials for soil carbon sequestration in agricultural soils to mitigate climate change (e.g. Lal, 2001, 2004; Minasny et al., 2017; Smith et al., 2008). In this context, other possible impacts of increasing soil organic carbon (SOC) on important soil functions and services have also been highlighted (e.g. on soil biodiversity, soil structure, and soil water retention and infiltration capacity; see Lal, 2004; Murphy, 2015). Management practices such as the application of organic amendments (i.e. compost, manure, biochar), cover cropping, crop diversification, and the adoption of conservation tillage systems are commonly considered to be beneficial for increasing SOC (Crystal-Ornelas et al., 2021). With an increase in soil organic carbon in terms of quantity, quality, and chemical diversity, soil communities are promoted, and biotic–abiotic interactions are enhanced, with positive impacts on the formation and storage of soil organic matter (Zhang et al., 2021). Physical properties of the soil are altered directly by soil organic carbon increases and indirectly through the activity of soil fauna (e.g. Arthur et al., 2015; Rivier et al., 2022; Nemes et al., 2005; Rawls et al., 2004). Soil structure has a major influence on the natural soil water retention capacity, an essential regulating ecosystem service provided by soils that may play an increasingly crucial role in mitigating drought-induced limitations as climate change progresses (Liu et al., 2021). Soil texture also strongly affects how soil hydraulic properties respond to organic amendments, as shown by a meta-analysis from Edeh et al. (2020), who reported decreased hydraulic conductivity of sandy soils and increased hydraulic conductivity of clayey soils after biochar additions. A recent meta-analysis performed for Europe has also shown that the adoption of organic amendments and continuous living cover benefit the soil water regulation functions (Blanchy et al., 2023).
With that in mind, the potential for achieving synergies between climate mitigation and adaptation seem promising. However, empirical evidence regarding the benefits of increasing soil organic carbon for reducing drought limitations in crops is inconclusive. For example, Minasny and McBratney (2017) performed a meta-analysis with globally distributed soil data combined with the development of pedotransfer functions (PTFs) and found that a 1 % increase in SOC has a minor effect on available water capacity (AWC), with more pronounced differences in sandy soils than in fine-textured soils. Libohova et al. (2018), however, evaluated the effect of SOC on AWC using the National Cooperative Soil Survey (NCSS) Soil Characterization Database and found that a 1 % increase in soil organic matter content increased AWC up to 1.5 % times its weight, depending on soil texture and clay mineralogy. Also, a global meta-analysis of 17 long-term field experiments conducted by Eden et al. (2017) found that plant available water increased significantly with the addition of organic material to the topsoil.
So far, only a few model-based analyses have explored the benefits of SOC increases for soil water availability systematically. Thereby, the assumption regarding SOC influences on soil hydraulic properties was based on evidence from pedotransfer functions (PTFs). Feng et al. (2022) applied the crop model APSIM at a regional scale in China to model yield variability of maize and identified a statistically significant relationship between SOC and the temperature sensitivity of maize yields, suggesting that SOC contributes to climate resilience. A different model-based study design was implemented by Bonfante et al. (2020), who applied the SWAP model (Kroes et al., 2017) to six different Italian soils with assumed increased soil organic matter up to 2 %–4 % in the topsoil. They found only minor increases in moisture supply capacity to be achieved with additional organic matter in the soil. In contrast to this, Ankenbauer and Loheide (2017), who applied a 1-D variably saturated groundwater flow model, found that increases in soil organic matter can contribute as much as 88 mm to transpiration or 35 additional water-stress-free days during a dry summer. Discrepancies in these studies' findings may be attributable to differences in pedo-climatic conditions, as well as to model setups and the chosen levels and depths of SOC increases.
A systematic analysis of the impacts of SOC increases on drought stress reduction depending on the depth of the SOC increase is lacking so far. It is thus the aim of this study to systematically evaluate and quantify the potential benefits of increasing SOC for drought limitations in a regional context not only under current conditions but also under projected future climatic conditions. As a study case, we chose to evaluate how changes in SOC at different depths affect the drought stress experienced by maize at the Swiss Central Plateau region, where agricultural land use dominates and for which region climate projections suggest a decrease in summer precipitation and an increase in winter precipitation as climate change progresses (CH2018, CH2018 Project Team, 2018). Annual precipitation sums are expected to remain largely the same over the projection period until the end of the century, ranging from 997 mm in the southwest to 1013 mm in the northeast. As previous studies have shown, drought stress is already limiting grain maize productivity under current conditions (Holzkämper et al., 2013, 2015b), and this limitation is expected to become more significant as climate change progresses. According to Holzkämper (2020), irrigation demands for grain maize might increase by up to 20 % by the end of this century in comparison with the reference period of 1981–2000, assuming that the duration of the growth season remains constant. If late-maturing varieties would be grown, given the possibility of an extended growth season with increasing temperatures, irrigation water demand may even increase by 40 % (Holzkämper, 2020). This raises concerns about the availability of irrigation water in the Swiss Central Plateau, where reoccurring irrigation bans have challenged farmers more and more frequently in recent drought years (BAFU, 2019, 2016). Solutions to make Swiss production systems less reliant on supplementary irrigation are urgently needed.
To systematically evaluate the benefits of increasing soil organic carbon (SOC) in terms of reducing drought limitations in a typical agricultural soil in the Swiss Central Plateau, we apply a field-scale agro-hydrological model that is deemed to be a suitable tool for interpreting interactions between crops and the environment (Maharjan et al., 2018). The soil component of the model was parameterized using a recently developed pedo-transfer function, and the model setup is validated against measurements of soil moisture dynamics in two lysimeters of a lysimeter station. Subsequently, the model is applied based on downscaled climate projection data in combination with scenarios of soil carbon increases.
2.1 Agro-hydrological modelling with SWAP
The Soil Water Atmosphere Plant model (SWAP, version 4.0.1) (Kroes et al., 2017) is a physically based agro-hydrological model that simulates the transport of water, solutes, and heat in the unsaturated (vadose) zone and, optionally, the upper part of the saturated (groundwater) zone, with the upper boundary condition defined by atmospheric conditions. Major arable crops and grasslands can be explicitly simulated in SWAP via incorporation of the WOFOST (WOrld FOod STudies, De Wit et al., 2019) model or by using a simple crop module.
In interaction with the crop development, the model simulates the heat and solute transport dynamics of variably saturated soils by employing the Richards equation in the vertical direction, including a sink term for root water uptake:
where C(h) (cm−1) is the specific water capacity, the derivative of the soil water retention function θ(h), which describes the relation between water content θ (cm3 cm−3) and soil water suction h (cm, defined as positive at unsaturated conditions); t (d) is time; K(h) (cm d−1) is the hydraulic conductivity as a function of h; z (cm) is the vertical spatial coordinate (negative downwards); and Sa(h) (d−1) is a sink term representing the rate of soil water extraction by plant roots.
The relationships θ(h) and K(h) are defined by the van Genuchten (1980)–Mualem (1976) (VGM) equations:
where θs and θr are the saturated and residual soil water content (cm3 cm−3); α (cm−1), n, m (), and l are empirical shape parameters; Ks is the saturated hydraulic conductivity (cm d−1); and the relative degree of saturation, Θ, is expressed as ).
In our study, the model used crop properties and atmospheric conditions on a daily basis to calculate the potential evapotranspiration based on the Penman–Monteith equation. Water stress was evaluated according to the reduction function by Hartge (1980), with the optimal root water uptake in the h ranges of −325.0 cm (h3H) or −600 cm (h3L) to −30 cm (h2), with oxygen stress linearly increasing for h higher than −15 cm (h1) and drought stress linearly increasing for h smaller than −8000 cm (h4). The crop growth module considers how the actual transpiration can be reduced by drought (too dry), αd(z); lack of oxygen (too wet), αo(z); or too-saline conditions (physiological drought), αs(z) – these factors are known to reduce crop growth. The actual root water flux, Sa(z) (d−1), is then a function of all considered stresses:
where Sp(z) is the potential root water extraction rate at a certain depth. The actual transpiration, Ta (cm d−1), is calculated by integrating the root water flux over the root zone:
where Droot is the root layer thickness (cm).
