the Creative Commons Attribution 4.0 License.
the Creative Commons Attribution 4.0 License.
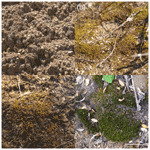
Biocrust-linked changes in soil aggregate stability along a climatic gradient in the Chilean Coastal Range
Nicolás Riveras-Muñoz
Steffen Seitz
Kristina Witzgall
Victoria Rodríguez
Peter Kühn
Carsten W. Mueller
Rómulo Oses
Oscar Seguel
Dirk Wagner
Thomas Scholten
Biological soil crusts (biocrusts) composed of cyanobacteria, bacteria, algae, fungi, lichens, and bryophytes stabilize the soil surface. This effect has mainly been studied in arid climates, where biocrusts constitute the main biological agent to stabilize and connect soil aggregates. Besides, biocrusts are an integral part of the soil surface under Mediterranean and humid climate conditions, mainly covering open spaces in forests and on denuded lands. They often develop after vegetation disturbances, when their ability to compete with vascular plants increases, acting as pioneer communities and affecting the stability of soil aggregates. To better understand how biocrusts mediate changes in soil aggregate stability under different climate conditions, we analyzed soil aggregate samples collected under biocrust communities from four national parks in Chile along a large climatic gradient ranging from (north to south) arid (Pan de Azúcar, PA), semi-arid (Santa Gracia, SG), Mediterranean (La Campana, LC) to humid (Nahuelbuta, NA). Biocrust communities showed a stabilizing effect on the soil aggregates in dry fractions for the three northern sites and the wet aggregates for the southernmost site. Here, permanent vascular plants and higher contents of organic carbon and nitrogen in the soil control aggregate stability more than biocrusts, which are in intense competition with higher plant communities. Moreover, we found an increase in stability for aggregate size classes < 2.0 and 9.5–30.0 mm. The geometric mean diameter of the soil aggregates showed a clear effect due to the climatic gradient, indicating that the aggregate stability presents a log-normal instead of a normal distribution, with a trend of low change between aggregate size fractions. Based on our results, we assume that biocrusts affect the soil structure in all climates. Their role in aggregate stability is masked under humid conditions by higher vegetation and organic matter contents in the topsoil.
- Article
(3147 KB) - Full-text XML
- BibTeX
- EndNote
In recent years, biological soil crusts (biocrusts) have gained particular interest as stabilizers of soil aggregates. Such biocrusts are highly variable communities of microscopic (cyanobacteria, algae, fungi, and bacteria) and macroscopic (lichens, bryophytes) organisms found on the surface and in the upper centimeters of the soil (Gao et al., 2017). They stabilize the soil surface (Garcia-Pichel et al., 2016), especially in arid climates, where biocrusts are the main biological agents for consolidating and connecting soil aggregates (Belnap and Büdel, 2016). However, biocrusts are also present in more mesic regions, e.g., pine barrens, serpentine soils, temperate steppes (Belnap et al., 2016), but due to their limited ability to compete for light, they are mainly relegated to open spaces or interspaces between vascular plants where sunlight reaches the soil surface (Malam Issa et al., 1999).
Because of their simple structure, biocrusts are present in a wide variety of climatic conditions. Biocrust organisms lack specialized desiccation control structures, such as stomata or impermeable cuticles, so their water content depends on the humidity in the surrounding environment (Thielen et al., 2021). However, low water demand, high drought tolerance (Chen et al., 2020), the ability to grow actively only when water is available, and recovering without physiological damage even after completely drying out for an extended period (Oliver et al., 2005) compensate for the lack of dedicated structures. For this reason, biocrusts form an almost continuous layer in arid regions where water availability limits vascular plant cover (Colesie et al., 2014; Grote et al., 2010). By slightly increasing the water availability, areas covered by plants and biocrusts increase in self-organized patterns where both coexist. However, when water demand is no longer restrained, vascular plants have an advantage in using light due to their canopy development, which leads to a decline of biocrusts (Chen et al., 2018).
The cover and composition of biocrusts strongly depend on water availability (Bowker et al., 2016). Under dry conditions, with high potential evapotranspiration, biocrusts are dominated by cyanobacteria, bacteria, and micro-fungi, with few bryophytes or lichens present. However, the occurrence of lichens is not restricted to more humid locations, but lichens were also found in arid regions like Pan de Azúcar (PA) in northern Chile (Jung et al., 2020a, b). It implies that the external morphology of the biocrusts ranges from smooth to rugose (Chamizo et al., 2016). In terms of soil conditions, the water-holding capacity determines how much water can be stored in the soil. Typical sources of soil water are precipitation in general and, more specifically, at valley bottoms with a close connection to the groundwater table, also groundwater. Furthermore, available water for lichen growth can be provided by fog and dew (Jung et al., 2019). Pore space, pore size, and following water-holding capacity of a soil largely depends on the parent material and its degree of weathering. Thus, soil formation indirectly controls the distribution and composition of biocrusts at ecoregional and local scales (Bowker et al., 2016). For instance, Steven et al. (2013) showed that the composition of biocrust communities differed at vertical scales of a few centimeters in soils with different parent material origins, while Bowker et al. (2016) concluded that heterogeneous distributions in parent materials result in abrupt transitions in biocrust distribution and cover.
Biocrusts can be understood as an organic sedimentary system within the topsoil where the inorganic and organic parts play dynamic roles in determining the architecture, evolution, and properties of the system, including structure and aggregate stability. On a small spatial scale, biocrusts interact with the soil system in nitrogen and carbon cycling (Barger et al., 2016). Globally, Elbert et al. (2012) pointed out that cryptogamic covers take up 3.9 Pg C yr−1. The main processes of nitrogen enrichment are biological fixation and dust capture, while nitrogen losses typically appear via dissolution, volatilization, and erosional loss (Barger et al., 2016). Photosynthesis is the most crucial carbon fixation process (Elbert et al., 2012; Porada et al., 2014), and soil erosion and biological decomposition are the primary loss source of carbon and other nutrients (Li et al., 2008).
Biocrusts affect soil erosion by acting as a physical barrier that shields the soil from direct exposition to water and wind (Seitz et al., 2016), protecting it from the effect of raindrops and thus splash erosion (Seitz et al., 2017; Goebes et al., 2015), and modulating the abrasive effect of wind and surface runoff (Belnap and Büdel, 2016). At the same time, biocrusts control water flow across the landscape and through the soil matrix (Thielen et al., 2021; Eldridge et al., 2020). Eldridge et al. (2000) described a decrease in surface runoff and an increase in water infiltration in the presence of biocrusts under semi-arid conditions, related to a reduction in sediment discharge. The influence of biocrusts on the composition of the soil porosity is variable and depends on its stage of development and composition. In some cases, this structuration generates discontinuities that hinder the flow of water in the soil, while in others, it generates a decrease in the tortuosity that is reflected in rapid infiltration (Fischer et al., 2013). Water infiltration is usually inversely related to surface runoff (Lichner et al., 2012). The successional stage of biocrusts affects water repellency compared to bare soil (Drahorad et al., 2013). It has been observed that with the development of biocrusts, the water repellency increased, and the sorptivity and conductivity decreased (Fischer et al., 2012; Lichner et al., 2012). Therefore, biocrusts affect soil erosion and hydrology through a wide variety of processes (Belnap and Büdel, 2016).
With regards to the stability of the soil surface, biocrusts further have a binding effect on aggregates and can form coherent structures (Belnap and Büdel, 2016). Typically, the organic carbon in the form of exopolysaccharides or structural filaments of the different organisms present within biocrust communities causes soil stabilization (Garcia-Pichel et al., 2016). Other structure-forming processes due to biocrusts, although to a lesser extent, are the compressive drying action on the soil matrix and the pH-dependent dissolution of cementing salts (Bowker et al., 2016). The biocrust-induced soil aggradation results in the formation of a defined layer, increasing the soil resistance and resilience to wind and water (Rosentreter et al., 2016).
Biocrusts stabilize individual aggregate units through different mechanisms depending on their species composition (Garcia-Pichel et al., 2016). For instance, bacteria, cyanobacteria, and also green algae play a crucial role in forming and stabilizing aggregates by extracellular polymeric substances that glue soil particles together (Six et al., 2004; Lewin, 1956). Vegetal debris serves as aggregation cores where the soil microorganisms use it as an energy source, but rapid decomposition is limited by the interaction with the inorganic matrix (Oades and Waters, 1991). On the other hand, fungi are important in forming soil aggregates due to their hyphal structure, which physically enmeshes micro-aggregates and soil particles (Totsche et al., 2018). In summary, processes of soil aggregate stabilization are dynamic and occur at different temporal and spatial scales, where an aggregate of soil particles is built up of structural units of various sizes held together by various binding agents.
