the Creative Commons Attribution 4.0 License.
the Creative Commons Attribution 4.0 License.
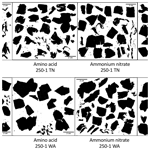
Organic and inorganic nitrogen amendments reduce biodegradation of biodegradable plastic mulch films
Sreejata Bandopadhyay
Marie English
Marife B. Anunciado
Mallari Starrett
Jialin Hu
José E. Liquet y González
Douglas G. Hayes
Sean M. Schaeffer
Biodegradable mulch films (BDMs) are a sustainable and promising alternative to non-biodegradable polyethylene mulches used in crop production systems. Nitrogen amendments in the form of fertilizers are used by growers to enhance soil and plant-available nutrients; however, there is limited research on how these additions impact the biodegradation of BDMs tilled into soils. A 4-month laboratory incubation study using soil microcosms was used to investigate the effects of inorganic (ammonium nitrate) and organic (urea and amino acids) nitrogen application on biodegradation of BDMs. We investigated the response of soil bacterial, fungal, and ammonia-oxidizing microbial abundance along with soil nitrogen pools and enzyme activities. Microcosms were comprised of soils from two diverse climates (Knoxville, TN, USA, and Mount Vernon, WA, USA) and BioAgri, a biodegradable mulch film made of Mater-Bi®, a bioplastic raw material containing starch and poly(butylene adipate-co-terephthalate) (PBAT). Both organic and inorganic nitrogen amendments inhibited mulch biodegradation, soil bacterial abundances, and enzyme activities. The greatest inhibition of mulch biodegradation in TN soils was observed with urea amendment where biodegradation was reduced by about 6 % compared to the no-nitrogen control. In WA soils, all nitrogen amendments suppressed biodegradation by about 1 % compared to the no-nitrogen control. Ammonia monooxygenase amoA gene abundances were increased in TN soils in all treatments but reduced for all treatments in WA soils. However, a significantly higher nitrate concentration and a lower ammonium concentration were seen for all nitrogen treatments compared to no-nitrogen controls in both TN and WA. This study suggests that the addition of nitrogen, particularly inorganic amendments, could slow down mulch biodegradation but that mulch biodegradation does not negatively affect soil nitrification activity.
- Article
(3489 KB) - Full-text XML
-
Supplement
(1558 KB) - BibTeX
- EndNote
Plastic mulch films provide several key benefits to crop production systems. In addition to conserving soil moisture and moderating soil temperatures, mulches suppress weed growth and limit contamination of crops by soil (Hayes et al., 2019; Kasirajan and Ngouajio, 2012; Serrano-Ruiz et al., 2021). The development of a conducive microclimate enhances crop yields (Miles et al., 2012; Moreno and Moreno, 2008). Multiple crops are grown using mulch films such as wheat (Chakraborty et al., 2008; Li et al., 2005); cotton (Chen et al., 2014); and vegetables like okra, squash, and bell peppers (Mahadeen, 2014; Filipović et al., 2016). Most of the mulch films used in agriculture are made of polyethylene (PE), which is poorly biodegradable and requires disposal after the growing season, typically ending up in landfills (Hayes et al., 2012). Fragments of PE mulches may remain in the soil and cause potential harm to soil and aquatic ecosystems (Bandopadhyay et al., 2018). Biodegradable mulch films (BDMs) are a sustainable alternative to PE films because they are made of biodegradable polymers such as starch, polyesters, and lignin. BDMs are meant to be tilled into soils where microbes convert them into carbon dioxide, water, and microbial biomass.
One concern that growers have regarding BDMs is the unpredictable breakdown in the field. Both above-soil and in-soil degradation rates differ among BDMs and climates. There is no standard currently available pertaining to BDM degradation in field conditions; however there are several which certify biodegradability of BDMs. ASTM D6400 (2012), which is an International Organization for Standardization (ISO) equivalent (Dentzman, 2019), tests biodegradation under industrial composting conditions and is the most commonly cited standard in reference to BDMs. The European Committee for Standardization (CEN) released the European Standard EN 17033 in January 2018, which was the first standard to certify plastic mulch films as “biodegradable” in ambient soil (CEN, 2018) and use laboratory tests mimicking field soil conditions. Both ASTM D6400 and EN 17033 address inherent biodegradability and ecotoxicity. The EN 17033 standard requires 90 % mineralization of mulch C to CO2 in 2 years using ASTM D5988 test, and the ASTM D6400 standard requires 90 % mineralization to CO2 within 6 months by the ASTM D5338 test. Of course, even BDMs meeting the standards in laboratory tests may have variable in-field degradation performance: climate, crop, weathering, and soil management can all influence biodegradation rates, and complete biodegradation is not always achieved quickly (Anunciado et al., 2021; Hayes, 2018).
Because of variable degradation rates of BDMs in the field, understanding controls on BDM biodegradation is critical to supporting the use of BDMs within the farming community. The possible accumulation of non-degraded BDM fragments in soil over time could impact soil infiltration rates, further affecting water uptake by plants and microbes. Even though BDMs are a small carbon input compared to extant soil carbon and crop residues (Ding et al., 2021), BDM fragments in soil may affect ecosystem functions over the long term. Short-term studies of 4 years demonstrate that BDMs have comparable effects to PE films and do not alter soil quality and soil biological functions significantly in that time period (Sintim et al., 2019, 2021). However, effective ways to facilitate mulch biodegradation in the soil are warranted in a way that would not adversely affect soil health.
Combining plastic mulching with fertilizer application has been known to increase crop yield almost 2-fold (Li et al., 2003; Lamont, 2005). Fertilizers have long been used in the field by growers to improve soil functioning and crop yields by providing essential nutrients to plants. Common inorganic nitrogen fertilizers include ammonium nitrate, urea, ammonium sulfate, and diammonium phosphate, and organic fertilizers commonly include amendments such as manure and straw. Even though the addition of organic fertilizers can increase the bacteria-to-fungi ratio in soil (Marschner et al., 2003) and rapidly increase CO2 evolution (Ajwa and Tabatabai, 1994), their nutrients are slowly released and hence often not immediately available to plants. Inorganic fertilizers act rapidly but can accumulate toxic concentration of salts in soils and vegetables if over-applied (Santamaria, 2006). When fertilizers are used in soils, these nutrient pulses could lower soil C : N ratios, causing enhanced or reduced decomposition of organic material in the soil (Marschner et al., 2003).
It has long been recognized that soil mineral elements, particularly nitrogen, play a critical role in constraining the rate of organic matter decomposition. Increased organic matter decomposition due to nitrogen deposition is well documented in the literature (Hobbie, 2000; Hobbie and Vitousek, 2000; Hunt et al., 1988; Knorr et al., 2005; Boxman et al., 1995; Vitousek et al., 1997). Nitrogen additions are also known to accelerate the decomposition of light soil carbon fractions (Neff et al., 2002). Even though most studies report a positive effect of nitrogen addition on the decomposition of plant litter and organic matter, a few studies also point towards a negative effect of nitrogen on microbial activity and organic matter decomposition (Fog, 1988; Fang et al., 2007; Janssens et al., 2010). Thus, mixed results are encountered when looking at the effects of nitrogen on stability and turnover of soil carbon pools.
Some environmental factors that affect biodegradation rates of BDMs are considered to be (1) environmental conditions, such as temperature, moisture, pH, and oxygen availability, and (2) physicochemical properties of the polymeric materials (Kijchavengkul et al., 2008). However, information regarding the impacts of soil-specific factors such as nitrogen content on BDM degradation processes is sparse. It has been speculated that nitrogen-limited microorganisms may not produce esterases that degrade BDMs because the hydrolysis of polyesters does not increase nitrogen availability for the organism (Sander, 2019). One study showed better correlation of the degree of degradation of biodegradable plastics with the total nitrogen content of soil than with the total carbon content (Hoshino et al., 2001). Another study by Thompson et al. (2019) evaluated the effects of urea and other biodegradation amendments on polylactic acid (PLA) mulch degradation in microcosms, but the impacts of such amendments on mulch decomposition and microbial respirations were reported to be inconsistent across the different PLA mulch types throughout the study.
The overall objective of our study was to better understand how nitrogen amendments influence biodegradation of BDMs in soil. Our specific objectives were to determine if (1) the addition of nitrogen amendments in the form of organic and inorganic treatments stimulates the biodegradation of biodegradable mulch films and (2) the addition/degradation of BDMs has an impact on soil nitrification activity. Since the polymers used in BDMs are composed of C, O, and H, microbes need to acquire nitrogen from the surrounding soil for growth. Therefore, microbes may experience nitrogen limitation in the soil or on the surfaces of rapidly depolymerizing C-rich polymers. Thus, we hypothesized that nitrogen amendments would enhance mulch biodegradation and nitrification by alleviating N limitation compared to treatments with no added nitrogen. We also hypothesized that biodegradation of mulch material would have a minimal effect on soil nitrification processes based on our prior observations that BDMs had a minimal impact on soil microbial communities and biogeochemical cycling in field soils (Sintim et al., 2019, 2021; Bandopadhyay et al., 2020). For this study, we intentionally chose soils from an experimental agricultural field where BDMs had been used for the prior 3 years, and the microbial communities had a legacy of mulch exposure. We did not alter the soil chemistry prior to the experiment. Details for the field experiment design and management can be found in Sintim et al. (2021).
2.1 Site description and soil sampling
Soils for this experiment were collected from two locations: East Tennessee Research and Education Center (ETREC), University of Tennessee, Knoxville, Tennessee, USA, and the Northwestern Washington Research and Extension Center (NWREC), Washington State University, Mount Vernon, Washington, USA. The soil at Knoxville is a sandy loam (59.9 % sand, 23.5 % silt, and 16.6 % clay), classified as fine-loamy, mixed, subactive, thermic Typic Hapludult or Haplic Alisols. The soil at Mount Vernon is a silt loam (14.2 % sand, 69.8 % silt, and 16 % clay), classified as fine-silty, mesic Fluvaquent Endoaquepts or Haplic Fluvisols. Soil and weather characteristics are included in Table 1.
Table 1Soil and weather characteristics in Knoxville, TN, and Mount Vernon, WA (Sintim et al., 2019). CEC: cation exchange capacity.
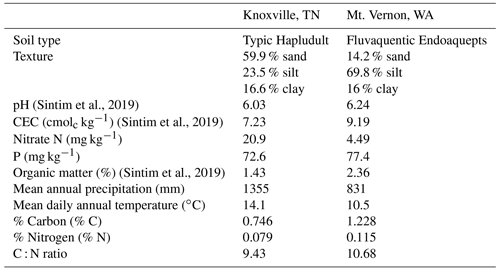
Soil samples were obtained from control (unmulched) plots from a vegetable cropping field experiment comparing different mulch treatments (experimental details can be found in (Sintim et al., 2021). A total of 30 soil cores were collected using soil augers (0 to 10 cm depth) from the four replicated unmulched plots in TN and WA in July 2018. Soils were collected into pre-sterilized buckets, and any roots or debris were picked out. Soils from one plot were composited by homogenizing them by hand and then putting them into Ziplock® bags. These were stored in a cooler with ice packs for transport to the laboratory. Field-moist soil was then passed through a 2 mm sieve to remove any remaining coarse rock and plant material. Soils from the four no-mulch plots at each location were then mixed together to form a homogenized soil sample. These soils were kept at room temperature for 7 d at 25 ∘C.