In our simulations, we did not consider stresses caused by saline conditions and focused on the drought-induced transpiration reduction (Treddry) as an indicator of drought stress during the cropping period.
2.2 Climate data of three distinct study sites from measured and projected scenarios
Typical Swiss agricultural conditions were evaluated at three distinct sites distributed along the Swiss Central Plateau (the main agricultural production zone in Switzerland): Nyon–Changins (CGI), Zurich–Reckenholz (REH), and Wynau (WYN). Measured climatic variables from meteorological stations were obtained from MeteoSwiss. Table 1 contains annual mean values of the meteorological variables required by SWAP, while Fig. 1 presents their seasonal variation. While the three sites have similar altitudes, on average, the CGI site has the driest and warmest climate, with higher solar radiation and wind speed. WYN is, on average, the wettest and coldest. In all sites, the rainfall is relatively well distributed during the year, with higher precipitation, temperature, and solar radiation in the summer season.
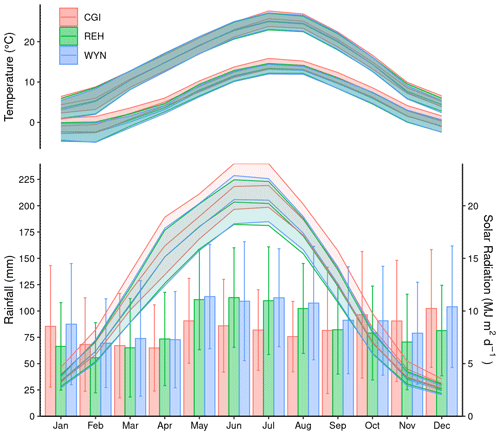
Figure 1Seasonal variability of climatic variables considering monthly mean ± standard deviation (shades and bars) values observed at the meteorological stations between 1981 and 2022. Rainfall corresponds to monthly sums, while other variables represent daily values averaged by month. Minimum (bottom lines) and maximum (upper lines) temperatures are presented.
Table 1Site description and climatic variables based on mean ± standard deviation values observed between 1981 and 2022 from MeteoSwiss.
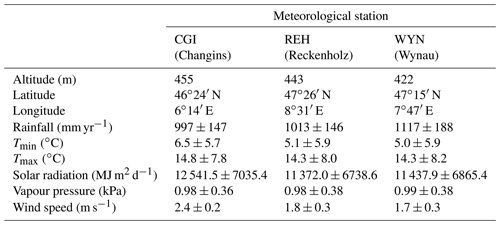
Future scenarios were evaluated using climate projections developed by the National Centre for Climate Services (NCCS) in Switzerland (CH2018 Project Team, 2018). The dataset contains transient daily time series for the period 1981–2099 for several variables at individual Swiss stations (daily–local), produced by applying a statistical downscaling and bias correction method (quantile mapping, QM) to the original output of all EURO-CORDEX climate model simulations employed in CH2018 (CH2018 Project Team, 2018). From all available projections with different representative concentration pathways (RCPs), we selected the ones that presented the dataset with all required input variables for SWAP, as listed in Table 1. In total, we used 22 projections for RCP8.5, 17 for RCP4.5, and 8 for RCP2.6. For more details about the selected model chains, see the Supplement (Sect. S1). Figure 2 presents an overview of the projected climate variables for the summer (JJA) and winter (DJF) months during the baseline (1981–2020), mid-century (2031–2060), and end-of-century (2081–2099) periods for each of the RCP8.5 projections. More details about the other RCPs can be found in the Supplement (Sect. S2). With the most pessimistic assumption about the evolution of greenhouse gas emissions (RCP8.5), climate projections estimate lower precipitation, higher temperature, and higher solar radiation for future summers, while they predict higher precipitation, higher temperature, and lower solar radiation for winters at the end of the century.
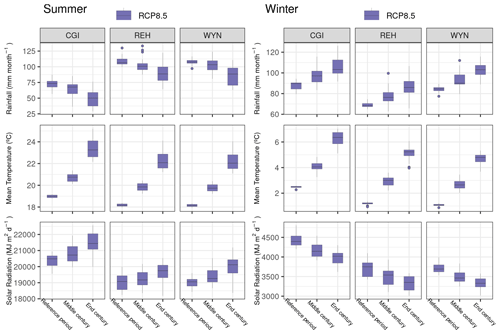
Figure 2Summary of climatic variables considering monthly mean values at the stations Changins (CGI), Reckenholz (REH), and Wynau (WYN) for the projections of RCP8.5. Summer was considered to be the months of June, July, and August, and winter corresponds to December, January, and February. Reference period: 1981–2020, mid-century: 2031–2060, end of century: 2071–2099. Rainfall corresponds to monthly sums, mean temperature is the mean between the maximum and minimum temperatures per day averaged by month, and solar radiation corresponds to daily values averaged by month.
2.3 Model reference data and setup
Reference information on soil water dynamics at four different depths (10, 30, 60, and 90 cm) was available from lysimeters of 135 cm depth and 1 m2 surface area at the lysimeter facility of Agroscope (Zurich–Reckenholz) (Prasuhn et al., 2016). Soil moisture was monitored from 2009 to 2022 using frequency domain reflectometry sensors (FDR; ThetaProbe ML2x, Delta-T Devices) at the depths of 10, 30, 60, and 90 cm. In each of the lysimeters, two identical sensors were installed at each depth with a time resolution of 1 h. We utilize the data of two lysimeters that contain similar soil monoliths from a typical agricultural soil nearby (loamy-silty cambisol above ground moraine (FAO, 2015); see Table 2 for the soil profile description). The monoliths have a 15 cm layer of purified quartz sand and gravel at the bottom, which helps facilitate free drainage.
Table 2Soil physical and chemical properties of the evaluated typical Swiss agricultural profile at the lysimeter facility at Agroscope Reckenholz. SOC: soil organic carbon, BD: bulk density, PD: particle density, CEC: cation exchange capacity. Soil class and horizon description according to Prasuhn et al. (2016).
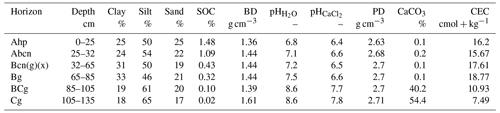
For the model setup, the measured physical and chemical soil parameters (Table 2) were used in combination with the pedotransfer function (PTF) developed by Szabó et al. (2021) using the R package in which the euptfv2 is implemented (Weber et al., 2020). The euptfv2 is a random-forest-based PTF with various options for input and output parameters, and it has proven to be one of the most accurate PTFs for estimating soil hydraulic properties for Europe when tested on diverse datasets (Nasta et al., 2021). As the standard setup for all simulations, we used option PTF02, which requires the depth of the soil layer, soil texture, and soil organic carbon content (SOC) as inputs and estimates the VGM parameters for the soil water retention (θ(h)) and hydraulic conductivity (K(h)) functions (Eq. 2).
Table 3 presents the parameters of Eq. (2) at the evaluated soil profile from the lysimeter station, calculated using the chosen PTF. The soil water retention and hydraulic conductivity curves are visualized in Fig. 3.
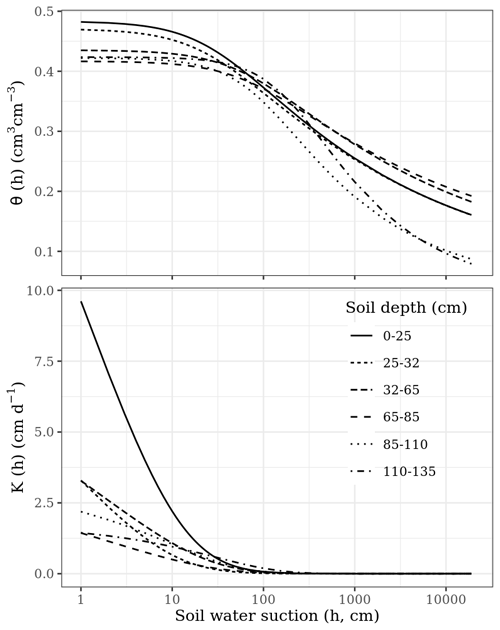
Figure 3Soil water retention (θ) and soil hydraulic conductivity (K) as functions of the soil water suction (h) at the evaluated soil profile estimated by the euptfv2 (option PTF02).