In this study, we investigate how and to what extent biocrusts stabilize the soil surface under different climatic conditions. Therefore, we compare the stability of macroaggregates and varying soil properties in topsoil with or without biocrust cover (BSC+ or BSC−) along a climatic gradient from arid to humid climate conditions along the Chilean Coastal Range. We test the following hypotheses:
- i.
If biocrusts cover the soil surface, soil aggregates show higher stability because the biocrusts protect the soil surface physically, shelter soil organic matter within aggregates, modify the structure of microbial communities, and change water flow into the soil.
- ii.
If the climate is arid, the effect of biocrusts on the soil surface is most pronounced because other sources of organic matter are at minimum, and biocrusts represent the main soil cover.
- iii.
If the humidity of the climate increases, the stabilizing effects of biocrusts are reduced, although without disappearing entirely, because water availability increases and higher vegetation hinder the growth of biocrusts.
2.1 Study sites and experimental settings
In order to assess the climatic effect on soil and its interactions with biocrusts, four study sites distributed between latitudes from 26∘06′ to 37∘48′ S and over 1300 km were established in the Chilean Coastal Range: Pan de Azúcar National Park (PA), Santa Gracia Natural Reserve (SG), La Campana National Park (LC) and Nahuelbuta National Park (NA), corresponding to arid, semi-arid, Mediterranean, and humid climates, respectively (Bernhard et al., 2018).
The study sites are comparable in geology, geomorphology, land use, and glacial and volcanic influence (Bernhard et al., 2018). The parent material in all the study sites is granitoid, keeping this factor of soil formation nearly constant along the studied gradient (Oeser et al., 2018). The dominant topography is generally fluvial valleys, and the sites had no glacial influence during the last glaciation (Hulton et al., 2002). The sites are located within protected natural areas, with limited anthropogenic influence compared to the surrounding areas. Despite this, the occasional entry of cows to LC (Rundel and Weisser, 1975) and goats to SG (Armesto et al., 2007) has been reported. These conditions allow us to focus on the environmental effect on two other soil-forming factors, i.e., climate and vegetation.
The mean annual temperature (MAT) decreases from north to south (PA: 16.8 ∘C, SG: 13.7 ∘C, LC: 14.1 ∘C, NA: 6.6 ∘C). The mean annual precipitation (MAP) in the study sites increases from north to south (PA: 12 mm yr−1, SG: 66 mm yr−1, LC: 367 mm yr−1, NA: 1469 mm yr−1) with similar rainfall distribution mostly concentrated in winter months (May to August) (Bernhard et al., 2018). The elevation of the sites increases from north to south (PA: 329–351 , SG: 642–720 , LC: 708–732 , NA: 1200–1270 ). Paleoclimate modeling studies (Mutz et al., 2018) indicate that these climate patterns have been persistent since the late Pliocene; thus, the study sites represent the long-term impact of climate on the soil (Ewing et al., 2006). Bernhard et al. (2018) classified soils in the study sites as Regosols in PA, Cambisols for SG and LC, and Umbrisols in NA. In general, pedogenic processes such as soil depth, clay contents, organic matter accumulation, porosity, and activity ratio are correlated with the humidity of the site.
For each of the four study sites, five plots of 1 m × 1 m were established as replicates. Each plot was located in the top-slope position with south-facing exposition, considering the presence of site-typical biocrust communities, similar slope and aspect, lack of anthropogenic disturbance, and a maximum distance of 30 m between each plot. Each plot included patches with at least the size of the samples with 100 % biocrust cover (BSC+), and additionally, a nearby point without biocrust cover (BSC−) was defined as control.
2.2 Biocrust sampling and classification
Biocrust patches of approximately 100 cm2 were identified according to Lange and Belnap (2016) and collected in the field by carefully detaching the biocrust layer, removing the loose soil, and storing it in paper envelopes after air-drying for every research plot. Samolov et al. (2020) describes the biocrust dominance in PA with cover of up to 40 %. The other study sites are dominated by higher vegetation that limits the cover of biocrust by up to 15 % in SG and 5 % in LC and NA. Sampled communities showed all typical biocrust classes from cyanobacteria, algae, fungi, lichens, liverworts, and mosses (Fig. 1). The species composition further showed a graduating change from lichen-dominating biocrusts in the northernmost site to bryophyte-dominating biocrusts in the southernmost site. Biocrusts in NA were specifically found in zones of forest soil disturbance. Bryophytes were sampled with rhizoids down to 5 mm depth; all other species were down to 2 mm. Dominant macroscopic biocrust species were determined for each of the four sites to the genus level by morphological characteristics using a stereomicroscope (Leitz TS, Wetzlar, Germany), a transmitted light microscope (Leitz Laborlux S, Wetzlar, Germany), and an ultraviolet light. Species groups were separated into bryophytes (Lightowlers, 1985; He, 1998; Ochyra and Matteri, 2001; Cuvertino et al., 2012; Fariña and Ardiles, 2014) and lichens (Galloway and Quilhot, 2009) and assigned to the different regions (Table 1). Baumann et al. (2018), based on morphological identification of enrichment cultures, reported that the biocrusts of all studied areas were composed of 18 to 15 species of algae and cyanobacteria, respectively, where the richness of green algae increased, while the richness of cyanobacteria decreased with increasing humidity and decreasing MAT. Samolov et al. (2020), based on morphological and molecular traits, reported 18 species in PA, 26 species in SG, 40 species in LC, and 27 species in NA. A more detailed survey and classification of individual species, including algae and cyanobacteria, will be sought for further studies.
2.3 Soil sampling and analyses
For soil characterization, bulk topsoil samples (0–5 cm) were taken with metal-core sample augers directly under biocrust patches and in comparative zones without biocrust cover and sieved to 2 mm after air-drying. Bulk density (BD) and soil water content were determined gravimetrically. The particle size distribution was determined for 7 fractions according to Köhn (1929), combining sieving of fractions > 20 µm and pipetting of fractions < 20 µm. Soil texture was interpreted according to the World Reference Base (WRB) soil classification system (Jahn et al., 2006). Soil pH was determined in water by a WTW pH 340 (WTW GmbH, Weilheim, Germany) using a Sentix 81 electrode, and electrical conductivity was measured with a conductivity meter (LE703, Mettler Toledo, USA). Total carbon (Ct) and nitrogen (Nt) of the bulk topsoil samples (0–5 cm) were analyzed using oxidative heat combustion at 1150 ∘C in a Vario EL III elemental analyzer (Elementar Analysensysteme GmbH, Hanau, Germany). Total organic carbon (TOC) was corrected by the carbonate content of samples with pH > 6.7. The carbonate content was determined from the volumetric titration of the reaction with 10 % HCl using a calcimeter (Eijkelkamp, Giesbeek, the Netherlands).
The physical stability of soil aggregates was measured to quantify the destructive effect of water and mechanical forces through two-stage sieving: dry and wet (Hartge and Horn, 2009). Water-stable aggregates (WSAs) were measured by sieving 200 g of undisturbed, air-dried soil samples, homogenized to 30.0 mm, through a stack of sieves of decreasing mesh size (19.0, 14.7, 9.8, 6.8, 4.8, 3.3, 2.0 mm, collecting the remainder below 2.0 mm), and then repeating the same process underwater (Six et al., 2000). Finally, coarse fragments (stones) were removed, and the values for each size were calculated relative to the weight of the initial sample. With this aggregate stability data, accumulated frequency curves were calculated, and a set of stability indexes were estimated: difference in mean weight diameter of the aggregates (ΔMWD) (Hartge and Horn, 2009; Van Bavel, 1950; Loaiza Puerta et al., 2018), difference in geometric mean diameter (ΔGMD) (Mazurak, 1950; Kemper and Rosenau, 1986), water stability aggregate ratio (WSAR) (Liu et al., 2014), and the proportion of soil macroaggregates of a diameter less than 2 mm (R<2 mm) (Liang et al., 2015) as described below. The ΔMWD and ΔGMD indicate how much the average diameter of soil aggregates changes between dry and wet conditions. The main difference between ΔMWD and ΔGMD is that the former considers a linear behavior between the different aggregate size classes, while the ΔGMD considers a logarithmic fitting.
Difference in mean weight diameter (ΔMWD):
where Wi is the corrected mass proportion of stable aggregate fraction i in the total 2–30 mm aggregate fractions, and Xi is the mean diameter of stable aggregate fraction i.