2.2 Biodegradable mulch used for incubation study
BioAgri, a starch–PBAT (poly(butylene adipate-co-terephthalate)) blend BDM made by BioBag® USA, was used in this study. Agriculturally weathered BioAgri plastic mulch samples (hereafter “plastic”) were collected at the end of the 2015 growing season at the TN field site after 3 months of exposure to the environment. Film thickness and surface area density of the weathered mulch were 0.078 ± 0.0188 mm and 25.17 ± 4.578 g m−2 respectively (Hayes et al., 2017). Mulch pieces were cleaned using a dry, clean brush to dust off soil by gentle dabbing prior to use. Plastic pieces were cut into 2 cm by 2 cm pieces and 250 mg plastic weighed in aluminum tins to be added to respective microcosms. About 25 to 30 mulch pieces were added per jar in the study. We know from previous studies that agriculturally weathered mulch films degrade differently than virgin mulch (Hayes et al., 2017), and the choice of agriculturally weathered mulch in this study was intentional. However, we acknowledge the possibility of agriculturally weathered mulch being a heterogeneous test material with altered chemistry and adherent soil particles and microbes. We intended this experiment to more closely represent field processes, even though it introduced more heterogeneity and variability to the system.
2.3 Laboratory incubation experimental design
After incubation at room temperature, 100 g of field moist soil was weighed into 473 mL glass canning jars and adjusted to 50 % water-holding capacity (WHC) by adding deionized water. After adjusting WHC, jars containing soils were pre-incubated in the dark at 25 ∘C for an additional 5 d. The experiments consisted of two treatment factors, plastic addition (0 and 250 mg) and nitrogen amendments in the form of urea (CH4N2O, from Fisher Chemical, purity 99.7 %), ammonium nitrate (NH4NO3, from Fisher Chemical, purity 99.9 %), amino acids (as a complete supplemental medium (CSM) powder from Sunshine Science Products), and a no-nitrogen control. A quantity of 790 mg of the CSM powder contained the following amounts of each amino acids: adenine hemisulfate (10 mg), L-arginine (50 mg), L-aspartic acid (80 mg), L-histidine hydrochloride monohydrate (20 mg), L-isoleucine (50 mg), L-leucine (100 mg), L-lysine hydrochloride (50 mg), L-methionine (20 mg), L-phenylalanine (50 mg), L-threonine (100 mg), L-tryptophan (50 mg), L-tyrosine (50 mg), L-valine (140 mg), and uracil (20 mg). At the start of the incubation experiment, jars containing soil were taken out of the incubator, and nitrogen amendments were added at a rate of 50 mg N per kg soil on an N-equivalent basis, similar to field application rates in TN. Soils were taken out of pre-incubated jars and put in a large weigh boat, where the soil was mixed with the respective amendments using a spatula. Quantities of 1 mL of 0.2 M solution of urea and ammonium nitrate and 1 mL of 31.6 g L−1 CSM, each dissolved in deionized (DI) water, were added to the respective soils, and 1 mL of DI water was added to soils designated as no-nitrogen control. All treatments were done in triplicate. After the mixing of amendments, soil and agriculturally weathered plastic pieces (pre-cut into 2 cm by 2 cm squares) were arranged alternately in the jars in a way such that two layers of plastic remained buried within the soils, ensuring that all the plastic was covered by soil. Given that this was a small volume packed with soil and mulch, we hypothesize that there was quite good contact between soil and mulch. Incubation was carried out in the dark at 30 ∘C for 16 weeks after amendment application. A total of 48 experimental units were set up in a full factorial design: 2 soil types × 2 plastic treatments × 4 nitrogen treatments × 3 replicates × 1 time point at 16 weeks. Time zero (t=0) samples were collected on the day of the start of the experiment before nitrogen application.
2.4 Soil analyses before incubation
For all t = 0 measurements, 10 additional jars were set up with soils adjusted to 50 % WHC (five from TN, five from WA). These were also pre-incubated along with all the experimental units. On the first day of the experiment (t = 0, when N amendments were added), 1 mL of DI water was added to one of the jars to account for the change in moisture after adding nitrogen amendment, and soils from that jar were stored in the −80 ∘C freezer for DNA extractions and enzyme assays to be done later. Gravimetric moisture content was determined for these soils, which was used for dry-soil-weight calculations. K2SO4 extractions were completed on soil samples at t = 0 with added nitrogen amendments to account for any differences in carbon and nitrogen pools, which might result due to the addition of N. For this, 5 g of soils from three of the five replicate jars was weighed out, and urea, ammonium nitrate, and amino acids (CSM) were added at the same concentration to 100 g soil in microcosms. A quantity of 20 mL of 0.5 M K2SO4 was added to these jars, and extractions were completed. All samples were shaken in an incubator shaker at 160 rpm for 4 h, after which these were filtered through a vacuum manifold using 1 µm glass microfiber filters and stored at −20 ∘C. These extracts were used for nitrate and ammonium assays.
Soils (40 mg) were collected before and after the incubation, dried at 60 ∘C and ground, and subsequently analyzed for the total percent of carbon (“% C”) and total percent of nitrogen (“% N”) using a Costech elemental analyzer (Costech, EA ECS4010) at the Stable Isotope Laboratory in the Department of Earth and Planetary Sciences at the University of Tennessee. L-glutamic acid and acetanilide were used as calibration standards for % C and % N.
2.5 Gas chromatography and microcosm maintenance
Headspace gas samples were collected in 12 mL gas vials starting from the day of start of experiment. Gas sampling was done every 2 to 3 d for the first 2 weeks after which it was reduced to once per week, sampling until day 119 (∼ 16 weeks). For the first day of gas sampling, after addition of N amendments, jars were vented, and then the first gas samples were taken using a 30 mL syringe. A quantity of 15 mL of gas was used to over-pressurize 12 mL vials (pre-evacuated to 200 mTorr). Needles were cleaned out between samples to avoid cross-contamination. For subsequent gas sampling days, jars were vented for a minute after gas sample collection to avoid excessive gas build-up in jars. Preliminary results from a trial run indicated the need to dilute gas samples. Thus, before reading gas samples, all vials were diluted in highly pure N2 gas about 13-fold. Diluted gas samples were then read in a gas chromatograph (Shimadzu GC-2014, Shimadzu, Japan) following the methods described in Hu et al. (2021b). The majority of the dilutions were prepared by one person to avoid human variation introduced with different techniques of dilution.
Microcosms were opened every sampling day to vent, and during that time jars were weighed and kept at a constant moisture. Jars weights taken at t = 0 were used as a reference, and the weights were kept constant by adding DI water as needed. Any unburied plastic pieces were re-buried. Gas samples were also taken after venting of jars was complete to make sure that the venting method was yielding similar gas concentrations in all jars. GC data confirmed that the venting was consistently done throughout the experiment.
2.6 Soil analyses after incubation
2.6.1 Soil physicochemical analyses
At the end of the experiment, soil samples from each microcosm were sieved through a 2 mm sieve to separate plastics and soil samples. Soils from each jar was weighed out for pH, electrical conductivity (EC), gravimetric soil moisture, K2SO4 extractions, nitrate assays, ammonium assays, DNA extractions, and enzyme assays.
Gravimetric soil moisture was measured on the soils collected at t = 16 weeks by weighing ∼ 3 g of soil from the jars and then drying them in an oven (Heratherm OGS100, Thermo Scientific) for 48 h at 105 ∘C. The dry weight was noted after 48 h, and then the gravimetric soil moisture was calculated using Eq. (1).
where masswet soil is the mass of wet soil (g), massdry soil is the mass of dry soil (g), and pH and electrical conductivity (EC) were measured from soils collected at t = 16 weeks by preparing soil slurries (5 g soil: 10 mL deionized water). pH was measured using a benchtop pH meter (SevenCompact™ Benchtop pH meter, Mettler Toledo), whereas EC was measured using a handheld multiparameter meter (Orion Star A329, Thermo Scientific), with each probe being calibrated prior to use. EC was measured immediately after vortexing the slurry, and pH was measured after approximately 15 min, allowing the fine particles to settle.
Soils were processed to determine % C and % N values similarly as the pre-incubation samples. Untreated soil samples from final and initial time points (without added nitrogen amendments and mulch) were used to calculate changes in % C, % N, and C : N ratios. The relative change in C : N ratio was calculated as per Eq. (2) (Schmidt and Gleixner, 2005).
2.6.2 Quantitative PCR
Extraction of DNA from soil samples was completed using the MoBio™ PowerLyzer™ Power Soil DNA isolation kit (now Qiagen™ PowerSoil kit) per manufacturer's instructions. A quantity of 0.25 g soil was used for the extractions, and the DNA obtained after the final elution step (60 µL) was stored at −20 ∘C until further analyses. The extracted DNA was used to quantify bacterial, fungal, and nitrifier gene abundances in the soil collected both at t = 0 and t = 16 weeks.
As a proxy for bacterial and fungal abundances, 16S rRNA (bacteria) and ITS (fungi) gene copy numbers were quantified from soil DNA samples using a Femto™ Bacterial DNA Quantification Kit (Zymo Research) and a Femto™ Fungal DNA Quantification Kit (Zymo Research) following the manufacturer's protocol. DNA extracts were diluted 1:10 prior to quantification. All samples were analyzed in triplicate. No-template negative controls were included in each run. Bacterial and fungal DNA standards were provided in the kit and used to calculate to copy numbers. qPCR reactions were performed in a CFX Connect Real-Time PCR Detection System (BioRad). qPCR efficiencies averaged around 79 % for bacterial and fungal assays. Standard curves had R squared values ranging from 0.98 to 0.99.
Quantitative PCR of ammonia-oxidizing bacteria (AOB) amoA (ammonia monooxygenase subunit) genes was performed using the SYBR Green Master Mix and a CFX Connect Real-Time PCR Detection System (Bio-Rad laboratories, Hercules, CA, USA) following the procedure described in Hu et al. (2021b). Briefly, primers amoA-1F and amoA-2R (Rotthauwe et al., 1997) were used, and standard curves were constructed with plasmids containing cloned amoA products from environmental DNA. qPCR efficiencies averaged around 86 %. Standard curves had R squared values ranging from 0.98 to 0.99.
2.6.3 Soil enzyme assays
Fluorescence microplate enzyme assays were conducted to determine soil enzyme activity rates before and after the incubation study using the protocol described in Bandopadhyay et al. (2020). Briefly, assays were completed using fluorescently labeled substrates targeted for three common carbon and nitrogen cycling enzymes that are known to degrade sugar, chitin, and cellulose (Bell et al., 2013). The synthetic fluorescent indicators used were 4-methylumbelliferone (MUB) and 7-amino-4-methylcoumarin (MUC). The enzyme activity was measured as the fluorescent dye was released from the substrate by an enzyme-catalyzed reaction, with higher fluorescence indicating more substrate degradation compared to lower fluorescence. We prepared separate calibration curves of fluorescent standards for each soil extract, such that each soil sample had its own calibration curve. The targeted enzymes and their respective fluorescently labeled substrates are given in Table S1.