Table 3Soil hydraulic parameters calculated using the euptfv2 at the original soil profile considering option PTF02, which uses soil texture, soil carbon content, and soil depth as input.
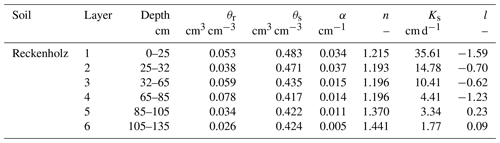
The validation of SWAP with the lysimeter information included three cropping periods with grain or silage maize in 2009, 2015, and 2020, with annual precipitation of 1018.9, 831.5 and 855.2 cm, respectively. A daily time step was adopted, and vertically, the top soil layer up to 65 cm was discretized using 1.0 cm sub-compartments, while subsequent layers were discretized with 5 and 10 cm sub-compartments. The boundary condition was set to free outflow at the soil–air interface, which is considered to be a valid option for lysimeters. The evapotranspiration was calculated using weather data and application of the Penman–Monteith equation. No macropore flow, lateral drainage, or solute dispersion was simulated. For the validation, the daily averaged values of measured soil water content at each replicate sensor and depth (eight time series per lysimeter) were compared to the modelled values by SWAP. As validation metrics, we used the root mean square error (RMSE) and the Pearson correlation (r). See Sect. S3 in the Supplement for details regarding the model setup.
2.4 Design of simulation experiments
In the absence of consistent and comparable data from long-term and holistic studies that account for the impacts of management on soil hydraulic properties, pedotransfer functions (PTFs) are seen as a suitable choice to systematically account for linkages between SOC and soil hydraulic properties. We thus used the chosen PTF to systematically capture secondary effects of SOC instead of directly inferring the effects of specific drivers of change on the soil hydraulic properties due to the uncertain interaction effects between SOC, soil type, climate, and management.
We assumed that management improvements have led to increased SOC from the beginning of the simulation period and that SOC remained stable over the simulation period, thereby testing different scenarios of successful carbon sequestration. The model parametrization included three distinct depth scenarios: (i) changes in SOC occur only within the top 0–25 cm, (ii) changes in SOC occur from 0 to 65 cm depth, and (iii) changes in SOC are achieved for the entire soil profile. In terms of SOC change, we simulated the addition of up to 4 % SOC to current SOC levels in 1 % increments in the (i) and (ii) depth scenarios, but we applied reduction factors of 0.8 and 0.6 for the 65–105 and 105–135 cm depths respectively in depth scenario (iii). This approach considers it to be that obtaining greater SOC via management likely affects the topsoil more than the deeper soil layers. The outlined depth and SOC level scenarios are listed in Table 4 for easier comprehension.
Table 4Description of %SOC levels added per depth and final values of SOC considering the described scenarios (i), (ii), and (iii). Bold values represent the layers where changes on SOC were applied.
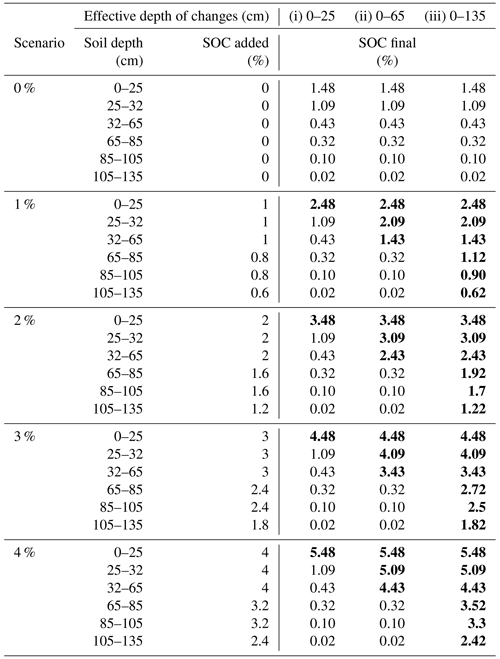
It should be emphasized that the levels of SOC in the soil are dependent on several factors including land use and management, climate, and geomorphology, which were considered as empirical relationships in this work.
To quantify the impacts of increasing SOC on drought stress in maize under climate change, SWAP was applied to the 22 climate projections at the three sites Changins (CGI), Reckenholz (REH), and Wynau (WYN) in combination with the scenarios of SOC increase listed in Table 4. We assumed grain maize to be sown on 6 May (DOY 126) and harvested on 17 October (DOY 290) as registered in the Swiss variety trial data for a medium–late variety type (Agroscope, 2023). The bottom boundary condition was set as free drainage, representing a soil profile with deep groundwater levels. For details of general SWAP parameterization, see Sect. S3.
All simulations considered rain-fed conditions and were performed using the simple crop growth module for a static crop, which simulates a fixed development of leaf area index and rooting depth, independent of climatic conditions, in order to keep the cropping period fixed for all scenarios. In this study, we worked with 165 d of crop-growing period; the crop component's parameterization is described in Sect. S4.
Overall, we conducted a total of 990 simulation runs (5 levels of SOC × 3 soil depths × 3 sites × 22 climate projections) for the period 1981–2099 and used cumulative amounts of drought-induced transpiration reduction (Treddry) as an indicator of drought stress during the cropping period. The 10-year moving average of Treddry was calculated to represent decadal changes and to exclude interannual variability. The range of Treddry values among the available climate projections were represented by the 0.05 quantile (q0.05) and the 0.95 quantile (q0.95) as upper and bottom boundaries respectively. The difference between management scenarios in terms of crop transpiration, defined as the average transpiration gain (ATG) with SOC increase, was calculated as the difference between the scenario with no addition of SOC (0 %) and the one with the maximum addition of SOC (4 %).
3.1 Model validation
Using the soil hydraulic parameters from Table 3, we simulated soil water content in the lysimeter soil profiles and compared them with moisture data measured by FDR sensors. The lumped values of the two lysimeters, considering all maize cropping periods (2009, 2015, and 2020) at all depths (10, 30, 60, and 90 cm) with duplicated sensors, resulted in a median (q0.5) RMSE of 0.066 cm3 cm−3 (q0.05=0.050 cm3 cm−3, q0.75=0.098) and a correlation median correlation r of 0.79 (q0.05=0.68, q0.75=0.84). In general, the simulations were more accurate for the deeper layers as compared to the topsoil. At 10 cm, the RMSE was, on average, 0.11 cm3 cm−3, whereas it was 0.04 cm3 cm−3 at the bottom.
3.2 Effect of increasing SOC on the soil hydraulic properties and soil water balance
The effects of adding different amounts of SOC at different soil layers (Fig. 4) are reflected in PTF estimates of soil hydraulic properties with updated SOC contents. The 0 % line, corresponding to the VGM parameters in Table 3, represents the properties of the different soil layers with current SOC. For the soil water retention curve, the effects of the increase in SOC reflected an estimated increase in pore space, whose expression varied with soil depth and added SOC. In the topsoil, the differences between the addition of 1 % and 4 % SOC were not as remarkable as in the subsoil layers, where an addition of 1 % SOC lead to a substantial increase in estimated pore space. For saturated hydraulic conductivity, the overall trend was a reduction in conductivity with the increase in SOC, with the biggest contrasts found in the topsoil.
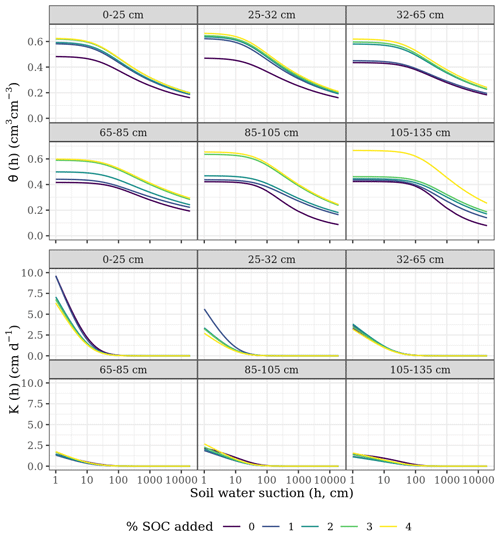
Figure 4Effects of SOC increase on the soil water retention (θ) and soil hydraulic conductivity (K) as functions of the soil water suction (h) as predicted by euptfv2, option PTF02.