Difference in geometric mean diameter (ΔGMD):
where Xi is the sieve opening size (mm), Wi is the proportion of the total sample mass occurring in the i-size fraction, and n is the number of particle fractions.
Water stability aggregate ratio (WSAR):
where WSA is the > 2 mm water-stable aggregate content, and A is the > 2 mm dry aggregate content.
Proportion of soil macro-aggregate of a diameter less than 2 mm (R<2 mm):
where Wr<2 is the weight of macroaggregates with a diameter less than 2 mm, WT is the total sample weight, Wr<2 is the weight of micro-aggregates with a diameter less than 2 mm.
2.4 Statistical analyses
The influence of the climatic gradient (study site) and biocrust presence on physicochemical soil parameters and aggregate stability in 40 plots (4 study sites, 2 biocrust treatments, 5 replicates) were assessed by factorial generalized linear models (GLMs) because of the lack of normal distribution for most of the variables according to the Shapiro–Wilk test. The link functions used for each model were selected based on the lowest Akaike information criterion (AIC) selection and characteristics of the data (skewness, counts, continuous variables, proportions) between Gaussian, Gamma, inverse Gaussian, and Tweedie distributions. Differences in treatments were tested using Tukey's post-hoc test with p < 0.05 as significance criteria. The analyses were conducted in R 4.2.0 (R Core Team, 2018), and the GLM distributions were extended from the base R core with the Tweedie 2.3.3 package (Dunn, 2017). All visualizations were made with the package ggplot2 3.3.3 (Wickham, 2016).
3.1 Soil properties
Soil pH was significantly affected by the climatic gradient (Fig. 2), with mean values of 7.7 in PA, 6.2 in SG, 5.9 in LC, and 4.4 in NA, with acidification levels of 6.2 in BSC− to 5.9 in BSC+. In terms of electrical conductivity (EC), a remarkably higher value of 2646.1 µS cm−1 in PA is outstanding in comparison with the low and homogeneous values of 109.3 µS cm−1 for SG, 153.8 µS cm−1 for LC, and 102.3 µS cm−1 for NA. EC did not differ for the biocrust treatment. Nevertheless, when looking at the site and biocrust in combination, the BSC+ results in a reduction of the EC in PA; meanwhile, , there was no noticeable change in SG, LC, and NA.
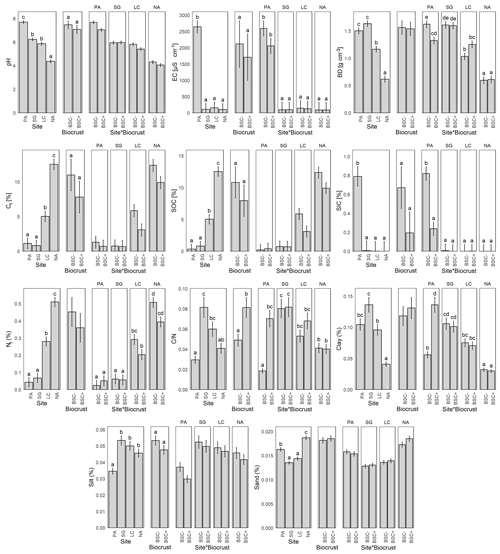
Figure 2Mean and standard error of soil properties for Pan de Azúcar (PA), Santa Gracia (SG), La Campana (LC), and Nahuelbuta (NA), without (BSC−) and with biocrust cover (BSC+), and the interaction of both factors. EC = electrical conductivity, BD = bulk density, Ct = total carbon, SOC = soil organic carbon, SIC = soil inorganic carbon, Nt = total nitrogen, CN = carbon to nitrogen ratio. Significant factors include Tukey's post-hoc test indicated by letters on the top of the bars. Different letters represent significant differences at p < 0.05.
Bulk density (BD) showed a significant difference between the study sites, with higher values in the two dryer sites, with 1.5 g cm−3 in PA, 1.6 g cm−3 in SG, and a decrease in the more humid sites, with 1.2 g cm−3 in LC and 0.6 g cm−3 in NA. Biocrust showed no changes for BD in SG and NA, but in PA BSC+ resulted in a reduction of the BD of 18.2 %, while in LC BSC+ showed an increase in BD of 21.9 % (Fig. 2).
The total carbon (Ct) content was directly proportional to the humidity along the climatic gradient (Fig. 2), with values of 1.1 % in PA, 0.8 % in SG, 5.0 % in LC, and 12.5 % in NA, but with a significant decrease when the BSC+ is present, from 5.6 % to 4.2 % on average. Soil inorganic carbon (SIC) was significantly higher in PA, with an average of 0.8 % of the soil mass, while in SG, LC, and NA, it was not found. When looking at the SIC along the different sites and under biocrust in combination, the BSC+ results in a reduction of 70.1 % in the SIC for PA, while there was no change in SG, LC, and NA. Soil organic carbon (SOC) showed a similar pattern as Ct, with a reduction in PA to 0.3 % and the same 0.8 % in SG, 5.0 % in LC, and 12.5 %. The total nitrogen (Nt) content was directly proportional to the humidity along the climatic gradient, with values of 0.04 % for PA, 0.07 % for SG, 0.28 % for LC, and 0.51 % for NA (Fig. 2); BSC+ showed a reduction of 30.3 % in LC and 22.3 % in NA, while the Nt content remained stable in PA and SG under the influence of BSC. The relation between Ct and Nt, expressed as , showed significantly different values of 33.9 in PA, 12.3 in SG, 16.7 in LC, and 24.5 in NA on average; and with a significant decrease from 27.0 in BSC− to 16.7 in BSC+ (Fig. 2). It is important to note that although PA and NA present the highest values, the condition changes diametrically when observed together with the BSC+ treatment, with a large dispersion in PA and stable values in NA.
The distribution of the soil particle size classes did not show clear patterns along the climatic gradient, with PA deviating from it in all cases. Despite this, the observed values were significant, with clay values of 9.6 % for PA, 7.3 % in SG, 10.4 % in LC, and 24.6 % in NA. However, when looking at the interaction between site and biocrust, there is an increase of 143.4 % in clay content for the BSC+ condition, while no difference was observed for SG, LC, and NA. The observed values for silt are as follows: 28.9 % in PA, 18.7 % in SG, 20.0 % in LC and 21.9 % in NA. The values for sand are as follows: 61.5 % in PA, 73.9 % in SG, 69.6 % in LC, and 53.5 % in NA. When biocrusts were present, a significant decrease from 13.6 % to 12.6 % in clays and an increase from 21.2 % to 23.6 % in silt was observed, with higher dispersion for the arid site (PA) (Fig. 2).
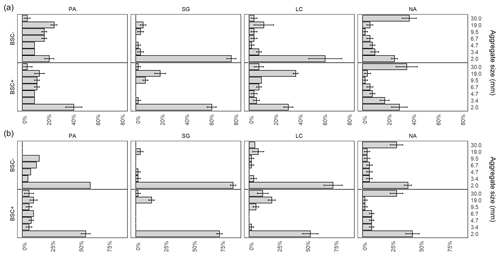
Figure 3Mean value and standard error of aggregate proportion for dry (a) and wet (b) sieved fractions for Pan de Azúcar (PA), Santa Gracia (SG), La Campana (LC), and Nahuelbuta (NA) for biocrust (BSC+) and non-biocrust (BSC−) treatments. Significant factors and corresponding models for response variables are included in Appendix A.
3.2 Soil aggregate stability
Dry sieving showed a significant difference between study sites but not for the biocrust treatment (Fig. 3). Dry aggregates in the 19.0–30.0 mm range showed significantly different values of 4.4 % in PA, 1.2 % in SG, 5.5 % in LC, and 36.2 % in NA. The fraction 9.5–19.0 mm revealed differences in the interaction between study sites and biocrusts, increasing the proportion of aggregates from 13.2 % in PA to 19.3 % in SG and 29.3 % in LC; this increase was not in NA with only 2.9 % in the presence of biocrusts. The aggregates between 6.7–9.5 mm showed a significant decrease from 13.3 % in PA, 5.2 % in SG, 6.5 % in LC, and 4.1 % in NA. When interacting with biocrusts, it showed a significant increase in the proportion when it was present. The fraction from 4.7–6.7 mm showed significantly different values of 13.6 % in PA, 3.4 % in SG, 5.0 % in LC, and 5.6 % in NA, while the interaction with biocrusts showed a significant increase when it is present (BSC+). Aggregates from 3.4–4.7 mm in size showed significance among study sites, with 9.8 % in PA, 3.6 % in SG, 4.4 % in LC, and 7.9 % in NA. Aggregates between 2.0–3.4 mm showed a slightly similar amount of 9.9 % in PA, 5.9 % in LC, and 14.3 % in NA, but with a minor proportion of 4.9 % in LC. However, when biocrust is present in LC, it showed a slight decrease. Finally, for the dry sieved aggregates under 2 mm, there was a significant reduction for the study sites, with values of 69.1 % in SG and 47.3 % in LC relative to 30.1 % in PA, but not in NA with 28.9 %; the biocrust effect in interaction with the site is significant with 60.6 % in SG and 40.8 % in LC, indicating the same proportion of aggregates in this site for SG with 30.0 % and NA with 39.8 %.