2.6.4 Nitrate and ammonium assays
Nitrate and ammonium concentrations in soil samples before and after 16 weeks' incubation were determined using colorimetric assays. K2SO4 extractions were completed after 16 weeks on all 48 soil samples collected from the experimental units. A quantity of 10 g of soil was mixed with 40 mL of 0.5M K2SO4, and blanks were also extracted using only K2SO4. All samples were shaken in an incubator shaker at 160 rpm for 4 h, after which these were filtered through a vacuum manifold using 1 µm glass microfiber filters and stored at −20 ∘C. Nitrate and ammonium were measured on these extracts. Ammonium in soil extracts was measured according to Rhine et al. (1998). Briefly, 70 µL of sample extract and ammonium standards (ammonium sulfate [(NH4)2SO2]) was pipetted into respective wells of a 96-well plate; all samples were analyzed in triplicate. Reagents (50 µL citrate reagent, 50 µL 2-phenylphenol-nitroprusside, 25 µL buffered hypochlorite reagent, 50 µL Milli-Q water) were allowed to react with the sample by shaking for 30 s on a table shaker. After incubation at room temperature for 2 h, absorbance (660 nm) was measured on a Biotek® Synergy H1 plate reader. Some samples were run again using a 1 : 1 dilution so that they could be read using the standard curve; samples were diluted in 0.5M K2SO4. Nitrate in soil extracts was measured according to Doane and Horwáth (2003). A quantity of 30 µL of sample and nitrate standards (potassium nitrate, KNO3) was added to respective wells in 96-well plates; all samples were analyzed in triplicate. The reagent, vanadium (III) chloride solution (300 µL), was then added to each well and the plate incubated for 5 h at room temperature. Absorbance (540 nm) was measured on the plate reader. All samples were diluted 1:10 in 0.5 M K2SO4.
2.7 Plastic analysis after incubation
2.7.1 Plastic sampling strategy and imaging
Soils were sieved post incubation to separate remaining plastics. Plastics collected from one of the replicates were laid out on a piece of white paper (12 cm × 12 cm) and imaged using a 12-megapixel camera, which was put on a level to stabilize. Visual percent of biodegradation, estimated through the loss of surface area for the mulch film pieces, was determined using Image-J software (Schneider et al., 2012) using the following steps: the scale was set to a ruler included in the image, the image was converted to an 8 bit image, and the threshold was adjusted to black and white before analyzing the particles (Cowan et al., 2013). One concern was that some particles were larger than the starting value of 4 cm2, indicating that some plastics were fused together. Therefore, the visual estimates may underestimate the actual amount of plastic remaining. Plastic mulches recovered from the first replicate microcosms for each treatment were also imaged on a Zeiss EVO MA 15 scanning electron microscope.
2.7.2 Percent biodegradation calculation using CO2 evolution data
Based on the ASTM D5988-18 standard (ASTM, 2018), the extent of aerobic biodegradation of BioAgri was determined by measuring evolved CO2 as a function of time that the plastic was exposed to the soil. The starting amount of plastic carbon in the soil microcosms was determined to be 1309 µg plastic carbon per g dry soil for TN jars and 1317 µg plastic carbon per g dry soil for WA jars. Using the CO2 evolution data (µg C released per g dry soil), the amount of plastic carbon released over 16 weeks was calculated. This along with the starting plastic carbon value was used in Eq. (3) to determine the percent of biodegradation for each treatment after 16 weeks.
where % bio is the percent of biodegradation, is the CO2 C evolved in mulch treatments (µg C per g dry soil), is the CO2 C evolved in treatments without mulch (µg C per g dry soil), and MC,mulch is the total amount of C added in the form of mulch C (µg C per g dry soil).
2.7.3 Thermogravimetric analysis (TGA)
TGA was carried out for BioAgri plastics retrieved from microcosms after 16-week incubation and compared to the starting material (agriculturally weathered plastic stored at room temperature) following the protocol by Hayes et al. (2017). Briefly, the test was conducted using a Discovery TGA (TA Instruments, New Castle, DE USA) at a heating rate of 10 ∘C min−1 from room temperature (25 ∘C) to 600 ∘C in an unsealed platinum sample pan under a nitrogen atmosphere which underwent dynamic flow.
2.7.4 ATR–FTIR spectrometry and gel permeation chromatography
Attenuated total reflectance–Fourier transform infrared (ATR–FTIR) absorbance spectra (4000–600 cm−1) were collected to determine changes in chemical bonding of mulch polymers (Table S7) that resulted from the nitrogen treatments following the protocol described by Hayes et al. (2017). Briefly, spectra were taken of the plastics, before and after laboratory incubation using an IRAffinity-1 spectrometer (Shimadzu Co., Tokyo, Japan) equipped with a single-reflection ATR system (MIRacle ATR, PIKE Technologies, Madison, WI, USA). The parameters for measurement included a resolution of 2 cm−1 and 16 scans per spectrum. Data were corrected for the background signal attributable to the ATR crystal. Spectral data were normalized by equating the integrated peak area of the entire spectrum to 1.0 (mean normalization). Gel permeation chromatography was performed as described in Hayes et al. (2017).
2.7.5 Statistical analysis
Student's t test was conducted to look at differences in bacterial 16S rNA, fungal ITS, and amoA gene abundances; nitrate and ammonium concentrations; and enzyme activity rates at t = 16 weeks compared to t = 0. Additionally, for statistics reported in figures, this study used a two-way analysis of variance (ANOVA) with plastic and nitrogen amendment as factors to isolate the effects of nitrogen treatment on all response metrics, separating out the location variable prior to two-way ANOVA testing. For mulch component analysis (% starch, % PBAT) through TGA, data were subjected to two-way ANOVA using N amendment and location as factors. Differences between means were performed using Tukey's honestly significant difference (HSD) analysis at significant difference level of α=0.05. Three-way ANOVA values including effects of location, plastic, and nitrogen amendment were also conducted (results reported in the Supplement). Analysis was conducted using R version 3.4.0. When the ANOVA indicated significant effects (p<0.05), a post hoc analysis with Tukey's HSD (honest significance test) was applied to compare the means. The experiment design included three replications of each treatment. Data were checked for normality using the Shapiro–Wilk test (W>0.9). Outliers were identified as those differing substantially from the rest of the data (typically Z score >3) and removed. If normality was not achieved, the data were log-transformed as appropriate. For microbial abundances, nitrate and ammonium concentrations, and enzyme activity rates, the starting value of the soil was subtracted from the measured value after 16 weeks, and this change score was used for analyses.
To understand if location had a significant effect on the different parameters measured, we conducted three-way ANOVA type III tests (Table S2). We found a significant main effect of location and significant interaction effects of location with nitrogen and plastic addition for almost all parameters measured, and hence we focused on two way-ANOVA results within TN and WA sites separately (Table 2). The two-way ANOVAs tested differences between nitrogen amendments and plastic addition levels for the different parameters evaluated: bacterial, fungal, and amoA gene abundances; nitrate and ammonium concentrations; and enzyme activities.
Table 2F values from a two-way ANOVA showing effects of nitrogen and plastic treatment on soil chemical and biological characteristics. Significant differences are in bold. * p<0.05. p<0.01. p<0.001.
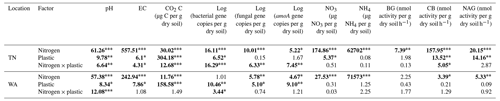
EC: electrical conductivity. BG: β-glucosidase. CB: β-D-cellubiosidase. NAG: N-acetyl β-glucosaminidase.
3.1 Soil physicochemical response
When comparing treatments with and without plastic using two-way ANOVAs, it was observed that ammonium nitrate significantly reduced EC with plastic compared to ammonium nitrate treatment without plastic for TN soils, whereas for WA soils, all treatments had significantly reduced EC with plastic as compared to without plastic. Urea application resulted in significantly greater pH with plastic for both TN and WA soils. The addition of nitrogen, in any form, caused a significant increase in the EC both in the presence and in the absence of plastic in TN and WA soils. Likewise, the addition of nitrogen, in any form, caused a significant drop in pH both in the presence and in the absence of plastic in TN and WA soils, with the exception of urea treatment in WA, where the pH drop is neutralized by plastic. Significant interaction effects were seen between nitrogen and plastic for all pH and EC data in TN and WA soils, except for EC measurements in WA soil where main effects for nitrogen and plastic treatments are reported (Fig. 1, Table 2).
Soil C : N ratios decreased for nitrogen treatments as well as no-nitrogen controls in TN and WA after incubation (Table S3). When analyzing only the treatments which did not receive any added nitrogen or mulch, we observed a reduction in the % C, % N, and C : N values after 16 weeks (comparing final to initial time point; Table S3). The relative change in C : N ratio was calculated for the no-mulch and no-nitrogen controls and revealed a 6 % decrease for TN soil and 9 % decrease for WA soil.
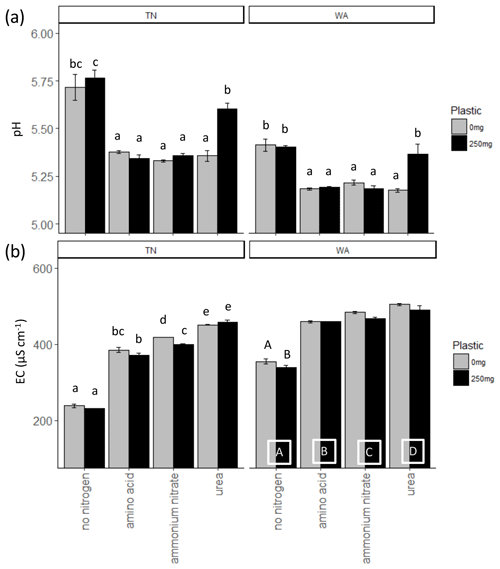
Figure 1(a) pH and (b) electrical conductivity (EC) measurements at t = 16 weeks. Lowercase letters indicate significant interaction effects at α≤0.05. For EC measurements in WA, uppercase letters along the x axis denote a significant main effect of nitrogen amendment at α≤0.05. Uppercase letters on top of the bars in the EC plot for WA indicate a significant main effect of plastic at α≤0.05. TN: Tennessee. WA: Washington.