Considering the effects of adding SOC at different soil depths, Fig. 5 presents an overview of the transient simulations between 1980 and 2099 with the most unfavourable climate scenario projections (RCP8.5). For each year of simulation, a range of values of Treddry was generated by the 22 climate projections, which are represented by a band defined by the q0.05 and q0.95 quantiles, and the q0.5 quantile (median) is represented by a line within that band. The average transpiration gain (ATG) line is the difference between the median (q0.5) values of the original Reckenholz soil profile (i.e. 0 % SOC addition) and the one that had 4 % SOC added. The ATG can be interpreted as the amount of seasonal transpiration gained in response to increased SOC. The absolute increase in Treddry when comparing the reference period with the end of the century was, on average, 269, 207, and 269 mm at CGI, REH, and WYN, respectively. Additional results considering other representative climate projections (RCP 2.6 and 4.5) are presented in the Supplement (Sect. S5).
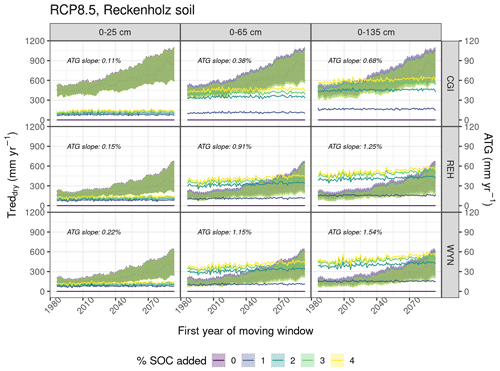
Figure 5Transpiration reduction due to drought stress (Treddry) (left axis, green band) for actual and future climate conditions considering different levels of SOC increase in the soil at different effective soil depths and average transpiration gain, ATG, (right axis, coloured lines) between 0 % and 4 % addition of SOC. Climate projections considered the RCP8.5 pathway and were averaged for every 10 years. The green shaded area of Treddry refers to the values between the (dotted) quantiles q0.05 and q0.95 of the climate projections. ATG is interpretable as average seasonal gain in transpiration due to SOC increase, and ATG slope refers to the slope of the ATG line between 0 % and 4 % SOC addition.
According to the simulated scenarios, the main driver of the absolute values of Treddry is the climate, with more drought stress under the climate of the drier site (CGI) and very similar stress levels under the climate of the other two sites, REH and WYN, that are wetter and appear to resemble each other somewhat. There was a clear tendency of increased stress towards the end of the century, driven by more unfavourable climatic conditions during the cropping period (Fig. 2). The ATGs were very similar amongst the three considered climates, with maximum values around 60 mm yr−1, and values slightly higher in the CGI climate. The ATG slopes calculated between the beginning and the end of the century were higher at REH and WYN, which are the sites with less water stress under current conditions. This is an implication of not considering a gradual build-up period for increased SOC but considering the same levels of SOC addition for the entire simulation period.
The simulations were performed considering the addition of SOC down to three different depths (25, 65, and 135 cm). The addition of SOC to the top 25 cm seems to have a modest effect on Treddry. The effects of increasing SOC all the way to 135 cm are the greatest but are comparable to the intermediate option of adding SOC till 65 cm depth. In general, adding 2 % SOC already lead to considerable reduction in Treddry and is a more realistic, easier-to-implement alternative to adding 4 % SOC.
3.3 Detailed soil water dynamics and drought stress over the cropping period
Figure 6 depicts, as an example, how the soil moisture profile develops and how the ATG in moisture deficit builds up during the simulated 2015 cropping year at the REH site, with the different increased SOC levels in the entire soil profile (depth scenario iii). The addition of SOC leads to a clear pattern of increasing soil water retention. The blue line depicts the daily simulated crop transpiration deficit (Treddry) of the 0 % added SOC scenario, while the black lines depict the same obtained for the relevant depth and SOC addition scenario in each plot. Their difference, when cumulated for the year, yields the transpiration deficit ATG for the given year and scenario. The most remarkable seasonal ATGs were observed in the beginning of the cropping season and could be linked to increased soil water retention capacity combined with the availability of water in that season. According to Fig. 4, increased SOC content generally yielded increased soil water retention capacity relative to the base scenario of no SOC addition. In the early cropping season, this increased capacity is capitalized on in the form of retaining more water in the system by the end of the recharge period in the wet and cold winter and spring seasons. The simulated extra amount of water is clearly demonstrated in Fig. 6. During the early part of the growing season, this excess water then becomes available to the crop, dampening the effects of any drought stress or at least delaying its onset. The soil will also not dry out to the same degree during the later half of the season or, at least, not to the same depth. Similar results for the other evaluated sites are presented in the Supplement (Sect. S6).
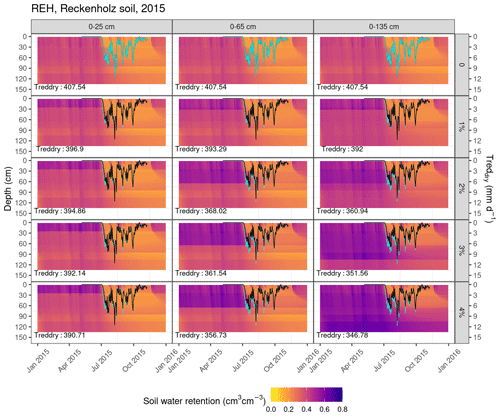
Figure 6Detailed profile of soil water content (left axis) and Treddry (right axis, black lines) according to the different added SOC levels at the Reckenholz site (REH) in the year of 2015. The blue line represents Treddry for the original soil profile (0 % SOC). When cumulated for the year, their difference yields the annual ATG in crop transpiration deficit that is due to the addition of carbon to the soil.
4.1 Increasing soil organic carbon reduces drought stress in maize
We observed that, according to the predictions of the used PTF, an increase in SOC has a small effect on (but generally decreases) soil hydraulic conductivity (Fig. 4). This may be counter-intuitive in that textbook knowledge connects greater SOC content with better soil structure formation; greater porosity; and, in turn, enhanced water transport properties (hydraulic conductivity) (Nemes et al., 2005). However, several studies have now emerged that correlated greater SOC content with lower hydraulic conductivity. These studies include both experimental data and the mining of several extended databases using machine learning (Nemes et al., 2005; Wang et al., 2009; Jarvis et al., 2013; Larsbo et al., 2016). The rationale behind this notion is that, when SOC content increases, there is enhanced porosity, but the tortuosity of conductive pathways may increase due to enhanced microbial activity and the formation of more complex aggregates, resulting in better water retention but reduced hydraulic conductivity. Some of these authors noted increased predicted water retention in the effective porosity (i.e. the range between field capacity and saturation), which supports the proposed notion.
Results from this simulation study suggest that increases in SOC would generally decrease drought stress in maize cultivated in a typical agricultural soil in Switzerland. The summer season precipitation amount at the evaluated sites is expected to be decreased by around 60–65 mm till the end of the century (Fig. 2). In this scenario, a 2 % addition of SOC can reduce the drought stress of maize by 10.5 to 40 mm during the cropping season and potentially compensate for part of the rainfall reduction with climate change. Bonfante et al. (2020) suggest that the effect of SOC on moisture supply capacity should be evaluated in more climatic zones in order to obtain a broader picture of its potential impact. What we observed in this work was that the degree of decrease in Treddry was only minimally dependent on regional climatic conditions, with the wettest site (WYN) benefitting least from the SOC increases under current climate conditions. As conditions get drier, as projected with climate change for the Swiss Central Plateau, the transpiration gain increases but reaches a maximum at 60 mm with SOC increases down to 135 cm.