In a second stage, aggregate stability under wet conditions was characterized, with a clear difference between sites, while biocrusts had a significant effect only on the aggregate size classes < 2.0 and 9.5–30.0 mm (Fig. 3). At the same time, the fraction 19.0–30.0 mm showed an increasing significant pattern in the amount of aggregates, with 2.8 % in NA, 0.8 % in SG, 8.9 % in LC, and 29.9 % in NA, while biocrusts significantly increased the proportion of aggregates by 35.6 %, from 9.0 % for BSC− to 12.2 % for BSC+. For the fraction 9.5–19.0 mm, there were significant differences between the study sites, the biocrust effect, and its interactions, with an increase from 5.4 % to 11.7 % on average when biocrusts were present. The wet sieved aggregates in the range of 6.7–9.5 mm showed significant differences only between the study sites, with 10.6 % in PA, 2.5 % in SG, 4.2 % in LC, and 4.3 % in NA. Wet aggregates in the range of 4.7–6.7 mm showed significant differences between the study sites with 10.6 % in PA, 2.3 % in SG, 3.7 % in LC, and 5.8 in NA. The fraction between 3.4–4.7 mm showed only significant differences between the study sites, with 6.9 % in PA, 2.2 % in SG, 3.0 % in LC, and 6.1 % in NA. Aggregates ranging from 2.0–3.4 mm showed differences between the study sites, with 5.8 % in PA, 3.2 % in SG, 4.2 % in LC, and 6.8 % in NA. The fractions under 2.0 mm were significantly different for the study sites, with 58.3 % in PA, 79.6 % in SG, 62.4 % in LC, and 41.3 % in NA, while for biocrusts, the values ranged from 63.9 % for BSC+ treatment to 56.9 % for BSC− treatment.
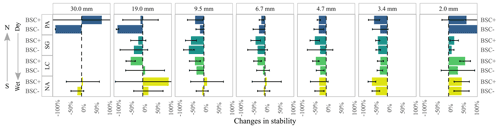
Figure 4Mean and standard deviation of aggregate size distribution changes between dry and wet sieving for the sites Pan de Azúcar (PA), Santa Gracia (SG), La Campana (LC), and Nahuelbuta (NA) for biocrust (BSC+) and non-biocrust (BSC−) treatments. Error bars were, in some cases, truncated for better visualization.
When comparing the changes of the aggregate distributions between wet and dry conditions (Fig. 4, Mean and standard deviation), an irregular pattern was observed, with a general decrease in most of the analyzed fractions, except for an increase in the amount of aggregates of 19.0 mm for NA. This was even higher than for the BSC+ treatment. It is important to mention that NA also showed a slight increase in the proportion of 9.5 and 6.7 mm aggregates.
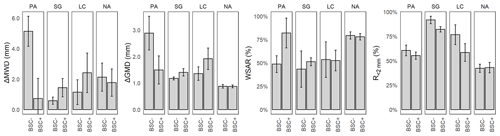
Figure 5Mean and standard error of aggregate stability indexes for Pan de Azúcar (PA), Santa Gracia (SG), La Campana (LC), and Nahuelbuta (NA) for biocrust (BSC+) and non-biocrust (BSC−) treatments. ΔMWD = difference in mean weight diameter, ΔGMD = difference in geometric mean diameter, WSAR = water stability aggregate ratio, R<2 mm = ratio of aggregates less than 2 mm. Significant factors and corresponding models for response variables are included in Appendix A.
Soil aggregate stability was evaluated through different indexes to integrate the different sizes and sieving conditions in a summary value. The difference in mean weight diameter of the aggregates (ΔMWD) showed no significance in any of the conditions (Fig. 5). However, there was a significant difference in the difference of the geometric mean diameter of the aggregates (ΔGMD) for the study sites, with values of 1.86 mm for PA, 1.2 mm for SG, 1.4 mm for LC, in comparison with the more stable condition of 0.83 mm for NA. The WSAR was significant for the study sites, showing differences between NA with 81.1 % and the other study sites (57.7 % PA, 66.2 % SG, and 73.4 % LC). The ratio of soil macroaggregates of a diameter less than 2.0 mm (R<2 mm) presents differences in the study sites and for biocrust treatments. SG showed a value of R<2 mm of 79.6 % and NA of 41.9 %, which was different from PA and LC, with 58.6 % and 62.4 %, respectively. For biocrust treatments, R<2 mm changed from 63.7 % to 57.5 % with biocrusts, indicating a biocrust-induced decrease in the proportion for this fraction. Finally, according to the analyzed indexes, NA showed the most stable conditions, and alternating PA and SG showed the most unstable conditions.
As shown in Fig. 2, the climatic gradient (site) had significant pH, electrical conductivity (EC), bulk density (BD), total carbon (Ct), soil organic carbon (SOC), soil inorganic carbon (SIC), total nitrogen (Nt), , clay, silt, sand, dry and wet aggregates under 30 mm, ΔGMD, WSAR, and R<2 mm. Biocrust treatments were significant for clay, silt, pH, EC, Ct, SOC, SIC, Nt, , and wet aggregates from 9.5 to 30.0 mm, > 2 mm, and R<2 mm. Finally, the interaction of the site and biocrusts was significant for clay, BD, Nt, dry aggregates from 4.7 to 19.0 mm and 0 to 3.4 mm, wet aggregates from 9.5 to 30.0 mm, and ΔMWD.
4.1 Aggregate stability and soil properties along the climatic gradient
The climatic gradient has a significant effect on the stability of soil aggregates. Using the geometric mean diameter (ΔGMD), an index that replaces the linear fitting of ΔMWD with a logarithmic one, significant differences for the study sites along the climatic gradient can be observed (p value: < 0.001). When soil aggregate stability was evaluated with the difference in mean weight diameter (ΔMWD), it did not show significant changes along the climatic gradient. The WSAR, an index that shows the ratio of aggregates that persist stably after disruption by water, showed a similar behavior as ΔMWD. The main difference between ΔMWD and ΔGMD is that ΔGMD performs better in non-uniform particulate substances (Hatch and Choate, 1929; Gardner, 1956), which corresponds to soils equilibrated in the content of sand, silt, and clay (Mazurak, 1950) and indirectly marking soil texture as a critical factor in aggregate stability along the climatic gradient. Further, considering the ΔGMD data, an increase in stability was observed when moving along the climatic gradient to higher water availability conditions, except for SG. The lower value of ΔGMD for SG indicates comparably higher aggregate stability as it would be expected when we assume a steady trend from arid to humid climate. In PA, in the drier north, the condition proved to be less stable than NA, despite the high content of inorganic carbon. In SG, the highest ratio of unstable aggregates under the studied range of sizes (highest R<2 mm) was presented; the lowest ratio was presented in NA, with close to half of it indicating augmented aggregate stability in the complementary range of sizes.
The effect of the climatic gradient is not only expressed in the stability of soil aggregates, but it is also present with different intensities in a variety of soil properties. The pH decreases continuously from the northern arid to the southern humid study site in accordance with Bernhard et al. (2018). The high pH in PA can be attributed to the constant input of atmospheric aerosols, e.g., salts, gypsum, and calcium carbonates (Ewing et al., 2006) in combination with the arid climate that allows salts to accumulate in the topsoil (Slessarev et al., 2016). Whereas in the southern sites, the forwarding increase of precipitations results in a reduction in the pH due to leaching of soluble salts (Slessarev et al., 2016) and an increase in soil respiration (Orchard and Cook, 1983). The accumulation of soluble salts is well established for the arid site PA, as saline conditions (Allison and Richards, 1954) are indicated by the high EC value. These higher amounts of salts have a strong effect in structure degradation dynamics, linked to the destabilizing effect of sodium and stabilizing of carbonates (Corwin, 2021). Although Ct and Nt follow the climatic gradient, when comparing the , PA and NA have higher values. High values of the indicate a nitrogen limitation of plants and other organisms (Brust, 2019), pointing out that this occurs at the two opposite sites along the climatic gradient. This could be explained by the biological activity (Zhang et al., 2013), which may be close to a physiological limitation in the case of NA, while for PA, it may indeed be due to low nitrogen availability (Hooper and Johnson, 1999). However, there was also a high amount of carbonates in PA, which makes PA hardly comparable in terms of Ct. Despite the properties following the climatic gradient, SG deviates from the other sites in terms of higher BD, lower clay, and higher sand content. This can indicate a degraded condition for the semi-arid site caused by the current land use, including grazing (Armesto et al., 2007), compacting the surface and thus activating erosive processes (Scholten and Seitz, 2019), in favor of the accumulation of sand particles (Govers, 1985). The aggregate distribution stresses this finding, where SG has a lower proportion of water-stable aggregates > 2 mm and a higher water-destabilization of aggregates between 9.5 to 4.7 mm, indicating that the nature of the structuring agent in that zone is water-soluble.