3.2 Plastic biodegradation in microcosms
Nitrogen addition resulted in similar responses in CO2 evolution (µg C per g dry soil) in both TN and WA soils over the 16-week incubation (Fig. S1). When analyzing the cumulative CO2 C evolved after 16 weeks using two-way ANOVA, significant interaction effects were seen between nitrogen and plastic in TN; hence, interaction effects are reported (Table 2, Fig. 2). In WA, interaction effects were not significant; hence, the main effects of nitrogen amendment and plastic addition are reported. When plastic was added, cumulative CO2-C respiration was significantly greater for the no-nitrogen control compared to amino acid, ammonium nitrate, and urea in TN and ammonium nitrate and urea in WA (TN no-nitrogen: 230.4 µg C per g dry soil, WA no-nitrogen: 155.06 µg C per g dry soil; Fig. 2). The amino acid treatment had the least reduction in CO2-C evolution compared to no-nitrogen control over time in both locations, whereas the inorganic amendments using urea and ammonium nitrate had the greatest reduction in CO2-C evolution over 16 weeks. Plastic treatments showed significantly greater CO2-C evolution compared to treatments without plastic in both TN and WA, indicating that biodegradation was occurring. When only nitrogen amendments were added without plastic, CO2 C evolved was significantly higher for amino acid amendment compared to ammonium nitrate in TN, whereas in WA, CO2 C was significantly greater for no-nitrogen and amino acid treatments compared to ammonium nitrate and urea. The increased plastic degradation observed with no-nitrogen and amino acid treatments in TN and WA was corroborated visually by images of remaining plastic material in microcosms taken after 16 weeks (Fig. 3, Supplement Figs. S2 and S3) and reflected in maximum theoretical percent biodegradation estimates (Table S4) and visual % degradation via surface area calculation in ImageJ (Fig. S4).
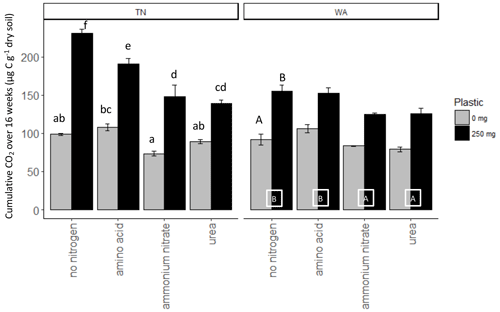
Figure 2Cumulative CO2 C released over 16 weeks (119 d) in soil (TN and WA) microcosms with and without biodegradable plastic mulches (BDMs). AA: amino acids. AN: ammonium nitrate. No N: no nitrogen added. TN: Tennessee. WA: Washington.
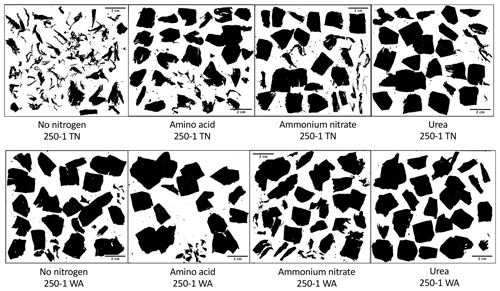
Figure 3Black–white contrast images in ImageJ of plastic pieces after 16 weeks incubation. All images were taken from mulches from the first replicate microcosm for each treatment. TN: Tennessee. WA: Washington.
Thermogravimetric analysis (TGA) results were analyzed using thermograms (Fig. 4a, b) and differential thermograms (the first derivative of the thermograms or DTGs; Fig. 4c, d) of BioAgri after nitrogen amendment to the soil. The heating rate was constant, at 10 ∘C min−1. Peaks for the two main heating stages occur at 310 and 390 ∘C, which represent starch and PBAT, respectively. The relative proportion of mass loss during a heating stage, represented by the area underneath the curve for the DTG peaks, represent the relative mass fractions for the components. The onset, maximum, and final temperatures of the heating stage for starch and PBAT components of BioAgri are provided in the Supplement Table S5. A few nitrogen amendments led to a shift to lower temperatures for the PBAT heating stage, TN-urea, TN-ammonium nitrate, and WA-amino acid, most easily observable by the DTGs (Fig. 4c, d). All nitrogen treatments led to a broadening of the starch heating stage, suggesting starch's microbial utilization and possibly more efficient biomass incorporation. Nitrogen treatment increased the percentage of weight (hereafter “% weight”) remaining at 600 ∘C, specifically, for ammonium nitrate in TN and amino acid in WA (Supplement Table S5). Any residual material remaining at 600 ∘C is likely inorganic components of the mulch and soil particulates adhered to the mulch, and/or the formation of gelled polymer formation due to UV radiation from sunlight, which is unlikely to occur in a soil environment. Therefore, the % weight remaining at 600 ∘C is expected to increase during biodegradation, due to utilization of the polymeric components as carbon sources for microorganisms. After incubation in TN soil, the % weight remaining at 600 ∘C was highest for ammonium nitrate (33 %) and no-nitrogen (33 %), followed by urea (28 %) and amino acid (20 %) treatments. In contrast, for WA the % weight remaining was higher for amino acids (37 %) and no-nitrogen (23 %) amendments. Both ammonium nitrate and urea treatments in WA had comparable % weight remaining, at ∼ 18 %.
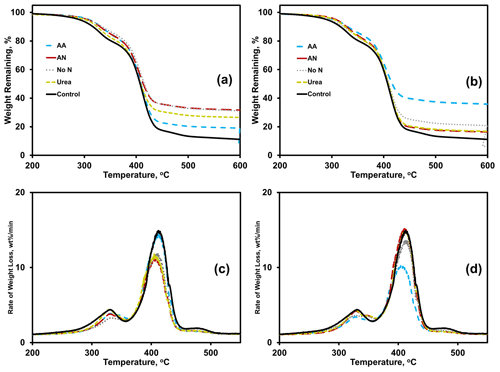
Figure 4Effects of nitrogen amendments on BioAgri plastic as revealed by thermogravimetric analysis (TGA thermograms; a, b) and differential thermograms (DTG; c, d). Plastic were exposed to either TN (a, c) or WA (b, d) soils. AA: amino acid. AN: ammonium nitrate. No N: no nitrogen added. TN: Tennessee. WA: Washington. Control refers to agriculturally weathered BioAgri plastic mulch samples in TN (Hayes et al., 2017).
The fraction of starch and PBAT as components of BioAgri mulch was determined after the 16-week incubation (Fig. S5) using the thermal degradation process. Comparison of mass fractions of PBAT between soils from TN and WA did show trends. Starch significantly decreased in nitrogen amendment treatments (p=0.0344) compared to agriculturally weathered control but was not significantly different between two soil types used (p=0.0542). For both TN and WA soils, amino acid and ammonium nitrate treatments led to more loss of starch components than other treatments.
The gel permeation chromatography analysis of molecular weight data solely represents PBAT since PBAT is the only component of BioAgri that dissolves in chloroform. Loss of weight-averaged molecular weight was observed for all nitrogen treatments, including no-nitrogen, for both TN and WA soils compared to agriculturally weathered control (Supplement Table S6). The decrease of weight-averaged molecular weight, Mw, was not statistically significant (p=0.0591) among the nitrogen treatments and between soils (p=0.4829). Polydispersity index (PDI), however, which reflects the distribution of molecular weight values for the polymeric strands, increased after nitrogen addition in TN soil (p=0.0072), with significant statistical difference among treatments (p=0.0091). The no-nitrogen controls for both soils underwent a PDI increase similar to all nitrogen treatments in TN soils but not for any of the nitrogen-amended soil in WA (Supplement Table S6).
FTIR data show changes in the spectra for amino acid and ammonium nitrate treatments, particularly in TN: the increase of peak intensity of –OH stretching (3700–3000 cm−1) and a shift in the carbonyl (C = O) spectral region to lower wavenumbers (1800–1600 cm−1), especially the formation of a band at 1650 cm−1, which likely represents COOH end groups (Fig. 5). Peak assignments for FTIR analysis are shown in the Supplement Table S7. These changes in FTIR spectra reflect the hydrolysis that occurred on the surface of mulch films when soil was amended with amino acid and ammonium nitrate substrates, likely a result of biodegradation (Fig. 5). In contrast, the FTIR spectrum for urea treatment was nearly identical with the spectrum for the control, suggesting that the extent of hydrolysis was relatively small in the presence of urea.
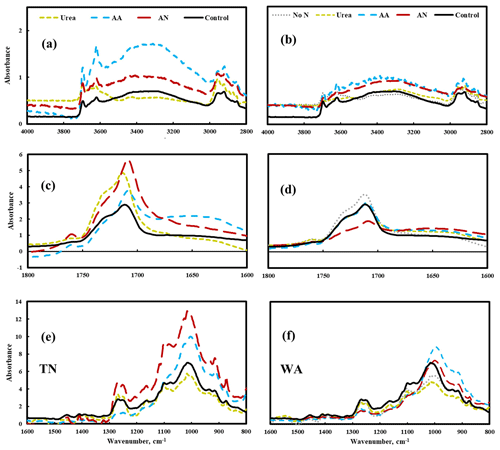
Figure 5Changes in chemical bonds of biodegradable mulch (BioAgri) after biodegradation in nitrogen-amended soil (left panel a, c, e: TN, right panel b, d, f: WA) via FTIR analysis. Mulches retrieved from no-nitrogen (TN) control were too degraded for FTIR analysis. AA: amino acid. AN: ammonium nitrate. No N: no nitrogen added. TN: Tennessee. WA: Washington. Control refers to agriculturally weathered BioAgri plastic mulch samples in TN (Hayes et al., 2017).
3.3 Bacterial and fungal abundances in microcosms
For bacterial abundances, significant interaction effects were observed between nitrogen and plastic factors in TN and WA using two-way ANOVAs; hence, interaction effects are reported (Fig. S6a, Table 2). For fungal abundances, interaction effects were observed with TN but not with WA soils (Fig. S6b, Table 2). When comparing plastic treatments, it was observed that plastic addition tempered the bacterial reduction effect from ammonium nitrate, with treatments without plastic showing significantly reduced bacterial abundance compared to treatments with plastic in TN. Similarly, in WA, the only significant difference was seen with the no-nitrogen control, with plastic addition tempering the bacterial reduction effect. There were no significant differences in fungal abundance between treatments with and without plastic in TN for any of the nitrogen treatments. However, for WA soils, plastic addition caused a significantly greater fungal abundance compared to treatments without plastic for all nitrogen amendments.
Effects of nitrogen amendments on bacterial and fungal abundances were evaluated for treatments without added plastic (Fig. S6). It was seen that without plastic, ammonium nitrate resulted in significantly reduced bacterial abundance in TN compared to other nitrogen treatments. However, no differences were observed between nitrogen treatments without plastic in WA. Ammonium nitrate without plastic also resulted in significantly reduced fungal abundances compared to amino acid and urea without plastic in TN, whereas the no-nitrogen control had significantly reduced fungal abundance compared to amino acid treatment. In WA, amino acid treatment without plastic showed significantly greater fungal abundances compared to other treatments without plastic. For treatments with plastic, nitrogen amendments did not cause a significant change in bacterial abundances for TN and WA. Nitrogen treatments with plastic also did not cause a significant change in fungal abundances for TN; however, in WA, amino acid treatment resulted in a significantly greater fungal abundance compared to other treatments. Overall, for both TN and WA, a reduction in bacterial abundance was observed after 16 weeks. However, there was a significant increase in fungal abundance for both locations over 16 weeks.