Our study suggests minor benefits of increasing SOC in the topsoil (maximum ATG reached is 15 mm, Fig. 5). However, if SOC was increased down to at least 65 cm, this beneficial effect can be considerably higher (maximum ATG reached is 45 mm, Fig. 5). Overall, the maximum ATG of Treddry quantified in this study was 60 mm (at the end of the century, with SOC increase down to 135 cm), suggesting that, without supplementary irrigation, seasonal crop transpiration can be up to 60 mm greater with increased SOC compared to the reference situation. This amount is comparable to 1–2 irrigation dosages and makes up for roughly 30 % of the average theoretical irrigation water demand estimated by Holzkämper (2020) for the region between Wynau (WYN) and Changins (CGI). The productivity gain to be achieved will strongly depend on the period in the cropping cycle when this extra water will be available. Considering that transpiration benefits are greatest at the onset of drought during early summer (Fig. 6), the productivity gains may be particularly high if the effect coincides with the critical reproductive phase of the crop. This might imply that transpiration gains achieved with increases in SOC have a significant potential to increase yield stability, particularly in situations where and when irrigation is not an option.
The positive slopes of calculated ATGs of Treddry (i.e. transpiration gained with SOC increase) in Fig. 5 suggest that the benefits of SOC additions could slightly increase with projected future climate change – especially at WYN, the least water-limited site under current conditions. At the driest site, CGI, the ATG (i.e. benefit of SOC increase) reached under current climatic conditions is roughly at the same level as it is at WYN at the end of the century. These findings imply that the benefits of SOC accumulation may increase as water input (precipitation) during the cropping period decreases over time. However, there appears to be a threshold beyond which benefits are not seen as Treddry further increases (the ATG slope in Fig. 5 decreases from the wettest to the driest site). The benefit of extra water availability comes from the balance of two elements: available water and available storage capacity. It appears that the available storage capacity component is enhanced by the addition of some SOC (i.e. 2 % addition in our simulations), but the system becomes water-limited by the end of the century. The extra storage capacity that additional SOC may yield will not be filled up by the actual water input, and the potential extra benefit cannot be realized. The within-year occurrence of the same phenomenon is observable in Fig. 6. The biggest reduction in Treddry occurs in the beginning of the cropping season, when the increased retention capacity was present at the same time as when an ample amount of water was recharging the system during and after the cold, rainy season with little or no plant water uptake.
A similar balance is likely to apply when the outcome of a 135 cm deep application of added SOC is interpreted. When simulating SOC addition to 135 cm depth vs. only 65 cm, the added benefit in terms of reduced crop Treddry appears to be limited. We argue that, while some excess water storage capacity is simulated, there is little actual benefit realized from that given the reduced amount of predicted precipitation by the end of the century. In addition, few, if any, crop roots reach that depth, which means that the only way the crop has a direct benefit from water stored in the deeper soil layers in the growing season is if water is redistributed upwards via capillary and vapour transport.
4.2 Possibilities to increase soil organic carbon
Results from our study suggest that the beneficial effects of increasing SOC are small if SOC is only increased in the top soil (0–25 cm), but these become more significant if SOC is increased to only 65 cm depth by at least 2 %. We assumed that such SOC increases can be achieved, while different management adaptations and combinations thereof may be suitable to reach this target. Commonly considered strategies to increase SOC include additions of organic amendments, planting of deep-rooting crops, cover-cropping, intercropping, mulching with organic material, retaining crop residues in the field, and reduced tillage or no till (Topa et al., 2021; IPCC, 2022). No till or reduced tillage decreases the carbon oxidation process and soil disturbance with the loss of soil organic carbon and nutrient availability (Modak et al., 2019; Kan et al., 2020). Also, Angers and Eriksen-Hamel (2008) found that tillage affects the distribution of SOC over the depth of the soil profile with important implications in crop water availability. A meta-analysis on the effects of tillage on SOC (Krauss et al., 2022) has shown that it is not uncommon that depletion in SOC of a subsoil layer co-occurs with increased SOC levels in the topsoil. We tested this with the particular soil and PTF used in our study and found that the hydrological effects of reducing SOC at the depth of 25–32 cm were almost identical to the scenario in which the same amount of SOC increase at the depths of 0–25 cm was simulated but without subsoil SOC depletion (Fig. 5). We emphasize that, from the point of view of water availability to plants with deep roots, management strategies should aim to increase SOC content deeper than only in the topsoil.
According to Bai et al. (2019), reduced tillage or no till increases SOC mostly in the top 10 cm and also in the subsoil below 50 cm. The same study found that cover-cropping could increase SOC down to 70 cm depth. Incorporation of perennial grasses into crop rotations could help increase SOC to 60 cm depth, beyond the plough layer (Carter and Gregorich, 2010). Evidence of this under Swiss conditions was provided by Guillaume et al. (2021, 2022). Overall, such strategies were found to be most beneficial to SOC accumulation near the soil surface (Bai et al., 2019). One management operation that could effectively contribute to an accumulation of SOC in deeper soil layers is deep ploughing (Alcántara et al., 2016). However, when the soil is loosened. the SOC oxidation process is enhanced, and erosion may be triggered, which has to be accounted for when planning such interventions.
We have tested the scenario of incorporating extra amounts of SOC in the soil down to a depth of 135 cm. This is a scenario that would require similar strategies as the previously discussed scenario, but it is likely rather difficult to implement, especially with greater amounts of SOC stored. Our study showed that, in terms of water availability to the (maize) crop, this scenario has little extra benefit to offer over the scenario of having extra SOC sequestered to 65 cm depth. Hence, any investment in sequestering SOC into such depths should not be driven by a high expectation of hydrological benefits.
4.3 Limitations and further work
Our study, as well as previous modelling studies exploring the impacts of SOC additions on soil water availability (e.g. Ankenbauer and Loheide, 2017; Bonfante et al., 2020; Feng et al., 2022), builds on pedo-transfer functions that are believed to be best in estimating soil hydraulic parameters for the study area based on levels of SOC and other soil properties. The selection of PTFs, however, may play a crucial role in the outcome of simulated scenarios. While recent studies confirm the validity of the equations used (e.g. Nasta et al., 2021; Wagner et al., 2004), uncertainties in derived estimates may still be large (Fatichi et al., 2020). PTF structure may also have an influence in that more advanced (a.k.a. better) PTFs are usually products of refined machine learning algorithms that may produce strong results in general but may have different estimation qualities in different parts of the data domain. Since such local performance is rarely evaluated, future work should thus explore the sensitivity of SOC benefits via using an ensemble of PTFs. Moreover, measurements of soil hydraulic properties in combination with SOC, texture, and bulk density in long-term field trials investigating management alternatives affecting SOC would provide very useful evidence to help disentangle the effects of land use and management on the relationships between soil texture and hydraulic properties. By integrating management and also local climate information in PTFs, their uncertainties in predicting soil hydraulic properties in specific context could be reduced (Van Looy et al., 2017). Many historic records do not provide sufficient information on how certain measurements were performed, or when the samples were taken. Also, the timing of field sampling is likely to play a role here as it is known that soil hydraulic properties vary in time and are influenced e.g. by precipitation regime or land use and management (Caplan et al., 2019; Lu et al., 2020).
In this study, we focused on transpiration reduction, which is likely to imply biomass reduction but may not necessarily imply yield reduction – depending on the timing of water stress. Other studies have investigated the impacts of CC on yields for grain maize in Switzerland (Holzkämper, 2020; Holzkämper et al., 2015a), and it was found that yield trends differ depending on the choice of varieties assumed to be planted. In our study here, we focus on drought impacts on crop transpiration alone. Subsequent yield formation will be affected by crop transpiration but also by various other drivers (e.g. temperature and radiation limitations, timing of stresses, heat stress). In order to obtain a clearer view on the impacts of SOC increases on crop transpiration, we elected not to consider the multitude of such interactive effects in the presented study. In future work, it will be interesting to explore possibilities to further increase the benefits of SOC additions by combining that strategy with other adaptations of crop and soil management (e.g. earlier maturing varieties, cover-cropping, mulching of soil to reduce evaporation). In this context, it will be advisable to also account for a dynamic development of phenology and thus leaf area index to account for possible interactions between crop growth and soil moisture conditions.