4.2 Biocrusts altering soil properties along the climatic gradient
Despite these factors beyond the climatic gradient, biocrusts showed effects on clay, silt, pH, Ct, Nt, , and wet aggregates from 9.5 to 30.0 mm and > 2 mm. However, as this was an observational study, it only allows the establishment of associations between factors and not cause–effect relationships (Cox, 1992; Rosenbaum, 2005). It is thus possible that changes in soil characteristics promote the biocrust establishment, as well as that biocrust establishment triggers changes in these properties (Belnap and Lange, 2003).
The biocrust treatments showed a significant decrease in pH (p value: 0.002404), reflecting the biological activity of its constituent organisms, which acidifies the soil due to the carbon dioxide released by cellular respiration (Bachar et al., 2010). The pH values reported by Bernhard et al. (2018) are in the same range as ours but without differentiating between BSC+ and BSC−, as this factor was not part of their study. The content of Ct and Nt were significantly different when biocrusts were present, but it did not affect any aggregate sizes or stability indexes. In this sense, biocrusts play a role in the carbon and nitrogen cycles (Chen et al., 2000) as they are formed mainly by photosynthetic and nitrogen-fixing organisms (Maestre et al., 2013), but it has not had an immediate impact on the soil aggregate stability and points out that the primary stabilizing agent is of organic origin (Wagner et al., 2007; Six et al., 2004).
Considering the stabilizing effect of biocrusts on wet sieved aggregates between 9.5 and 30.0 mm, we could show that it occurs prominently at the three northern sites, whereas in NA, there was no difference with and without biocrusts. This points to a threshold in the biocrust-induced stabilization of the soil aggregates between LC and NA and partially confirms our initial assumption that biocrusts have the greatest effect in arid conditions. However, the effect on aggregate stability for the wet condition varies according to the variable used, being specific for limited aggregate sizes in terms of mechanical disturbances (dry sieving) but without a substantial improvement concerning water stability (wet sieving). This lack of difference in wet sieved aggregate points to a non-soluble nature of the stabilizing agents, which can be attributed to stabilization due to organic structures and exudates (Rillig, 2004), and stresses the idea that NA differs from the other sites in the mechanisms of aggregate stabilization as a local adaptation which is conducted by water stable mechanisms due to the higher proportion of precipitation.
Soil aggregate stability showed clear differences along the climatic gradient. However, when considering the effect of biocrusts, differences were limited to the smallest aggregate size class (R<2 mm), referring to changes in micro-aggregate size distribution as described by Totsche et al. (2018). Further, a difference for wet sieved aggregates with and without biocrusts between 9.5 and 30.0 mm point to biocrust-induced stabilization of soil aggregates.
The results indicate that soil aggregate stabilization mechanisms are different in PA than in the other sites. With that in mind, it was found that in PA, biocrusts grow in areas with a lower content of clay and higher content of silt, which implies increased nutrient availability and water-holding capacity (Chen et al., 2000), while the sand fraction was not related. However, the method used can amplify that difference since the determination of particle size distribution does not consider coarse fragments (Köhn, 1929) which were abundant at PA. In addition, the soil covered with biocrusts showed a lower value for BD only for PA, while in the other sites, this property was not affected. This can be interpreted as a biocrust-induced decrease in soil density due to increased intra- and extra-aggregate porosity and organic matter (Whitney et al., 2017), or that biocrusts grow under the least-limiting condition (Bowker et al., 2014). Soils with biocrust cover showed a trend of lower EC, which can be explained by the inhibition of biocrusts by toxicity due to the accumulation of salts in the soil, or to the consumption of salts as a source of nutrients by the organisms in the biocrusts (Abed et al., 2019).
Biocrust plays a role along the climatic gradient affecting different properties, i.e., clay, silt, pH, Ct, Nt, , and wet aggregates from 9.5 to 30.0 mm and > 2 mm. Nevertheless, the way that each property change responds to local conditions can be described as follows: in the arid northernmost site, there is a strong influence of the salts in terms of stabilization and establishment of biocrusts, while at the southernmost site, there is no stabilization of the aggregates, but a contribution to the carbon and nitrogen contents. The most consistent property along the climatic gradient was pH, an indicator of biological activity. However, at the scale of the climatic gradient, it is not possible to distinguish the origin of biological activity between plants, microorganisms, fungi, bacteria, etc. Finally, considering the largest size of the persistent wet aggregates match the characteristics attributed to fungi and bryophytes, capable of retaining micro-aggregates and soil particles between their hyphae and rhizoids, respectively (Kleber et al., 2007; Six et al., 2004; Totsche et al., 2018), and points to this as the most significant mechanism of soil aggregate stabilization of biocrusts.
This study aimed to investigate how and to what extent biocrusts stabilize soil aggregates along an arid to humid climatic gradient in Chile. We show that biocrusts play a role in soil aggregate stability along the climatic gradient by increasing the stability for wet aggregates from 9.5 to 30.0 mm and > 2 mm. Biocrusts also modify other soil properties such as Ct, Nt, , clay, and sand, indicating an initial accumulation of organic matter that then give place to aggregation processes. The effect of biocrusts on aggregate stability was lower under humid climate conditions, which indicates a transition in the main biotic agents driving the aggregation of the soil surface, moving from biocrust communities in arid regions to vascular plants in humid conditions.
Finally, we conclude that the biocrusts in our study area proved to be a valuable agent in stabilizing the upper topsoil layer, but for a narrow spectrum of conditions and mostly under arid conditions. Therefore, the effect could be considered a transition in ecological succession toward a stable ecosystem. In this process, biocrusts improve conditions for other more demanding species, such as vascular plants, initially improving the availability of carbon and nitrogen in the soil.
The code that supports the findings of this study are available from the corresponding author upon request.
The data that support the findings of this study are available from the corresponding author upon request.
TS, DW, CWM, OS, and StS conceptualized the study. OS, KW, NRM, and RO collected the soil samples. StS collected and analyzed the biocrust samples, and NRM analyzed the soil samples. NRM performed the analyses and prepared the manuscript. PK and VR gave feedback to improve the paper.
The contact author has declared that none of the authors has any competing interests.
Publisher's note: Copernicus Publications remains neutral with regard to jurisdictional claims in published maps and institutional affiliations.
We are deeply grateful to the students, technical assistants, and colleagues who helped in the field and laboratory phases. A special thanks to Martin Nebel and Sonja Thielen for their help in classifying bryophytes and lichens. Oliver Burkhardt (GFZ German Research Centre for Geosciences) and Franziska Steiner (Technical University of Munich) for their support in the field. Hugo Pérez for his help and experience in the soil physics laboratory of the Universidad de Chile. We are grateful to the Chilean National Forest Corporation (CONAF) and Sucesión Gálvez Muñoz, who provided access to the study sites. We acknowledge support by the Open Access Publishing Fund of the University of Tübingen.
The study was funded by the German Science Foundation DFGSPP 1803 (EarthShape; http://www.earthshape.net, last access: 30 November 2022).
This open-access publication was funded
by the University of Tübingen.
This paper was edited by Cornelia Rumpel and reviewed by two anonymous referees.
Abed, R. M. M., Tamm, A., Hassenrück, C., Al-Rawahi, A. N., Rodríguez-Caballero, E., Fiedler, S., Maier, S., and Weber, B.: Habitat-dependent composition of bacterial and fungal communities in biological soil crusts from Oman, Sci. Rep.-UK, 9, 6468, https://doi.org/10.1038/s41598-019-42911-6, 2019.