3.4 Nitrification processes and extracellular enzymatic functions in soil microcosms
For ammonia monooxygenase (amoA) gene abundances, two-way ANOVAs revealed a significant interaction effect between nitrogen and plastic in TN, while the main effects are reported for WA (Table 2). When comparing between plastic treatments, the no-nitrogen control with plastic was shown to cause a significantly higher amoA gene abundance compared to no-nitrogen control without plastic in TN (Fig. 6a). In WA, treatments without plastic showed a significantly greater reduction in amoA gene abundances when compared to plastic treatments for all nitrogen amendments. Urea without plastic treatments in TN showed significantly greater amoA abundance compared to ammonium nitrate and no-nitrogen without plastic, whereas, in WA, amino acid and urea without plastic showed significantly reduced amoA abundances compared to no-nitrogen without plastic. No significant difference in amoA gene abundance was observed for nitrogen treatments with plastic in TN. In WA, amino acid and urea with plastic showed significantly reduced amoA abundances compared to no-nitrogen with plastic. Overall, trends differed between TN and WA, with increased amoA abundance in TN but a decreased abundance in WA after 16 weeks.
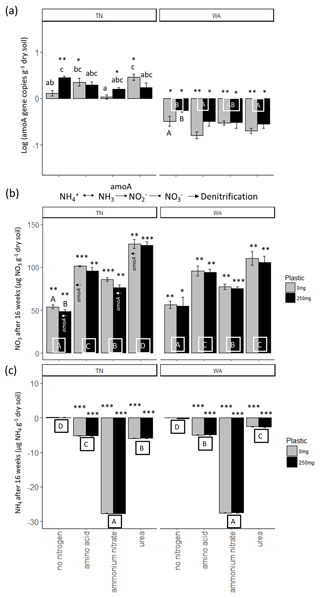
Figure 6(a) Changes in ammonia monooxygenase (amoA) gene abundances over 16 weeks. Gene abundances were log-transformed, and then abundances in initial soils were subtracted from final (16 week) samples. Each bar represents a mean of 3 replicate microcosms and error bars are standard error. Lowercase letters indicate interaction effects at α≤0.05 for amoA abundance in TN. Uppercase letters along x axis in WA plot indicate significant main effect of nitrogen treatment at α≤0.05. Uppercase letters below bars in WA indicate a significant main effect of plastic at α≤0.05. Asterisks indicate significant increase or decrease in amoA gene abundance from t = 0 as per a t test. * p≤0.05, p≤0.01, p≤0.001. (b) Changes in nitrate (NO3) and (c) ammonium (NH4) concentration in soils over 16 weeks. Each bar represents a mean of 3 replicate microcosms and error bars are standard error. For nitrate concentration, uppercase letters along the x axis indicate a significant main effect of nitrogen treatment at α≤0.05 and uppercase letters above bars indicate a significant main effect of plastic at α≤0.05. Treatments that had increased quantities of amoA genes (per panel a) are indicated with amoA↑ for reference. For ammonium concentration, uppercase letters below bars indicate a significant main effect of nitrogen treatment. Asterisks indicate significant increase or decrease in nitrate and ammonium concentrations from t = 0 based on a t test. * p≤0.05, p≤0.01, p≤0.001. TN: Tennessee, WA: Washington.
Nitrification was increased post nitrogen amendment addition as demonstrated via changes in nitrate and ammonium concentrations after 16 weeks, as revealed by two-way ANOVAs (Fig. 6b, c). All added nitrogen treatments resulted in significantly increased nitrate and decreased ammonium concentrations compared to no-nitrogen controls in both TN and WA soils after 16 weeks. Plastic treatments had significantly lower nitrate concentration compared to treatments without plastic in TN. The results were supported by amoA gene abundances in TN, which were significantly increased from t = 0 for urea and amino acid treatments with no plastic added and no-nitrogen and ammonium nitrate with added plastic.
Most of the enzyme activities measured decreased after 16 weeks except for no-nitrogen control (with and without plastic) and ammonium nitrate (without plastic) in TN, which showed increased β-D-cellubiosidase (CB) activity (Fig. S7). β-Glucosidase (BG) and CB activities increased for ammonium nitrate treatment (with added plastic) in WA after 16 weeks but were not significant. There was no difference in enzyme activities when comparing between plastic treatments, with a few exceptions. Addition of plastic caused a greater reduction in N-acetyl β-glucosaminidase (NAG) activity for all nitrogen treatments and a reduction in CB activity for ammonium nitrate treatment in TN after 16 weeks (Fig. S7).
When comparing nitrogen treatments without plastic addition, it was seen that ammonium nitrate caused a significantly greater reduction in BG activity compared to no-nitrogen and amino acid in TN. For CB activity in TN, amino acid and urea had a significant reduction in activity compared to no-nitrogen and ammonium nitrate which caused increases in CB activity after 16 weeks. In WA, urea caused a significantly greater reduction in CB activity compared to ammonium nitrate. Nitrogen amendments without plastic caused a significantly greater reduction in NAG activity in TN compared to no-nitrogen control, whereas in WA, urea and no-nitrogen caused a greater reduction in NAG activity compared to ammonium nitrate (Fig. S7).
When comparing nitrogen treatments with added plastic, it was observed that ammonium nitrate had significantly reduced BG activity compared to no-nitrogen and amino acid in TN after 16 weeks. Amino acid and urea caused significantly greater reductions in CB activity compared to ammonium nitrate in TN, whereas only the no-nitrogen control had an increase in CB activity after 16 weeks. In WA, no-nitrogen, urea, and amino acid amendments caused reduced CB activity after 16 weeks, with only ammonium nitrate having a significant increase in CB activity compared to the reduction observed for urea treatment. All nitrogen amendments with plastic had reduced NAG activity compared to no-nitrogen in TN, whereas in WA, no-nitrogen and urea caused significant reductions compared to an increase in NAG activity for ammonium nitrate (Fig. S7).
4.1 Nitrogen application reduced plastic biodegradation in both TN and WA soils
The plastics added in the microcosms underwent biodegradation, as evidenced by the increased CO2 C released in microcosms with added plastic, with about 10 % and 5 % biodegradation in no-nitrogen controls in TN and WA, respectively. The greater mulch mineralization in TN may be explained by a lower C : N ratio compared to WA soils, suggesting increased availability of bacterial cellular resources to utilize carbon substrates. The occurrence of biodegradation is also supported by the decrease in molecular weight for PBAT (GPC (gel permeation chromatography) analysis); the utilization of starch, which is the anticipated carbon source for the early stage of biodegradation (TGA analysis); and evidence of hydrolysis on the surface of the mulches (FTIR analysis), with the observed changes being greater for TN than for WA. There was some discrepancy between the theoretical biodegradation percentages and the visual estimates reported by imaging of plastics after incubation and calculation of their surface area in ImageJ. In certain treatments, it was observed that the plastics were fused together to form larger particle sizes, whereas in no-nitrogen controls the plastics were too degraded to be able to physically recover them from the jars. Hence, it is likely that the visual estimates were underestimating the actual area of plastic remaining post incubation. While there are constraints to the visual estimation of mulch degradation, we still argue that it gives good relative estimates across treatments and has been shown as effective supporting data in other studies of mulch degradation (e.g., Sintim et al., 2020; Yamamoto-Tamura et al., 2015). Methodically, it is challenging to recover mulches which have degraded to fine particles using a sieving method, and better approaches need to be developed for recovery of mulch particles and accurate assessments.
Contrary to our hypothesis that nitrogen addition would stimulate plastic biodegradation, we instead saw a reduction of plastic biodegradation with added nitrogen amendments. This was accompanied by a minor reduction of background soil organic matter decomposition in response to nitrogen amendment only. Addition of nitrogen resulted in reduction of plastic biodegradation by 6 percentage points over 16 weeks for urea treatment in TN; in WA, all the nitrogen treatments showed around 1 percentage point reduction in biodegradation. However, given there were minimal differences in mulch degradation between nitrogen and no-nitrogen treatments in WA soils, it is important to note that reduction by 1 percentage point in mulch biodegradation could be due to variations between incubations. The CO2-C results were validated visually, with decreased macroscopic plastic degradation apparent in nitrogen-amendment microcosms. Even though TN and WA soils had different starting soil properties, the trends for reduction in CO2 C were similar between treatments in both locations, indicating that both soils were probably not nitrogen limited to begin with. Studies with forest soils have reported comparable findings where nitrogen deposition was shown to prevent organic matter decomposition when nitrogen was not limiting (Janssens et al., 2010). However, in such a situation it would be expected that the responses to nitrogen addition would be comparable to no-nitrogen controls. The fact that there was a reduction in microbial activity in response to nitrogen amendments in the present study suggests a possible negative effect. Excessive nitrogen deposition in an ecosystem can reach a point of nitrogen saturation which could have severe environmental impacts on soil chemistry and water quality (Aber, 1992). There could also be a decrease in productivity in terrestrial ecosystems through the loss of base cations and decreased phosphorus availability (Jin-yan and Jing, 2003; Janssens et al., 2010). A study by Thompson et al. (2019) measured biodegradation of different PLA mulches in response to biodegradation amendments such as urea and reported that PLA mulch with composites such as soy particles experienced greater degradation in the presence of urea when compared to other PLA mulches without composites. It could be that the addition of labile carbon to the PLA enhanced biodegradation in response to nitrogen amendments and that we did not see this affect with our PBAT mulch because it lacked a more labile additive. The decreased mulch biodegradation in the nitrogen addition treatments could also be partly explained by acidification of the soils due to nitrification of added nitrogen compounds. Highest mulch biodegradation occurred in the no-nitrogen controls, which also had the highest pH. Low pH has been shown to limit carbon use efficiency by increasing fungal abundance (Silva-Sánchez et al., 2019). In agreement with this, we observed an increased fungal abundance for some nitrogen treatments with added mulch compared to no-nitrogen.
The form of nitrogen added dictated the magnitude of the degradation repression especially in TN soils. For this experiment, though we added different forms of nitrogen, we added it at a rate similar to field application rates of nitrogen in TN to mimic agronomic reality. Addition of amino acids in the form of a complete supplemental media resulted in greater plastic biodegradation compared to urea. This may be because in addition to N, amino acids supply labile carbon and sulfur, which may have stimulated microbial activity. In the absence of plastic, microbial activity was highest for the amino acid amendment in both TN and WA. Complex organic nitrogen sources have been reported to have a positive effect on organic matter decomposition in other studies, due to carbon source or a vitamin effect rather than a nitrogen induced effect (Fog, 1988). It should also be noted that our study was carried out over a period of 4 months. A detailed review of various studies reported by Fog (1988) indicates that the repressive effects of nitrogen addition are more apparent in longer-term (months) studies because (1) nitrogen addition can affect the competition between potent and less potent decomposers; (2) “ammonia metabolite repression” occurs, where nitrogen blocks production of certain enzymes, especially in fungi; and (3) toxic or inhibitory compounds are produced, formed by the reaction of amino compounds with polyphenols and other decomposition products. Although it is generally known that addition of nitrogen does not always promote decomposition of organic matter, it was not until the research conducted by Keyser et al. (1978) that it was clearly demonstrated how nitrogen can have a negative effect on the production of certain enzymes involved in organic matter decomposition in fungi, particularly in Basidiomycetes.