While our study focused solely on the impacts of SOC additions on soil water dynamics, SOC increases could have additional benefits for crop productivity and yield stability by feeding and supporting beneficial microbial communities in the soil (e.g. rhizobacteria, nitrogen-fixing bacteria, and mycorrhizal fungi), which increase the crops' ability to take up water and nutrients (Coban et al., 2022; Renwick et al., 2021; Kallenbach and Grandy, 2011). Such aspects could be addressed in future field experimental studies. Beyond that, future field- and model-based studies may also evaluate trade-offs or synergies of SOC, promoting management strategies with regard to other soil-related ecosystem service indicators such as nitrate leaching, soil loss, or runoff generation to provide insights regarding the possibilities to increase the sustainability of agricultural production overall (Bonfante et al., 2019). Alternative modelling approaches considering dynamic changes in soil hydraulic properties could also be applied in the future to investigate the influence of soil structural dynamics on the adaptation benefits of SOC accumulation (e.g. based on Meurer et al., 2020a, b) as, to our understanding, current models do not facilitate the representation of soil as a temporally variable medium.
Our study is the first to investigate the possibilities of reducing Treddry, an indicator of drought stress, in maize cultivated in the Swiss Central Plateau through increasing SOC in the topsoil and subsoil. Our simulations showed that Treddry in maize is expected to increase with climate change in the Swiss Central Plateau region by around 60–65 mm irrespective of SOC increase. Increasing SOC in a typical agricultural soil in Switzerland, however, is beneficial to reduce drought limitations in maize, shown by consistently positive average transpiration gains. These benefits are minimal if SOC is only increased in the top 25 cm but become considerable if SOC is increased down to 65 or 135 cm depth. With a 2 % addition of SOC down to 65 cm depth, a considerable average transpiration gain of 40 mm can be reached. This scenario can be achievable considering management adaptations such as cover cropping or compost applications. It appears that a greater or deeper SOC addition would not return substantial extra benefits in terms of offsetting more crop drought stress rooting in the changing climate.
The source code of the SWAP model can be downloaded at https://www.swap.alterra.nl/ (University of Wageningen, 2023). Data evaluation scripts are included in the repository at https://doi.org/10.5281/zenodo.10068907 (Turek and Holzkämper, 2023).
The supplement related to this article is available online at: https://doi.org/10.5194/soil-9-545-2023-supplement.
Conceptualization: MET, AH. Data curation: MET. Formal analysis: MET, AH. Funding acquisition: AH. Investigation: MET, AN, AH. Methodology: MET, AH. Resources: AH. Software: MET. Visualization: MET. Writing – original draft preparation: MET, AH. Writing – review and editing: MET, AN, AH.
The contact author has declared that none of the authors has any competing interests.
Publisher’s note: Copernicus Publications remains neutral with regard to jurisdictional claims made in the text, published maps, institutional affiliations, or any other geographical representation in this paper. While Copernicus Publications makes every effort to include appropriate place names, the final responsibility lies with the authors.
This project was developed in the framework of the OPTAIN and SoilX-EJP SOIL projects. OPTAIN (OPtimal strategies to retAIN and re-use water and nutrients in small agricultural catchments across different soil-climatic regions in Europe, https://cordis.europa.eu, last access: 1 November 2023) has received funding from the European Union's Horizon 2020 research and innovation programme (grant agreement no. 862756). SoilX is part of the European Joint Program for SOIL “Towards climate-smart sustainable management of agricultural soils” (EJP SOIL) funded by the European Union Horizon 2020 research and innovation programme (grant agreement no. 862695). The authors thank Volker Prasuhn for providing full assistance with the lysimeter data.
This research has been supported by the Horizon 2020 Framework Programme (grant nos. 862756 and 862695).
This paper was edited by David Dunkerley and reviewed by two anonymous referees.
Agroscope: Sortenversuche – Resultate Mais, https://www.agroscope.admin.ch/agroscope/de/home/themen/pflanzenbau/ackerbau/kulturarten/mais/sortenversuche-resultate.html (last access: 7 November 2023), 2023 (in German).
Alcántara, V., Don, A., Well, R., and Nieder, R.: Deep ploughing increases agricultural soil organic matter stocks, Glob. Change Biol., 22, 2939–2956, https://doi.org/10.1111/gcb.13289, 2016.
Angers, D. A. and Eriksen-Hamel, N. S.: Full-Inversion Tillage and Organic Carbon Distribution in Soil Profiles: A Meta-Analysis, Soil Sci. Soc. Am. J., 72, 1370–1374, https://doi.org/10.2136/sssaj2007.0342, 2008.
Ankenbauer, K. J. and Loheide, S. P.: The effects of soil organic matter on soil water retention and plant water use in a meadow of the Sierra Nevada, CA, Hydrol. Process., 31, 891–901, https://doi.org/10.1002/hyp.11070, 2017.
Arthur, E., Tuller, M., Moldrup, P., and de Jonge, L. W.: Effects of biochar and manure amendments on water vapor sorption in a sandy loam soil, Geoderma, 243–244, 175–182, https://doi.org/10.1016/j.geoderma.2015.01.001, 2015.
BAFU: Hitze und Trockenheit im Sommer 2015, Auswirkungen auf Mensch und Umwelt, Bern, BAFU, https://www.bafu.admin.ch/bafu/de/home/themen/klima/publikationen-studien/publikationen/hitze-und-trockenheit.html (last access: 1 November 2023), 2016.
BAFU: Hitze und Trockenheit im Sommer 2018, Bern, BAFU, https://www.bafu.admin.ch/bafu/de/home/themen/klima/publikationen-studien/publikationen/hitze-und-trockenheit.html (last access: 1 November 2023), 91 pp., 2019.
Bai, X., Huang, Y., Ren, W., Coyne, M., Jacinthe, P.-A., Tao, B., Hui, D., Yang, J., and Matocha, C.: Responses of soil carbon sequestration to climate-smart agriculture practices: A meta-analysis, Glob. Change Biol., 25, 2591–2606, https://doi.org/10.1111/gcb.14658, 2019.
Blanchy, G., Bragato, G., Di Bene, C., Jarvis, N., Larsbo, M., Meurer, K., and Garré, S.: Soil and crop management practices and the water regulation functions of soils: a qualitative synthesis of meta-analyses relevant to European agriculture, SOIL, 9, 1–20, https://doi.org/10.5194/soil-9-1-2023, 2023.
Bonfante, A., Terribile, F., and Bouma, J.: Refining physical aspects of soil quality and soil health when exploring the effects of soil degradation and climate change on biomass production: an Italian case study, SOIL, 5, 1–14, https://doi.org/10.5194/soil-5-1-2019, 2019.
Bonfante, A., Basile, A., and Bouma, J.: Exploring the effect of varying soil organic matter contents on current and future moisture supply capacities of six Italian soils, Geoderma, 361, 114079, https://doi.org/10.1016/j.geoderma.2019.114079, 2020.
Caplan, J. S., Giménez, D., Hirmas, D. R., Brunsell, N. A., Blair, J. M., and Knapp, A. K.: Decadal-scale shifts in soil hydraulic propertiesas induced by altered precipitation, Sci. Adv., 5, eaau6635, https://doi.org/10.1126/sciadv.aau6635, 2019.
Carter, M. R. and Gregorich, E. G.: Carbon and nitrogen storage by deep-rooted tall fescue (Lolium arundinaceum) in the surface and subsurface soil of a fine sandy loam in eastern Canada, Agr. Ecosyst. Environ., 136, 125–132, https://doi.org/10.1016/j.agee.2009.12.005, 2010.
CH2018 Project Team: CH2018 – Climate Scenarios for Switzerland, National Centre for Climate Services, https://doi.org/10.18751/Climate/Scenarios/CH2018/1.0, 2018.
Coban, O., De Deyn, G. B., and van der Ploeg, M.: Soil microbiota as game-changers in restoration of degraded lands, Science, 375, abe0725, https://doi.org/10.1126/science.abe0725, 2022.
Crystal-Ornelas, R., Thapa, R., and Tully, K. L.: Soil organic carbon is affected by organic amendments, conservation tillage, and cover cropping in organic farming systems: A meta-analysis, Agr. Ecosyst. Environ., 312, 107356, https://doi.org/10.1016/j.agee.2021.107356, 2021.
de Wit, A., Boogaard, H., Fumagalli, D., Janssen, S., Knapen, R., van Kraalingen, D., Supit, I., van der Wijngaart, R., and van Diepen, K.: 25 years of the WOFOST cropping systems model, Agr. Syst., 168, 154–167, https://doi.org/10.1016/j.agsy.2018.06.018, 2019.