Allison, L. E. and Richards, L. A.: Diagnosis and improvement of saline and alkali soils (No. 60), Soil and Water Conservative Research Branch, Agricultural Research Service, US Department of Agriculture, https://www.ars.usda.gov/ARSUserFiles/20360500/hb60_pdf/hb60complete.pdf (last access: 30 November 2022), 1954.
Armesto, J. J., Arroyo, M. T., and Hinojosa Opazo, L.: The Mediterranean environment of central Chile, in: The Physical Geography of South America, edited by: Veblen, T., Young, K., and Orme, A., Oxford University Press, 184–199, https://doi.org/10.1093/oso/9780195313413.003.0019, 2007.
Bachar, A., Al-Ashhab, A., Soares, M. I. M., Sklarz, M. Y., Angel, R., Ungar, E. D., and Gillor, O.: Soil Microbial Abundance and Diversity Along a Low Precipitation Gradient, Microb. Ecol., 60, 453–461, https://doi.org/10.1007/s00248-010-9727-1, 2010.
Barger, N. N., Weber, B., Garcia-Pichel, F., Zaady, E., and Belnap, J.: Patterns and controls on nitrogen cycling of biological soil crusts, in: Biological soil crusts: an organizing principle in drylands, edited by: Weber, B., Büdel, B., and Belnap, J., Springer, 257–285, https://doi.org/10.1007/978-3-319-30214-0_14, 2016.
Baumann, K., Jung, P., Samolov, E., Lehnert, L. W., Büdel, B., Karsten, U., Bendix, J., Achilles, S., Schermer, M., Matus, F., Oses, R., Osses, P., Morshedizad, M., Oehlschläger, C., Hu, Y., Klysubun, W., and Leinweber, P.: Biological soil crusts along a climatic gradient in Chile: Richness and imprints of phototrophic microorganisms in phosphorus biogeochemical cycling, Soil Biol. Biochem., 127, 286–300, https://doi.org/10.1016/j.soilbio.2018.09.035, 2018.
Belnap, J. and Büdel, B.: Biological soil crusts as soil stabilizers, in: Biological soil crusts: an organizing principle in drylands, edited by: Weber, B., Büdel, B., and Belnap, J., Springer, 305–320, https://doi.org/10.1007/978-3-319-30214-0_16, 2016.
Belnap, J. and Lange, O. L. (Eds.): Biological soil crusts: structure, function, and management, 1, Ecological Studies, Springer, Berlin Heidelberg, https://doi.org/10.1007/978-3-642-56475-8, 2003.
Belnap, J., Weber, B., and Büdel, B. (Eds.): Biological soil crusts as an organizing principle in drylands, in: Biological soil crusts: an organizing principle in drylands, Springer, 3–13, https://doi.org/10.1007/978-3-319-30214-0_1, 2016.
Bernhard, N., Moskwa, L.-M., Schmidt, K., Oeser, R. A., Aburto, F., Bader, M. Y., Baumann, K., von Blanckenburg, F., Boy, J., van den Brink, L., Brucker, E., Büdel, B., Canessa, R., Dippold, M. A., Ehlers, T. A., Fuentes, J. P., Godoy, R., Jung, P., Karsten, U., Köster, M., Kuzyakov, Y., Leinweber, P., Neidhardt, H., Matus, F., Mueller, C. W., Oelmann, Y., Oses, R., Osses, P., Paulino, L., Samolov, E., Schaller, M., Schmid, M., Spielvogel, S., Spohn, M., Stock, S., Stroncik, N., Tielbörger, K., Übernickel, K., Scholten, T., Seguel, O., Wagner, D., and Kühn, P.: Pedogenic and microbial interrelations to regional climate and local topography: New insights from a climate gradient (arid to humid) along the Coastal Cordillera of Chile, CATENA, 170, 335–355, https://doi.org/10.1016/j.catena.2018.06.018, 2018.
Bowker, M. A., Maestre, F. T., Eldridge, D., Belnap, J., Castillo-Monroy, A., Escolar, C., and Soliveres, S.: Biological soil crusts (biocrusts) as a model system in community, landscape and ecosystem ecology, Biodiversity and Conservation, 23, 1619–1637, https://doi.org/10.1007/s10531-014-0658-x, 2014.
Bowker, M. A., Belnap, J., Büdel, B., Sannier, C., Pietrasiak, N., Eldridge, D. J., and Rivera-Aguilar, V.: Controls on Distribution Patterns of Biological Soil Crusts at Micro- to Global Scales, in: Biological Soil Crusts: An Organizing Principle in Drylands, edited by: Weber, B., Büdel, B., and Belnap, J., Springer International Publishing, Cham, 173–197, https://doi.org/10.1007/978-3-319-30214-0_10, 2016.
Brust, G. E.: Chapter 9 – Management Strategies for Organic Vegetable Fertility, in: Safety and Practice for Organic Food, edited by: Biswas, D. and Micallef, S. A., Academic Press, 193–212, https://doi.org/10.1016/B978-0-12-812060-6.00009-X, 2019.
Chamizo, S., Belnap, J., Eldridge, D. J., Cantón, Y., and Issa, O. M.: The role of biocrusts in arid land hydrology, in: Biological soil crusts: An organizing principle in drylands, edited by: Weber, B., Büdel, B., Belnap, J., Vol. 226, Springer, Cham., 321–346, https://doi.org/10.1007/978-3-319-30214-0_17, 2016.
Chen, J., Blume, H.-P., and Beyer, L.: Weathering of rocks induced by lichen colonization – a review, CATENA, 39, 121–146, https://doi.org/10.1016/S0341-8162(99)00085-5, 2000.
Chen, N., Wang, X., Zhang, Y., Yu, K., and Zhao, C.: Ecohydrological effects of biological soil crust on the vegetation dynamics of restoration in a dryland ecosystem, J. Hydrol., 563, 1068–1077, https://doi.org/10.1016/j.jhydrol.2018.06.076, 2018.
Chen, N., Yu, K., Jia, R., Teng, J., and Zhao, C.: Biocrust as one of multiple stable states in global drylands, Science Advances, 6, eaay3763, https://doi.org/10.1126/sciadv.aay3763, 2020.
Colesie, C., Green, T. A., Haferkamp, I., and Buedel, B.: Habitat stress initiates changes in composition, CO 2 gas exchange and C-allocation as life traits in biological soil crusts, ISME J., 8, 2104–2115, https://doi.org/10.1038/ismej.2014.47, 2014.
Corwin, D. L.: Climate change impacts on soil salinity in agricultural areas, Eur. J. Soil Sci., 72, 842–862, https://doi.org/10.1111/ejss.13010, 2021.
Cox, D. R.: Causality: Some Statistical Aspects, J. R. Stat. Soc. A Stat., 155, 291–301, https://doi.org/10.2307/2982962, 1992.
Cuvertino, J., Ardiles, V., Osorio, F., and Romero, X.: New records and additions to the Chilean bryophyte flora, Ciencia e investigación agraria, 39, 245–254, https://doi.org/10.4067/S0718-16202012000200001, 2012.
Drahorad, S. L., Steckenmesser, D., Felix-Henningsen, P., Lichner, L'., and Rodný, M.: Ongoing succession of biological soil crusts increases water repellency—a case study on Arenosols in Sekule, Slovakia, Biologia, 68, 1089–1093, https://doi.org/10.2478/s11756-013-0247-6, 2013.
Dunn, P. K.: Tweedie: Evaluation of Tweedie Exponential Family Models (2.3.0), R package version 2.3.5, https://cran.r-project.org/package=tweedie (last access: 30 November 2022), 2017.
Elbert, W., Weber, B., Burrows, S., Steinkamp, J., Büdel, B., Andreae, M. O., and Pöschl, U.: Contribution of cryptogamic covers to the global cycles of carbon and nitrogen, Nat. Geosci., 5, 459–462, https://doi.org/10.1038/ngeo1486, 2012.
Eldridge, D. J., Zaady, E., and Shachak, M.: Infiltration through three contrasting biological soil crusts in patterned landscapes in the Negev, Israel, CATENA, 40, 323–336, https://doi.org/10.1016/S0341-8162(00)00082-5, 2000.
Eldridge, D. J., Reed, S., Travers, S. K., Bowker, M. A., Maestre, F. T., Ding, J., Havrilla, C., Rodriguez-Caballero, E., Barger, N., Weber, B., Antoninka, A., Belnap, J., Chaudhary, B., Faist, A., Ferrenberg, S., Huber-Sannwald, E., Malam Issa, O., and Zhao, Y.: The pervasive and multifaceted influence of biocrusts on water in the world's drylands, Glob. Change Biol., 26, 6003–6014, https://doi.org/10.1111/gcb.15232, 2020.