In our study we use the term biodegradation to refer to the theoretical biodegradation of mulch, which was computed by the subtraction of no-mulch controls and assumes that CO2 produced in the plastic treatments derives from the plastics. We acknowledge that there could be priming effects of added mulch inputs to soil carbon decomposition that complicate this assumption. Priming, defined as the change in microbial decomposition of soil organic carbon in response to fresh carbon inputs (Bastida et al., 2019), can be considered extra decomposition of native soil organic matter in a soil receiving an organic amendment. Lower nitrate values in the plastic treatments in TN could be an indicator of microbial assimilation of nitrogen required to support the uptake of plastic carbon by microbes (Ardisson et al., 2014). In the present study, it is difficult without labeled substrates (e.g. 13C-labeled mulch) to assess how much of the carbon is directly evolved from the plastic vs. enhanced CO2 release from soil organic matter due to the addition of mulch. This limitation is acknowledged, and future experiments with labeled mulches could precisely track these pools of carbon. Such labeled studies will also help to track whether starch or PBAT was preferentially utilized for microbial respiration, thus enabling a direct link between mulch component used and percent biodegradation. There are studies that have used similar approaches, such as the study by Zumstein et al. (2018). However, mulch carbon inputs to soil in the field are typically much lower than other forms of organic matter that support priming processes, and therefore we anticipate the contribution of priming to be minimal in our system.
There was good correlation between the percent degradation values from the CO2 data and the percent weight remaining from TGA for all the treatments in TN and WA. There is evidence that nitrogen amendment led to depolymerization of PBAT, as shown by the greater decrease in Mw in TN for nitrogen treatments compared to unamended soil (GPC analysis, Supplement Table S6) and the greater extent of hydrolysis on the surface, particularly for amino acid and ammonium nitrate treatments in TN (FTIR analysis, Fig. 5). Interestingly, specific treatments affected microbial communities' utilization of carbon source: for instance, ammonium nitrate and amino acid amendments and the no-nitrogen control underwent greater utilization of starch by microbes as demonstrated via TGA analysis (Fig. S5).
One important aspect to note about organic nitrogen amendments and mulch biodegradation is the addition of labile carbon along with nitrogen. Carbon was present in two treatments, the amino acid treatment (which was added as complete supplemental media) and urea. For the amino acid treatment, we observed relatively less reduction of mulch biodegradation compared to the other nitrogen treatments. In urea, we observed more reduction in biodegradation in comparison to the other nitrogen treatments. It is possible, in the case of urea treatment, that the carbon addition from urea was relieving the need to access mulch carbon; however, we note that the ratio of mulch carbon to amendment carbon was roughly 7.7 for the amino acid treatment and 57 for urea. Given that the carbon input from urea was particularly low, we anticipate this contribution to be minimal.
We note some caveats with respect to interpretation of our study. The focus of this experiment was on acute or early effects of a nitrogen fertilization event on mulch degradation and therefore would not necessarily predict degradation over longer timescales (e.g., years). Correlating the early reduction in biodegradation that we observe over 16 weeks to the performance of a standard 2-year biodegradability test would be a valuable follow-up experiment. Furthermore, we did not measure microbial biomass, but we know that changing nutrient conditions can change microbial carbon use efficiency (CUE), and typically a decrease in C : N leads to an increase in CUE. It may be that a portion of the CO2 decrease was due to changes in biomass. However, because our CO2 data correspond well to visual degradation patterns, it suggests that CO2 was a reliable indicator of degradation of mulch in our experiment and that biomass changes would have only minimally contributed to the observed change in CO2.
4.2 Responses of soil bacterial, fungal, and ammonia-oxidizing bacteria to nitrogen and plastic amendments
An overall decrease in bacterial gene abundance was observed for all treatments after 16 weeks. However, a significantly enriched fungal gene abundance was observed over 16 weeks for all nitrogen treatments with and without plastic in TN and WA. Increased fungal abundances in all nitrogen treatments with added plastic, coupled with reduced plastic biodegradation observed in only nitrogen-added treatments, point towards a possibility of ammonia metabolite repression in response to the added nitrogen. Such a repression has been demonstrated with Basidiomycetes, where enzymes degrading organic matter, such as lignin, are produced when the fungus has switched from primary to secondary metabolism. This switch is induced only when the supplies of available nitrogen, such as ammonium, have been exhausted (Fog, 1988). Several studies point towards the important role of fungi in the degradation of biodegradable mulch with evidence of enrichment of Aspergillus and Penicillium (Koitabashi et al., 2012) and several genera of Ascomycota (Muroi et al., 2016) under biodegradable mulch application. Cellulose- and polyester-degrading basidiomycetes are also common (de Souza, 2013; Watanabe et al., 2014). Even though nitrification processes reduced the ammonium concentration in microcosms after 16 weeks, the residual ammonium could have caused a repression of enzymatic function, which can explain the reduced mulch degradation observed.
The presence of nitrifying bacteria in the soil microcosms was confirmed by increased amoA gene abundances in TN soils after 16 weeks and changes in soil ammonium and nitrate pools in both TN and WA soil microcosms. Bacterial amoA gene abundances varied between treatments in TN soils. However, nitrate concentrations increased, and ammonium decreased for all nitrogen treatments compared to no-nitrogen for both TN and WA soils. These observations point towards active nitrification processes taking place in the soil microcosms with added nitrogen. Furthermore, the reduced pH we observed with nitrogen-amended soils could be explained by the formation of nitric acid from urea and ammonium salts during nitrification (Yan et al., 1996). Previous studies have reported that urea-N additions can lead to significant increases in ammonia-oxidizing bacteria (AOB) abundance, activity, and diversity (Chen et al., 2013; Hu et al., 2021a, b).
There was minimal effect of plastic addition on the nitrifying potential of the soil microbial community. This suggests that the plastic material used in the study does not inhibit nitrifying populations to an appreciable extent. This observation is supported by previous studies where use of Mater-Bi® demonstrated no ecotoxicological effect on the soil (Ardisson et al., 2014), and BioAgri films did not significantly affect bulk soil quality or microbial communities (Bandopadhyay et al., 2020; Sintim et al., 2019, 2021). The presence of plastic only influenced nitrate concentrations in TN soils where plastic addition reduced nitrate concentrations for all treatments. In both TN and WA soils, ammonium concentrations did not differ between plastic and no-plastic treatments. Previous studies have shown that plastic film mulches along with nitrogen fertilizers increased soil nitrate N (Gao et al., 2009) and decreased or did not affect N2O emissions (Liu et al., 2014; Berger et al., 2013) under the films while on the surface. In this study, we saw that BDMs buried in soil had comparable effects to no-plastic treatments on nitrification processes.
4.3 Responses of soil hydrolytic enzyme activities to nitrogen and plastic amendments
A general trend of reduced soil enzyme activities was observed for all treatments after 16 weeks. Acidification of soils in the microcosms may have led to reduced enzyme activities as a result of effects on microbial physiology and base cation limitation (Shi et al., 2018). Reduced CB activity was observed for most nitrogen amendments after 16 weeks, whereas a significantly increased activity was seen for the no-nitrogen control in TN. Reduced NAG activity has been reported in the literature post urea addition to soils at a rate of 392 kg N ha−1 yr−1 over a 7-year period (Zhang et al., 2016). A few other studies have reported no change in BG activity after nitrogen application in temperate grasslands (Wang et al., 2014), whereas another study found that nitrogen addition over just a 2-year period led to significant decreases in urease activity (Zhou et al., 2012). Decrease in peptidase activity has been documented by several other studies, where some report depressed activity after 10 years of ammonium nitrate addition (Stursova et al., 2006) and others report dramatically reduced activities after nitrogen addition (Saiya-Cork et al., 2002). Thus, these trends seem to point towards a general decline in enzyme activity after nitrogen addition in both short and long-term experiments. A negative relationship between NAG activities and soil NH-N content is also reported by Zhang et al. (2016), indicating that ammonia could have an inhibitory effect on N-hydrolases. Given these effects of nitrogen addition on soil enzyme activity, we point out that the reduction in plastic biodegradation in nitrogen-amended treatments could be a direct or indirect (via soil modification) effect of nitrogen amendment. While we do not test this explicitly in the present study, we do note that the reduction of mulch degradation could be due to reduced enzyme activities in nitrogen-treated soil. The only effect of plastic that was observed was for NAG activity in TN, where addition of plastic seemed to cause a greater reduction in NAG activity after 16 weeks compared to treatments without plastic. With field studies, it has been observed that addition of BDM in soil has very little effect on the activities of common carbon and nitrogen cycling enzymes when compared to treatments without mulch, at least over a 2-year period (Sintim et al., 2019; Bandopadhyay et al., 2020; Sintim et al., 2021).
In summary, we found that plastic biodegradation was slightly but significantly reduced under inorganic nitrogen amendments, with the highest plastic biodegradation seen for the no-nitrogen control, followed by amino acid treatments. Identical CO2-C respiration trends were observed for both soils from TN and WA although the percent of biodegradation of plastic varied between locations. Evidence of increased soil nitrification was observed under nitrogen treatments compared to no-nitrogen. Reduced soil enzyme activity rates after 16 weeks suggested a potential inhibitory effect of nitrogen activity on hydrolases. The effect of plastic addition had minimal effects on nitrification processes and enzyme activities after 16 weeks. The results indicate that addition of nitrogen fertilizers in cropping systems with tilled-in BDM fragments may inhibit biodegradation of mulch material without compromising nitrification processes.
All data used in the preparation of this paper are available in the figshare repository (https://doi.org/10.6084/m9.figshare.21566019, Bandopadhyay et al., 2023).
The supplement related to this article is available online at: https://doi.org/10.5194/soil-9-499-2023-supplement.
Conceived and designed the experiments: SB, JMD, DGH, and SMS. Performed the experiments: SB, ME, MBA, MS, JH, and JELyG. Analyzed and interpreted the data: SB, ME, MBA, JMD, and DGH. Wrote the paper: SB and JMD with inputs from other authors.
The contact author has declared that none of the authors has any competing interests.
Publisher's note: Copernicus Publications remains neutral with regard to jurisdictional claims in published maps and institutional affiliations.
We thank Sindhu Jagadamma and Shikha Singh for the training and help provided to operate the Shimadzu gas chromatograph, Xiangru Xu and Tori Beard for help with soil processing, and Arnold Saxton for statistical advice.
This research has been supported by the United States Department of Agriculture (grant no. 2014-51181-22382).
This paper was edited by Peter Fiener and reviewed by two anonymous referees.
Aber, J. D.: Nitrogen cycling and nitrogen saturation in temperate forest ecosystems, Trend. Ecol. Evol., 7, 220–224, https://doi.org/10.1016/0169-5347(92)90048-G, 1992.