Edeh, I. G., Mašek, O., and Buss, W.: A meta-analysis on biochar's effects on soil water properties – New insights and future research challenges, Sci. Total Environ., 714, 136857, https://doi.org/10.1016/j.scitotenv.2020.136857, 2020.
Eden, M., Gerke, H. H., and Houot, S.: Organic waste recycling in agriculture and related effects on soil water retention and plant available water: a review, Agron. Sustain. Dev., 37, 11, https://doi.org/10.1007/s13593-017-0419-9, 2017.
FAO: World reference base for soil resources 2014, International soil classification system for naming soils and creating legends for soil maps, edited by: Schad, P., van Huyssteen, C., and Michéli, E., FAO, ISBN 978-92-5-108369-7, 2015.
Fatichi, S., Or, D., Walko, R., Vereecken, H., Young, M. H., Ghezzehei, T. A., Hengl, T., Kollet, S., Agam, N., and Avissar, R.: Soil structure is an important omission in Earth System Models, Nat. Commun., 11, 522, https://doi.org/10.1038/s41467-020-14411-z, 2020.
Feng, P., Wang, B., Harrison, M. T., Wang, J., Liu, K., Huang, M., Liu, D. L., Yu, Q., and Hu, K.: Soil properties resulting in superior maize yields upon climate warming, Agron. Sustain. Dev., 42, 85, https://doi.org/10.1007/s13593-022-00818-z, 2022.
Guillaume, T., Bragazza, L., Levasseur, C., Libohova, Z., and Sinaj, S.: Long-term soil organic carbon dynamics in temperate cropland-grassland systems, Agr. Ecosyst. Environ., 305, 107184, https://doi.org/10.1016/j.agee.2020.107184, 2021.
Guillaume, T., Makowski, D., Libohova, Z., Bragazza, L., Sallaku, F., and Sinaj, S.: Soil organic carbon saturation in cropland-grassland systems: Storage potential and soil quality, Geoderma, 406, 115529, https://doi.org/10.1016/j.geoderma.2021.115529, 2022.
Hartge, K. H.: Feddes, R. A., Kowalik, P. I. und Zaradny, H.: simulation of field water use and crop yield. Pudoc (Centre for agricultural publishing and documentation) Wageningen, Niederlande, 195 Seiten, 13 Abbildungen, Paperback. Preis: hfl 30, Z. Pflanzenernaehr. Bodenk., 143, 254–255, https://doi.org/10.1002/jpln.19801430219, 1980.
Holzkämper, A.: Varietal adaptations matter for agricultural water use – a simulation study on grain maize in Western Switzerland, Agr. Water Manage., 237, 106202, https://doi.org/10.1016/j.agwat.2020.106202, 2020.
Holzkämper, A., Calanca, P., and Fuhrer, J.: Identifying climatic limitations to grain maize yield potentials using a suitability evaluation approach, Agr. Forest Meteorol., 168, 149–159, https://doi.org/10.1016/j.agrformet.2012.09.004, 2013.
Holzkämper, A., Calanca, P., Honti, M., and Fuhrer, J.: Projecting climate change impacts on grain maize based on three different crop model approaches, Agr. Forest Meteorol., 214–215, 219–230, https://doi.org/10.1016/j.agrformet.2015.08.263, 2015a.
Holzkämper, A., Fossati, D., Hiltbrunner, J., and Fuhrer, J.: Spatial and temporal trends in agro-climatic limitations to production potentials for grain maize and winter wheat in Switzerland, Reg. Environ. Change, 15, 109–122, https://doi.org/10.1007/s10113-014-0627-7, 2015b.
IPCC: Technical Summary, in: Climate Change and Land: an IPCC special report on climate change, desertification, land degradation, sustainable land management, food security, and greenhouse gas fluxes in terrestrial ecosystems, edited by: Shukla, P. R., Skea, J., Buendia, E. C., Masson-Delmotte, V., Pörtner, H.-O., Roberts, D. C., Zhai, P., Slade, R., Connors, S., Diemen, R. v., Ferrat, M., Haughey, E., Luz, S., Neogi, S., Pathak, M., Petzold, J., Pereira, J. P., Vyas, P., Huntley, E., Kissick, K., Belkacemi, M., and Malley, J., Cambridge University Press, https://doi.org/10.1017/9781009157988.002, 2022.
Jarvis, N., Koestel, J., Messing, I., Moeys, J., and Lindahl, A.: Influence of soil, land use and climatic factors on the hydraulic conductivity of soil, Hydrol. Earth Syst. Sci., 17, 5185–5195, https://doi.org/10.5194/hess-17-5185-2013, 2013.
Kallenbach, C. and Grandy, A. S.: Controls over soil microbial biomass responses to carbon amendments in agricultural systems: A meta-analysis, Agr. Ecosyst. Environ., 144, 241–252, https://doi.org/10.1016/j.agee.2011.08.020, 2011.
Kan, Z.-R., Ma, S.-T., Liu, Q.-Y., Liu, B.-Y., Virk, A. L., Qi, J.-Y., Zhao, X., Lal, R., and Zhang, H.-L.: Carbon sequestration and mineralization in soil aggregates under long-term conservation tillage in the North China Plain, CATENA, 188, 104428, https://doi.org/10.1016/j.catena.2019.104428, 2020.
Krauss, M., Wiesmeier, M., Don, A., Cuperus, F., Gattinger, A., Gruber, S., Haagsma, W. K., Peigné, J., Palazzoli, M. C., Schulz, F., van der Heijden, M. G. A., Vincent-Caboud, L., Wittwer, R. A., Zikeli, S., and Steffens, M.: Reduced tillage in organic farming affects soil organic carbon stocks in temperate Europe, Soil Till. Res., 216, 105262, https://doi.org/10.1016/j.still.2021.105262, 2022.
Kroes, J. G., Dam, J. C. V., Bartholomeus, R. P., Groenendijk, P., Heinen, M., Hendriks, R. F. A., Mulder, H. M., Supit, I., and Walsum, P. E. V. V.: SWAP version 4 Theory description and user manual, Report 2780, Wageningen Environmental Research, https://doi.org/10.18174/416321, 2017.
Lal, R.: World cropland soils as a source or sink for atmospheric carbon, in: Advances in Agronomy, Academic Press, 71, 145–191, https://doi.org/10.1016/S0065-2113(01)71014-0, 2001.
Lal, R.: Soil carbon sequestration to mitigate climate change, Geoderma, 123, 1–22, https://doi.org/10.1016/j.geoderma.2004.01.032, 2004.
Larsbo, M., Koestel, J., Kätterer, T., and Jarvis, N.: Preferential Transport in Macropores is Reduced by Soil Organic Carbon, Vadose Zone J., 15, 1–7, https://doi.org/10.2136/vzj2016.03.0021, 2016.
Libohova, Z., Seybold, C., Wysocki, D., Wills, S., Schoeneberger, P., Williams, C., Lindbo, D., Stott, D., and Owens, P. R.: Reevaluating the effects of soil organic matter and other properties on available water-holding capacity using the National Cooperative Soil Survey Characterization Database, J. Soil Water Conserv., 73, 411–421, https://doi.org/10.2489/jswc.73.4.411, 2018.
Liu, S., Lei, Y., Zhao, J., Yu, S., and Wang, L.: Research on ecosystem services of water conservation and soil retention: a bibliometric analysis, Environ. Sci. Pollut. R., 28, 2995–3007, https://doi.org/10.1007/s11356-020-10712-4, 2021.
Lu, J., Zhang, Q., Werner, A. D., Li, Y., Jiang, S., and Tan, Z.: Root-induced changes of soil hydraulic properties – A review, J. Hydrol., 589, 125203, https://doi.org/10.1016/j.jhydrol.2020.125203, 2020.
Maharjan, G. R., Prescher, A.-K., Nendel, C., Ewert, F., Mboh, C. M., Gaiser, T., and Seidel, S. J.: Approaches to model the impact of tillage implements on soil physical and nutrient properties in different agro-ecosystem models, Soil Till. Res., 180, 210–221, https://doi.org/10.1016/j.still.2018.03.009, 2018.