Ewing, S. A., Sutter, B., Owen, J., Nishiizumi, K., Sharp, W., Cliff, S. S., Perry, K., Dietrich, W., McKay, C. P., and Amundson, R.: A threshold in soil formation at Earth's arid–hyperarid transition, Geochim. Cosmochim. Ac., 70, 5293–5322, https://doi.org/10.1016/j.gca.2006.08.020, 2006.
Fariña, V. and Ardiles, M.: Flora no vascular de la Región de Arica y Parinacota, Chile: nuevos registros y consideraciones biogeográficas, Boletín del Museo Nacional de Historia Natural, Chile, 63, 213–223, 2014.
Fischer, T., Yair, A., and Veste, M.: Microstructure and hydraulic properties of biological soil crusts on sand dunes: a comparison between arid and temperate climates, Biogeosciences Discuss., 9, 12711–12734, https://doi.org/10.5194/bgd-9-12711-2012, 2012.
Fischer, T., Yair, A., Veste, M., and Geppert, H.: Hydraulic properties of biological soil crusts on sand dunes studied by 13C-CP/MAS-NMR: A comparison between an arid and a temperate site, CATENA, 110, 155–160, https://doi.org/10.1016/j.catena.2013.06.002, 2013.
Galloway, D. J. and Quilhot, W.: Checklist of Chilean lichen-forming and lichenicolous fungi, Gayana Bot., 55, 111–185, 2009.
Gao, L., Bowker, M. A., Xu, M., Sun, H., Tuo, D., and Zhao, Y.: Biological soil crusts decrease erodibility by modifying inherent soil properties on the Loess Plateau, China, Soil Biol. Biochem., 105, 49–58, https://doi.org/10.1016/j.soilbio.2016.11.009, 2017.
Garcia-Pichel, F., Felde, V. J. M. N. L., Drahorad, S. L., and Weber, B.: Microstructure and weathering processes within biological soil crusts, in: Biological soil crusts: an organizing principle in drylands, edited by: Weber, B., Büdel, B., and Belnap, J., Springer, 237–255, https://doi.org/10.1007/978-3-319-30214-0_13, 2016.
Gardner, W. R.: Representation of Soil Aggregate-Size Distribution by a Logarithmic-Normal Distribution, Soil Sci. Soc. Am. J., 20, 151–153, https://doi.org/10.2136/sssaj1956.03615995002000020003x, 1956.
Goebes, P., Seitz, S., Kühn, P., Li, Y., Niklaus, P. A., Oheimb, G. v., and Scholten, T.: Throughfall kinetic energy in young subtropical forests: Investigation on tree species richness effects and spatial variability, Agr. Forest Meteorol., 213, 148–159, https://doi.org/10.1016/j.agrformet.2015.06.019, 2015.
Govers, G.: Selectivity and transport capacity of thin flows in relation to rill erosion, CATENA, 12, 35–49, https://doi.org/10.1016/S0341-8162(85)80003-5, 1985.
Grote, E. E., Belnap, J., Housman, D. C., and Sparks, J. P.: Carbon exchange in biological soil crust communities under differential temperatures and soil water contents: implications for global change, Glob. Change Biol., 16, 2763–2774, https://doi.org/10.1111/j.1365-2486.2010.02201.x, 2010.
Hartge, K. H. and Horn, R.: Die physikalische Untersuchung von Böden, 4th Edn., Schweizerbart Science Publishers, https://www.schweizerbart.de/publications/detail/isbn/9783510652464/Die-physikalische-Untersuchung-von-Bden (last access: 30 November 2022), 2009.
Hatch, T. and Choate, S. P.: Statistical description of the size properties of non uniform particulate substances, J. Frankl. Inst., 207, 369–387, https://doi.org/10.1016/S0016-0032(29)91451-4, 1929.
He, S.: A checklist of the mosses of Chile, J. Hattori Bot. Lab., 85, 103–189, 1998.
Hooper, D. U. and Johnson, L.: Nitrogen limitation in dryland ecosystems: Responses to geographical and temporal variation in precipitation, Biogeochemistry, 46, 247–293, https://doi.org/10.1023/A:1006145306009, 1999.
Hulton, N. R. J., Purves, R. S., McCulloch, R. D., Sugden, D. E., and Bentley, M. J.: The Last Glacial Maximum and deglaciation in southern South America, Quaternary Sci. Rev., 21, 233–241, https://doi.org/10.1016/S0277-3791(01)00103-2, 2002.
Jahn, R., Blume, H., Asio, V., Spaargaren, O., and Schad, P.: Guidelines for soil description, 4th Edn., FAO, https://edepot.wur.nl/27622 (last access: 30 November 2022), 2006.
Jung, P., Emrich, D., Briegel-Williams, L., Schermer, M., Weber, L., Baumann, K., Colesie, C., Clerc, P., Lehnert, L. W., Achilles, S., Bendix, J., and Büdel, B.: Ecophysiology and phylogeny of new terricolous and epiphytic chlorolichens in a fog oasis of the Atacama Desert, MicrobiologyOpen, 8, e894, https://doi.org/10.1002/mbo3.894, 2019.
Jung, P., Baumann, K., Emrich, D., Springer, A., Felde, V. J. M. N. L., Dultz, S., Baum, C., Frank, M., Büdel, B., and Leinweber, P.: Lichens Bite the Dust – A Bioweathering Scenario in the Atacama Desert, iScience, 23, 101647, https://doi.org/10.1016/j.isci.2020.101647, 2020a.
Jung, P., Baumann, K., Lehnert, L. W., Samolov, E., Achilles, S., Schermer, M., Wraase, L. M., Eckhardt, K.-U., Bader, M. Y., Leinweber, P., Karsten, U., Bendix, J., and Büdel, B.: Desert breath—How fog promotes a novel type of soil biocenosis, forming the coastal Atacama Desert's living skin, Geobiology, 18, 113–124, https://doi.org/10.1111/gbi.12368, 2020b.
Kemper, W. and Rosenau, R.: Aggregate stability and size distribution, Methods of soil analysis: Part 1 Physical and mineralogical methods, Agronomy Monograph, 5, 425–442, 1986.
Kleber, M., Sollins, P., and Sutton, R.: A conceptual model of organo-mineral interactions in soils: self-assembly of organic molecular fragments into zonal structures on mineral surfaces, Biogeochemistry, 85, 9–24, https://doi.org/10.1007/s10533-007-9103-5, 2007.
Köhn, M.: Korngrößenanalyse vermittels Pipettanalyse, Tonindustrie-Zeitung, 53, 729–731, 1929.
Lange, O. L. and Belnap, J.: How Biological Soil Crusts Became Recognized as a Functional Unit: A Selective History, in: Biological Soil Crusts: An Organizing Principle in Drylands, edited by: Weber, B., Büdel, B., and Belnap, J., Springer International Publishing, Cham, 15–33, https://doi.org/10.1007/978-3-319-30214-0_2, 2016.
Lewin, R. A.: EXTRACELLULAR POLYSACCHARIDES OF GREEN ALGAE, Can. J. Microbiol., 2, 665–672, https://doi.org/10.1139/m56-079, 1956.
Li, J., Okin, G. S., Alvarez, L., and Epstein, H.: Effects of wind erosion on the spatial heterogeneity of soil nutrients in two desert grassland communities, Biogeochemistry, 88, 73–88, https://doi.org/10.1007/s10533-008-9195-6, 2008.
Liang, T., Shi, X., Guo, T., and Peng, S.: Arbuscular mycorrhizal fungus mediate changes in mycorrhizosphere soil aggregates, Agr. Sci. China, 6, 1455, https://doi.org/10.4236/as.2015.612141, 2015.
Lichner, L'., Holko, L., Zhukova, N., Schacht, K., Rajkai, K., Fodor, N., and Sándor, R.: Plants and biological soil crust influence the hydrophysical parameters and water flow in an aeolian sandy soil/Vplyv rastlín a biologického pôdneho pokryvu na hydrofyzikálne parametre a prúdenie vody v piesoènatej pôde, J. Hydrol. Hydromech., 60, 309–318, https://doi.org/10.2478/v10098-012-0027-y, 2012.
Lightowlers, P.: A synoptic flora of South Georgian mosses: Tortula, Scientific reports-British Antarctic Survey, 41–77, https://nora.nerc.ac.uk/id/eprint/523404 (last access: 30 November 2022), 1985.
Liu, M.-Y., Chang, Q.-R., Qi, Y.-B., Liu, J., and Chen, T.: Aggregation and soil organic carbon fractions under different land uses on the tableland of the Loess Plateau of China, CATENA, 115, 19–28, https://doi.org/10.1016/j.catena.2013.11.002, 2014.