Ajwa, H. A. and Tabatabai, M. A.: Decomposition of different organic materials in soils, Biol. Fert. Soil., 18, 175–182, https://doi.org/10.1007/bf00647664, 1994.
Anunciado, M. B., Hayes, D. G., Astner, A. F., Wadsworth, L. C., Cowan-Banker, C. D., Gonzalez, J. E. L. y., and DeBruyn, J. M.: Effect of environmental weathering on biodegradation of biodegradable plastic mulch films under ambient soil and composting conditions, J. Polym. Environ., 29, 2916–2931, https://doi.org/10.1007/s10924-021-02088-4, 2021.
Ardisson, G. B., Tosin, M., Barbale, M., and Degli-Innocenti, F.: Biodegradation of plastics in soil and effects on nitrification activity. A laboratory approach, Front. Microbiol., 5, 710, https://doi.org/10.3389/fmicb.2014.00710, 2014.
ASTM: “Standard Test Method for Determining Aerobic Biodegradation of Plastic Materials in Soil”, ASTM D5988-18, in: Annual Book of ASTM Standards, Vol. 08.03., 6 pp., https://doi.org/10.1520/D5988-18, 2018.
Bandopadhyay, S., Sintim, H. Y., and DeBruyn, J. M.: Effects of biodegradable plastic film mulching on soil microbial communities in two agroecosystems, PeerJ, 8, e9015, https://doi.org/10.7717/peerj.9015, 2020.
Bandopadhyay, S., Martin-Closas, L., Pelacho, A. M., and DeBruyn, J. M.: Biodegradable plastic mulch films: Impacts on soil microbial communities and ecosystem functions, Front. Microbiol., 9, 819, https://doi.org/10.3389/fmicb.2018.00819, 2018.
Bandopadhyay, S., English, M., Anunciado, M. B., Starrett, M., Hu, J., Gonzalez, J. E. L. y., Hayes, D. G., Schaeffer, S. M., and DeBruyn, J. M.: Dataset for article “Organic and inorganic nitrogen amendments reduce biodegradation of biodegradable plastic mulch films”, figshare [data set], https://doi.org/10.6084/m9.figshare.21566019.v2, 2023.
Bastida, F., García, C., Fierer, N., Eldridge, D. J., Bowker, M. A., Abades, S., Alfaro, F. D., Asefaw Berhe, A., Cutler, N. A., Gallardo, A., García-Velázquez, L., Hart, S. C., Hayes, P. E., Hernández, T., Hseu, Z.-Y., Jehmlich, N., Kirchmair, M., Lambers, H., Neuhauser, S., Peña-Ramírez, V. M., Pérez, C. A., Reed, S. C., Santos, F., Siebe, C., Sullivan, B. W., Trivedi, P., Vera, A., Williams, M. A., Luis Moreno, J., and Delgado-Baquerizo, M.: Global ecological predictors of the soil priming effect, Nat. Commun., 10, 3481, https://doi.org/10.1038/s41467-019-11472-7, 2019.
Bell, C. W., Fricks, B. E., Rocca, J. D., Steinweg, J. M., McMahon, S. K., and Wallenstein, M. D.: High-throughput fluorometric measurement of potential soil extracellular enzyme activities, J. Vis. Exp., 81, e50961, https://doi.org/10.3791/50961, 2013.
Berger, S., Kim, Y., Kettering, J., and Gebauer, G.: Plastic mulching in agriculture – friend or foe of N2O emissions?, Agr. Ecosyst. Environ., 167, 43–51, 2013.
Boxman, A. W., van Dam, D., van Dijk, H. F., Hogervorst, R., and Koopmans, C. J.: Ecosystem responses to reduced nitrogen and sulphur inputs into two coniferous forest stands in the Netherlands, Forest Ecol. Manag., 71, 7–29, 1995.
CEN: EN 17033:2018. Plastics – Biodegradable mulch films for use in agriculture and horticulture – Requirements and test methods, European Standard, European Committee for Standardization, Brussels, Belgium, European Committee for Standardization (CEN), 2018.
Chakraborty, D., Nagarajan, S., Aggarwal, P., Gupta, V., Tomar, R., Garg, R., Sahoo, R., Sarkar, A., Chopra, U. K., and Sarma, K. S.: Effect of mulching on soil and plant water status, and the growth and yield of wheat (Triticum aestivum L.) in a semi-arid environment, Agr. Water Manag., 95, 1323–1334, 2008.
Chen, L.-J., Feng, Q., Li, F.-R., and Li, C.-S.: A bidirectional model for simulating soil water flow and salt transport under mulched drip irrigation with saline water, Agr. Water Manag., 146, 24–33, https://doi.org/10.1016/j.agwat.2014.07.021, 2014.
Chen, Y., Xu, Z., Hu, H., Hu, Y., Hao, Z., Jiang, Y., and Chen, B.: Responses of ammonia-oxidizing bacteria and archaea to nitrogen fertilization and precipitation increment in a typical temperate steppe in Inner Mongolia, Appl. Soil Ecol., 68, 36–45, 2013.
Cowan, J. S., Inglis, D. A., and Miles, C. A.: Deterioration of Three Potentially Biodegradable Plastic Mulches Before and After Soil Incorporation in a Broccoli Field Production System in Northwestern Washington, HortTechnology Hortte, 23, 849–858, https://doi.org/10.21273/HORTTECH.23.6.849, 2013.
de Souza, W. R.: Microbial degradation of lignocellulosic biomass, in: Sustainable degradation of lignocellulosic biomass-techniques, applications and commercialization, InTech, in: Sustainable degradation of lignocellulosic biomass-techniques, applications and commercialization, 207–247, ISBN: 978-953-51-1119-1, https://doi.org/10.5772/54325, 2013.
Dentzman, K. and Hayes, D. G.: The Role of Standards for Use of Biodegradable Plastic Mulches: Truths and Myths Project Fact Sheet EXT-2019-01, https://biodegradablemulch.tennessee.edu/ (last access: 5 September 2023) 2019.
Ding, F., Flury, M., Schaeffer, S. M., Xu, Y., and Wang, J.: Does long-term use of biodegradable plastic mulch affect soil carbon stock?, Resour. Conserv. Recycl., 175, 105895, https://doi.org/10.1016/j.resconrec.2021.105895, 2021.
Doane, T. A. and Horwáth, W. R.: Spectrophotometric Determination of Nitrate with a Single Reagent, Anal. Lett., 36, 2713–2722, https://doi.org/10.1081/AL-120024647, 2003.
Fang, H., Mo, J., Peng, S., Li, Z., and Wang, H.: Cumulative effects of nitrogen additions on litter decomposition in three tropical forests in southern China, Plant Soil, 297, 233–242, 2007.
Filipović, V., Romić, D., Romić, M., Borošić, J., Filipović, L., Mallmann, F. J. K., and Robinson, D. A.: Plastic mulch and nitrogen fertigation in growing vegetables modify soil temperature, water and nitrate dynamics: Experimental results and a modeling study, Agr. Water Manag., 176, 100–110, 2016.
Fog, K.: The effect of added nitrogen on the rate of decomposition of organic matter, Biol. Rev., 63, 433–462, https://doi.org/10.1111/j.1469-185X.1988.tb00725.x, 1988.
Gao, Y., Li, Y., Zhang, J., Liu, W., Dang, Z., Cao, W., and Qiang, Q.: Effects of mulch, N fertilizer, and plant density on wheat yield, wheat nitrogen uptake, and residual soil nitrate in a dryland area of China, Nutr. Cycl. Agroecosyst., 85, 109–121, https://doi.org/10.1007/s10705-009-9252-0, 2009.
Hayes, D. G., Dharmalingam, S., Wadsworth, L. C., Leonas, K. K., Miles, C., and Inglis, D.:Biodegradable agricultural mulches derived from biopolymers, Degradable Polymers and Materials: Principles and Practice, 2nd Edn., Vol. 1114, 201–223, https://doi.org/10.1021/bk-2012-1114.ch013, 2012.
Hayes, D. G., Wadsworth, L. C., Sintim, H. Y., Flury, M., English, M., Schaeffer, S., and Saxton, A. M.: Effect of diverse weathering conditions on the physicochemical properties of biodegradable plastic mulches, Polymer Testing, 62, 454–467, https://doi.org/10.1016/j.polymertesting.2017.07.027, 2017.
Hayes, D. G., Anunciado, M. B., DeBruyn, J. M., Bandopadhyay, S., Schaeffer, S., English, M., Ghimire, S., Miles, C., Flury, M., and Sintim, H. Y.: Biodegradable Plastic Mulch Films for Sustainable Specialty Crop Production, in: Polymers for Agri-Food Applications, edited by: Gutiérrez, T. J., Springer International Publishing, Cham, 183–213, https://doi.org/10.1007/978-3-030-19416-1_11, 2019.
Hayes, D. G. and Flury, M.: Summary and assessment of EN 17033:2018, a new standard for biodegradable plastic mulch filmsProject Fact Sheet EXT-2018-01, https://biodegradablemulch.tennessee.edu/ (last access: 5 September 2023), 2018.
Hobbie, S. E.: Interactions between litter lignin and soil nitrogen availability during leaf litter decomposition in a Hawaiian montane forest, Ecosystems, 3, 484–494, 2000.
Hobbie, S. E. and Vitousek, P. M.: Nutrient limitation of decomposition in Hawaiian forests, Ecology, 81, 1867–1877, 2000.
Hoshino, A., Sawada, H., Yokota, M., Tsuji, M., Fukuda, K., and Kimura, M.: Influence of weather conditions and soil properties on degradation of biodegradable plastics in soil, Soil Sci. Plant Nutr., 47, 35–43, 2001.
Hu, J., Jin, V. L., Konkel, J. Y. M., Schaeffer, S. M., Schneider, L. G., and DeBruyn, J. M.: Soil health management enhances microbial nitrogen cycling capacity and activity, mSphere, 6, e01237, https://doi.org/10.1128/mSphere.01237-20, 2021a.
Hu, J., Richwine, J. D., Keyser, P. D., Li, L., Yao, F., Jagadamma, S., and DeBruyn, J. M.: Ammonia-oxidizing bacterial communities are affected by nitrogen fertilization and grass species in native C4 grassland soils, PeerJ, 9, e12592, https://doi.org/10.7717/peerj.12592, 2021b.
Hunt, H., Ingham, E., Coleman, D., Elliott, E., and Reid, C.: Nitrogen limitation of production and decomposition in prairie, mountain meadow, and pine forest, Ecology, 69, 1009–1016, 1988.
Janssens, I. A., Dieleman, W., Luyssaert, S., Subke, J. A., Reichstein, M., Ceulemans, R., Ciais, P., Dolman, A. J., Grace, J., Matteucci, G., Papale, D., Piao, S. L., Schulze, E. D., Tang, J., and Law, B. E.: Reduction of forest soil respiration in response to nitrogen deposition, Na. Geosci., 3, 315–322, https://doi.org/10.1038/ngeo844, 2010.