Meurer, K. H. E., Chenu, C., Coucheney, E., Herrmann, A. M., Keller, T., Kätterer, T., Nimblad Svensson, D., and Jarvis, N.: Modelling dynamic interactions between soil structure and the storage and turnover of soil organic matter, Biogeosciences, 17, 5025–5042, https://doi.org/10.5194/bg-17-5025-2020, 2020a.
Meurer, K. H. E., Barron, J., Chenu, C., Coucheney, E., Fielding, M., Hallett, P., Herrmann, A. M., Keller, T., Koestel, J., Larsbo, M., Lewan, E., Or, D., Parsons, D., Parvin, N., Taylor, A., Vereecken, H., and Jarvis, N.: A framework for modelling soil structure dynamics induced by biological activity, Glob. Change Biol., 26, 5382–5403, https://doi.org/10.1111/gcb.15289, 2020b.
Minasny, B. and McBratney, A. B.: Limited effect of organic matter on soil available water capacity, Eur. J. Soil Sci., 69, 39–47, https://doi.org/10.1111/ejss.12475, 2017.
Minasny, B., Malone, B. P., McBratney, A. B., Angers, D. A., Arrouays, D., Chambers, A., Chaplot, V., Chen, Z.-S., Cheng, K., Das, B. S., Field, D. J., Gimona, A., Hedley, C. B., Hong, S. Y., Mandal, B., Marchant, B. P., Martin, M., McConkey, B. G., Mulder, V. L., O'Rourke, S., Richer-de-Forges, A. C., Odeh, I., Padarian, J., Paustian, K., Pan, G., Poggio, L., Savin, I., Stolbovoy, V., Stockmann, U., Sulaeman, Y., Tsui, C.-C., Vågen, T.-G., van Wesemael, B., and Winowiecki, L.: Soil carbon 4 per mille, Geoderma, 292, 59–86, https://doi.org/10.1016/j.geoderma.2017.01.002, 2017.
Modak, K., Ghosh, A., Bhattacharyya, R., Biswas, D. R., Das, T. K., Das, S., and Singh, G.: Response of oxidative stability of aggregate-associated soil organic carbon and deep soil carbon sequestration to zero-tillage in subtropical India, Soil Till. Res., 195, 104370, https://doi.org/10.1016/j.still.2019.104370, 2019.
Mualem, Y.: A new model for predicting the hydraulic conductivity of unsaturated porous media, Water Resour. Res., 12, 513–522, https://doi.org/10.1029/WR012i003p00513, 1976.
Murphy, B.: Key soil functional properties affected by soil organic matter – evidence from published literature, IOP C. Ser. Earth Env., 25, 012008, https://doi.org/10.1088/1755-1315/25/1/012008, 2015.
Nasta, P., Szabó, B., and Romano, N.: Evaluation of pedotransfer functions for predicting soil hydraulic properties: A voyage from regional to field scales across Europe, J. Hydrol., 37, 100903, https://doi.org/10.1016/j.ejrh.2021.100903, 2021.
Nemes, A., Rawls, W. J., and Pachepsky, Y. A.: Influence of Organic Matter on the Estimation of Saturated Hydraulic Conductivity, Soil Sci. Soc. Am. J., 69, 1330–1337, https://doi.org/10.2136/sssaj2004.0055, 2005.
Prasuhn, V., Humphys, C., and Spiess, E.: Seventy-Two Lysimeters for Measuring Water Flows and Nitrate Leaching under Arable Land, NAS International Workshop on Applying the Lysimeter Systems to Water and Nutrient Dynamics, Wanju, Korea, 1–24, https://link.ira.agroscope.ch/en-US/publication/36079 (last access: 1 November 2023), 2016.
Rawls, W. J., Nemes, A., and Pachepsky, Y.: Effect of soil organic carbon on soil hydraulic properties, in: Developments in Soil Science, edited by: Pachepsky, Y. and Rawls, W. J., Elsevier, 95–114, https://doi.org/10.1016/S0166-2481(04)30006-1, 2004.
Renwick, L. L. R., Deen, W., Silva, L., Gilbert, M. E., Maxwell, T., Bowles, T. M., and Gaudin, A. C. M.: Long-term crop rotation diversification enhances maize drought resistance through soil organic matter, Environn Resn Lettn, 16, 084067, https://doi.org/10.1088/1748-9326/ac1468, 2021.
Rivier, P.-A., Jamniczky, D., Nemes, A., Makó, A., Barna, G., Uzinger, N., Rékási, M., and Farkas, C.: Short-term effects of compost amendments to soil on soil structure, hydraulic properties, and water regime, J. Hydrol. Hydromech., 70, 74–88, https://doi.org/10.2478/johh-2022-0004, 2022.
Smith, P., Martino, D., Cai, Z., Gwary, D., Janzen, H., Kumar, P., McCarl, B., Ogle, S., O'Mara, F., Rice, C., Scholes, B., Sirotenko, O., Howden, M., McAllister, T., Pan, G., Romanenkov, V., Schneider, U., Towprayoon, S., Wattenbach, M., and Smith, J.: Greenhouse gas mitigation in agriculture, Philos. T. R. Soc. B, 363, 789–813, https://doi.org/10.1098/rstb.2007.2184, 2008.
Szabó, B., Weynants, M., and Weber, T. K. D.: Updated European hydraulic pedotransfer functions with communicated uncertainties in the predicted variables (euptfv2), Geosci. Model Dev., 14, 151–175, https://doi.org/10.5194/gmd-14-151-2021, 2021.
Topa, D., Cara, I. G., and Jităreanu, G.: Long term impact of different tillage systems on carbon pools and stocks, soil bulk density, aggregation and nutrients: A field meta-analysis, CATENA, 199, 105102, https://doi.org/10.1016/j.catena.2020.105102, 2021.
Turek, M. E. and Holzkämper, A.: Supporting data to Turek et al. 2023 SOIL (Version 1), Zenodo [data set], https://doi.org/10.5281/zenodo.10068907, 2023.
University of Wageningen: SWAP Soil Water Atmosphere Plant, https://www.swap.alterra.nl/, last access: 7 November 2023.
van Genuchten, M. T.: A Closed-form Equation for Predicting the Hydraulic Conductivity of Unsaturated Soils, Soil Sci. Soc. Am. J., 44, 892–898, https://doi.org/10.2136/sssaj1980.03615995004400050002x, 1980.
Van Looy, K., Bouma, J., Herbst, M., Koestel, J., Minasny, B., Mishra, U., Montzka, C., Nemes, A., Pachepsky, Y. A., Padarian, J., Schaap, M. G., Tóth, B., Verhoef, A., Vanderborght, J., van der Ploeg, M. J., Weihermüller, L., Zacharias, S., Zhang, Y., and Vereecken, H.: Pedotransfer Functions in Earth System Science: Challenges and Perspectives, Rev. Geophys., 55, 1199–1256, https://doi.org/10.1002/2017RG000581, 2017.
Wagner, B., Tarnawski, V. R., and Stöckl, M.: Evaluation of pedotransfer functions predicting hydraulic properties of soils and deeper sediments, J. Plant Nutr. Soil Sc., 167, 236–245, https://doi.org/10.1002/jpln.200321251, 2004.
Wang, T., Wedin, D., and Zlotnik, V. A.: Field evidence of a negative correlation between saturated hydraulic conductivity and soil carbon in a sandy soil, Water Resour. Res., 45, W07503, https://doi.org/10.1029/2008WR006865, 2009.
Weber, T. K. D., Weynants, M., and Szabó, B.: R package of updated European hydraulic pedotransfer functions (euptf2), Zenodo [code], https://doi.org/10.5281/zenodo.4281045, 2020.
Zhang, X., Jia, J., Chen, L., Chu, H., He, J.-S., Zhang, Y., and Feng, X.: Aridity and NPP constrain contribution of microbial necromass to soil organic carbon in the Qinghai-Tibet alpine grasslands, Soil Biol. Biochem., 156, 108213, https://doi.org/10.1016/j.soilbio.2021.108213, 2021.