Jin-yan, Y. and Jing, F.: Review of study on mineralization, saturation and cycle of nitrogen in forest ecosystems, J. Forest. Res., 14, 239–243, 2003.
Kasirajan, S. and Ngouajio, M.: Polyethylene and biodegradable mulches for agricultural applications: a review, Agron. Sustain. Dev., 32, 501–529, https://doi.org/10.1007/s13593-011-0068-3, 2012.
Keyser, P., Kirk, T. K., and Zeikus, J. G.: Ligninolytic enzyme system of Phanaerochaete chrysosporium: synthesized in the absence of lignin in response to nitrogen starvation, J. Bacteriol., 135, 790–797, https://doi.org/10.1128/jb.135.3.790-797.1978, 1978.
Kijchavengkul, T., Auras, R., Rubino, M., Ngouajio, M., and Fernandez, R. T.: Assessment of aliphatic–aromatic copolyester biodegradable mulch films, Part I: Field study, Chemosphere, 71, 942–953, https://doi.org/10.1016/j.chemosphere.2007.10.074, 2008.
Knorr, M., Frey, S., and Curtis, P.: Nitrogen additions and litter decomposition: a meta-analysis, Ecology, 86, 3252–3257, 2005.
Koitabashi, M., Noguchi, M. T., Sameshima-Yamashita, Y., Hiradate, S., Suzuki, K., Yoshida, S., Watanabe, T., Shinozaki, Y., Tsushima, S., and Kitamoto, H. K.: Degradation of biodegradable plastic mulch films in soil environment by phylloplane fungi isolated from gramineous plants, AMB Express, 2, 40, https://doi.org/10.1186/2191-0855-2-40, 2012.
Lamont, W. J.: Plastics: Modifying the microclimate for the production of vegetable crops, HortTechnology, 15, 477–481, 2005.
Li, F.-M., Wang, J., and Xu, J.-Z.: Plastic film mulch effect on spring wheat in a semiarid region, J. Sustain. Agr., 25, 5–17, 2005.
Li, F., Xu, J., and Sun, G.: Restoration of degraded ecosystems and development of water-harvesting ecological agriculture in the semi-arid Loess Plateau of China, Acta Ecol. Sin., 23, 1901–1909, 2003.
Liu, J., Zhu, L., Luo, S., Bu, L., Chen, X., Yue, S., and Li, S.: Response of nitrous oxide emission to soil mulching and nitrogen fertilization in semi-arid farmland, Agr. Ecosyst. Environ., 188, 20–28, 2014.
Mahadeen, A. Y.: Effect of polyethylene black plastic mulch on growth and yield of two summer vegetable crops under rain-fed conditions under semi-arid region conditions, Am. J. Agr. Biol. Sci., 9, 202–207, 2014.
Marschner, P., Kandeler, E., and Marschner, B.: Structure and function of the soil microbial community in a long-term fertilizer experiment, Soil Biol. Biochem., 35, 453–461, https://doi.org/10.1016/S0038-0717(02)00297-3, 2003.
Miles, C., Wallace, R., Wszelaki, A., Martin, J., Cowan, J., Walters, T., and Inglis, D.: Deterioration of potentially biodegradable alternatives to black plastic mulch in three tomato production regions, HortScience, 47, 1270–1277, 2012.
Moreno, M. and Moreno, A.: Effect of different biodegradable and polyethylene mulches on soil properties and production in a tomato crop, Sci. Horticul., 116, 256–263, 2008.
Muroi, F., Tachibana, Y., Kobayashi, Y., Sakurai, T., and Kasuya, K.-i.: Influences of poly (butylene adipate-co-terephthalate) on soil microbiota and plant growth, Polym. Degrad. Stabil., 129, 338–346, 2016.
Neff, J. C., Townsend, A. R., Gleixner, G., Lehman, S. J., Turnbull, J., and Bowman, W. D.: Variable effects of nitrogen additions on the stability and turnover of soil carbon, Nature, 419, 915–917, https://doi.org/10.1038/nature01136, 2002.
Rhine, E., Mulvaney, R., Pratt, E., and Sims, G.: Improving the Berthelot reaction for determining ammonium in soil extracts and water, Soil Sci. Soc. Am. J., 62, 473–480, 1998.
Rotthauwe, J.-H., Witzel, K.-P., and Liesack, W.: The ammonia monooxygenase structural gene amoA as a functional marker: molecular fine-scale analysis of natural ammonia-oxidizing populations, Appl. Environ. Microbiol., 63, 4704–4712, 1997.
Saiya-Cork, K., Sinsabaugh, R., and Zak, D.: The effects of long term nitrogen deposition on extracellular enzyme activity in an Acer saccharum forest soil, Soil Biol. Biochem., 34, 1309–1315, 2002.
Sander, M.: Biodegradation of polymeric mulch films in agricultural soils: Concepts, knowledge gaps, and future research directions, Environ. Sci. Technol., 53, 2304–2315, https://doi.org/10.1021/acs.est.8b05208, 2019.
Santamaria, P.: Nitrate in vegetables: toxicity, content, intake and EC regulation, J. Sci. Food Agr., 86, 10–17, https://doi.org/10.1002/jsfa.2351, 2006.
Schmidt, M. and Gleixner, G.: Carbon and nitrogen isotope composition of bulk soils, particle-size fractions and organic material after treatment with hydrofluoric acid, Europ. J. Soil Sci., 56, 407–416, 2005.
Schneider, C. A., Rasband, W. S., and Eliceiri, K. W.: NIH Image to ImageJ: 25 years of image analysis, Nat. Method., 9, 671–675, https://doi.org/10.1038/nmeth.2089, 2012.
Serrano-Ruiz, H., Martin-Closas, L., and Pelacho, A. M.: Biodegradable plastic mulches: Impact on the agricultural biotic environment, Sci. Total Environ., 750, 141228, https://doi.org/10.1016/j.scitotenv.2020.141228, 2021.
Shi, B., Zhang, J., Wang, C., Ma, J., and Sun, W.: Responses of hydrolytic enzyme activities in saline-alkaline soil to mixed inorganic and organic nitrogen addition, Sci. Rep., 8, 4543, https://doi.org/10.1038/s41598-018-22813-9, 2018.
Silva-Sánchez, A., Soares, M., and Rousk, J.: Testing the dependence of microbial growth and carbon use efficiency on nitrogen availability, pH, and organic matter quality, Soil Biol. Biochem., 134, 25–35, https://doi.org/10.1016/j.soilbio.2019.03.008, 2019.
Sintim, H. Y., Bandopadhyay, S., English, M. E., Bary, A., Liquet y González, J. E., DeBruyn, J. M., Schaeffer, S. M., Miles, C. A., and Flury, M.: Four years of continuous use of soil-biodegradable plastic mulch: impact on soil and groundwater quality, Geoderma, 381, 114665, https://doi.org/10.1016/j.geoderma.2020.114665, 2021.
Sintim, H. Y., Bandopadhyay, S., English, M. E., Bary, A. I., DeBruyn, J. M., Schaeffer, S. M., Miles, C. A., Reganold, J. P., and Flury, M.: Impacts of biodegradable plastic mulches on soil health, Agr. Ecosyst. Environ., 273, 36–49, https://doi.org/10.1016/j.agee.2018.12.002, 2019.
Sintim, H. Y., Bary, A. I., Hayes, D. G., Wadsworth, L. C., Anunciado, M. B., English, M. E., Bandopadhyay, S., Schaeffer, S. M., DeBruyn, J. M., Miles, C. A., Reganold, J. P., and Flury, M.: In situ degradation of biodegradable plastic mulch films in compost and agricultural soils, Sci. Total Environ., 727, 138668, https://doi.org/10.1016/j.scitotenv.2020.138668, 2020.
Stursova, M., Crenshaw, C. L., and Sinsabaugh, R. L.: Microbial responses to long-term N deposition in a semiarid grassland, Microb. Ecol., 51, 90–98, 2006.
Thompson, A. A., Samuelson, M. B., Kadoma, I., Soto-Cantu, E., Drijber, R., and Wortman, S. E.: Degradation Rate of Bio-based Agricultural Mulch is Influenced by Mulch Composition and Biostimulant Application, J. Polym. Environ., 27, 498–509, https://doi.org/10.1007/s10924-019-01371-9, 2019.
Vitousek, P. M., Aber, J. D., Howarth, R. W., Likens, G. E., Matson, P. A., Schindler, D. W., Schlesinger, W. H., and Tilman, D. G.: Human alteration of the global nitrogen cycle: sources and consequences, Ecol. Appl., 7, 737–750, 1997.
Wang, R., Filley, T. R., Xu, Z., Wang, X., Li, M.-H., Zhang, Y., Luo, W., and Jiang, Y.: Coupled response of soil carbon and nitrogen pools and enzyme activities to nitrogen and water addition in a semi-arid grassland of Inner Mongolia, Plant Soil, 381, 323–336, 2014.
Watanabe, T., Shinozaki, Y., Yoshida, S., Koitabashi, M., Sameshima-Yamashita, Y., Fujii, T., Fukuoka, T., and Kitamoto, H. K.: Xylose induces the phyllosphere yeast Pseudozyma antarctica to produce a cutinase-like enzyme which efficiently degrades biodegradable plastics, J. Biosci. Bioeng., 117, 325–329, https://doi.org/10.1016/j.jbiosc.2013.09.002, 2014.
Yamamoto-Tamura, K., Hiradate, S., Watanabe, T., Koitabashi, M., Sameshima-Yamashita, Y., Yarimizu, T., and Kitamoto, H.: Contribution of soil esterase to biodegradation of aliphatic polyester agricultural mulch film in cultivated soils, AMB Express, 5, 10, https://doi.org/10.1186/s13568-014-0088-x, 2015.
Yan, F., Schubert, S., and Mengel, K.: Soil pH increase due to biological decarboxylation of organic anions, Soil Biol. Biochem., 28, 617–624, https://doi.org/10.1016/0038-0717(95)00180-8, 1996.
Zhang, X., Tang, Y., Shi, Y., He, N., Wen, X., Yu, Q., Zheng, C., Sun, X., and Qiu, W.: Responses of soil hydrolytic enzymes, ammonia-oxidizing bacteria and archaea to nitrogen applications in a temperate grassland in Inner Mongolia, Sci. Rep., 6, 32791, https://doi.org/10.1038/srep32791, 2016.
Zhou, X., Zhang, Y., and Downing, A.: Non-linear response of microbial activity across a gradient of nitrogen addition to a soil from the Gurbantunggut Desert, northwestern China, Soil Biol. Biochem., 47, 67–77, 2012.
Zumstein, M. T., Schintlmeister, A., Nelson, T. F., Baumgartner, R., Woebken, D., Wagner, M., Kohler, H.-P. E., McNeill, K., and Sander, M.: Biodegradation of synthetic polymers in soils: Tracking carbon into CO2 and microbial biomass, Sci. Adv., 4, eaas9024, https://doi.org/10.1126/sciadv.aas9024, 2018.