Loaiza Puerta, V., Pujol Pereira, E. I., Wittwer, R., van der Heijden, M., and Six, J.: Improvement of soil structure through organic crop management, conservation tillage and grass-clover ley, Soil Till. Res., 180, 1–9, https://doi.org/10.1016/j.still.2018.02.007, 2018.
Maestre, F. T., Escolar, C., de Guevara, M. L., Quero, J. L., Lázaro, R., Delgado-Baquerizo, M., Ochoa, V., Berdugo, M., Gozalo, B., and Gallardo, A.: Changes in biocrust cover drive carbon cycle responses to climate change in drylands, Glob. Change Biol., 19, 3835–3847, https://doi.org/10.1111/gcb.12306, 2013.
Malam Issa, O., Trichet, J., Défarge, C., Couté, A., and Valentin, C.: Morphology and microstructure of microbiotic soil crusts on a tiger bush sequence (Niger, Sahel), CATENA, 37, 175–196, https://doi.org/10.1016/S0341-8162(99)00052-1, 1999.
Mazurak, A. P.: Effect of gaseous phase on water-stable synthetic aggregates, Soil Sci., 69, 135–148, https://doi.org/10.1097/00010694-195002000-00005, 1950.
Mutz, S. G., Ehlers, T. A., Werner, M., Lohmann, G., Stepanek, C., and Li, J.: Estimates of late Cenozoic climate change relevant to Earth surface processes in tectonically active orogens, Earth Surf. Dynam., 6, 271–301, https://doi.org/10.5194/esurf-6-271-2018, 2018.
Oades, J. M. and Waters, A. G.: Aggregate hierarchy in soils, Soil Res., 29, 815–828, https://doi.org/10.1071/SR9910815, 1991.
Ochyra, R. and Matteri, C. M.: Bryophyta, Musci: Amblystegiaceae, in: Flora Criptogámica de Tierra del Fuego, edited by: Guarrera, S. A., Gamundi de Amos, I., and Matteri, C., Consejo Nacional de Investigaciones Científicas y Técnicas de al Republica Argentina, Buenos Aires, 14, 1–96, 2001.
Oeser, R. A., Stroncik, N., Moskwa, L.-M., Bernhard, N., Schaller, M., Canessa, R., van den Brink, L., Köster, M., Brucker, E., and Stock, S.: Chemistry and microbiology of the Critical Zone along a steep climate and vegetation gradient in the Chilean Coastal Cordillera, CATENA, 170, 183–203, https://doi.org/10.1016/j.catena.2018.06.002, 2018.
Oliver, M. J., Velten, J., and Mishler, B. D.: Desiccation Tolerance in Bryophytes: A Reflection of the Primitive Strategy for Plant Survival in Dehydrating Habitats?, Integr. Comp. Biol., 45, 788–799, https://doi.org/10.1093/icb/45.5.788, 2005.
Orchard, V. A. and Cook, F. J.: Relationship between soil respiration and soil moisture, Soil Biol. Biochem., 15, 447–453, https://doi.org/10.1016/0038-0717(83)90010-X, 1983.
Porada, P., Weber, B., Elbert, W., Pöschl, U., and Kleidon, A.: Estimating impacts of lichens and bryophytes on global biogeochemical cycles, Global Biogeochem. Cy., 28, 71–85, https://doi.org/10.1002/2013GB004705, 2014.
R Core Team: R: A language and environment for statistical computing, R Foundation for Statistical Computing, Vienna, Austria, 2 pp., 2018.
Rillig, M. C.: Arbuscular mycorrhizae, glomalin, and soil aggregation, Can. J. Soil Sci., 84, 355–363, https://doi.org/10.4141/s04-003, 2004.
Rosenbaum, P. R.: Sensitivity analysis in observational studies, in: Encyclopedia of statistics in behavioral science, edited by: Everitt, B. S. and Howell, D. C., https://doi.org/10.1002/0470013192.bsa606, 2005.
Rosentreter, R., Eldridge, D. J., Westberg, M., Williams, L., and Grube, M.: Structure, composition, and function of biocrust lichen communities, in: Biological soil crusts: An organizing principle in drylands, edited by: Weber, B., Büdel, B., and Belnap, J., Springer, 121–138, https://doi.org/10.1007/978-3-319-30214-0_7, 2016.
Rundel, P. W. and Weisser, P. J.: La Campana, a new national park in central Chile, Biol. Conserv., 8, 35–46, https://doi.org/10.1016/0006-3207(75)90077-4, 1975.
Samolov, E., Baumann, K., Büdel, B., Jung, P., Leinweber, P., Mikhailyuk, T., Karsten, U., and Glaser, K.: Biodiversity of Algae and Cyanobacteria in Biological Soil Crusts Collected Along a Climatic Gradient in Chile Using an Integrative Approach, Microorganisms, 8, 1047, https://doi.org/10.3390/microorganisms8071047, 2020.
Scholten, T. and Seitz, S.: Soil Erosion and Land Degradation, Soil Systems, 3, 68, https://doi.org/10.3390/soilsystems3040068, 2019.
Seitz, S., Goebes, P., Song, Z., Bruelheide, H., Härdtle, W., Kühn, P., Li, Y., and Scholten, T.: Tree species and functional traits but not species richness affect interrill erosion processes in young subtropical forests, SOIL, 2, 49–61, https://doi.org/10.5194/soil-2-49-2016, 2016.
Seitz, S., Nebel, M., Goebes, P., Käppeler, K., Schmidt, K., Shi, X., Song, Z., Webber, C. L., Weber, B., and Scholten, T.: Bryophyte-dominated biological soil crusts mitigate soil erosion in an early successional Chinese subtropical forest, Biogeosciences, 14, 5775–5788, https://doi.org/10.5194/bg-14-5775-2017, 2017.
Six, J., Paustian, K., Elliott, E. T., and Combrink, C.: Soil Structure and Organic Matter I. Distribution of Aggregate-Size Classes and Aggregate-Associated Carbon, Soil Sci. Soc. Am. J., 64, 681–689, https://doi.org/10.2136/sssaj2000.642681x, 2000.
Six, J., Bossuyt, H., Degryze, S., and Denef, K.: A history of research on the link between (micro)aggregates, soil biota, and soil organic matter dynamics, Soil Till. Res., 79, 7–31, https://doi.org/10.1016/j.still.2004.03.008, 2004.
Slessarev, E. W., Lin, Y., Bingham, N. L., Johnson, J. E., Dai, Y., Schimel, J. P., and Chadwick, O. A.: Water balance creates a threshold in soil pH at the global scale, Nature, 540, 567–569, https://doi.org/10.1038/nature20139, 2016.
Steven, B., Gallegos-Graves, L. V., Belnap, J., and Kuske, C. R.: Dryland soil microbial communities display spatial biogeographic patterns associated with soil depth and soil parent material, FEMS Microbiol. Ecol., 86, 101–113, https://doi.org/10.1111/1574-6941.12143, 2013.
Thielen, S., Gall, C., Ebner, M., Nebel, M., Scholten, T., and Seitz, S.: Water's path from moss to soil: A multi-methodological study on water absorption and evaporation of soil-moss combinations, J. Hydrol. Hydromech., 69, 421–435, https://doi.org/10.2478/johh-2021-0021, 2021.
Totsche, K. U., Amelung, W., Gerzabek, M. H., Guggenberger, G., Klumpp, E., Knief, C., Lehndorff, E., Mikutta, R., Peth, S., Prechtel, A., Ray, N., and Kögel-Knabner, I.: Microaggregates in soils, J. Plant Nutr. Soil Sc., 181, 104–136, https://doi.org/10.1002/jpln.201600451, 2018.
Van Bavel, C.: Mean weight-diameter of soil aggregates as a statistical index of aggregation, Soil Sci. Soc. Am. J., 14, 20–23, https://doi.org/10.2136/sssaj1950.036159950014000C0005x, 1950.
Wagner, S., Cattle, S. R., and Scholten, T.: Soil-aggregate formation as influenced by clay content and organic-matter amendment, J. Plant Nutr. Soil Sc., 170, 173–180, https://doi.org/10.1002/jpln.200521732, 2007.
Whitney, K. M., Vivoni, E. R., Duniway, M. C., Bradford, J. B., Reed, S. C., and Belnap, J.: Ecohydrological role of biological soil crusts across a gradient in levels of development, Ecohydrology, 10, e1875, https://doi.org/10.1002/eco.1875, 2017.
Wickham, H.: ggplot2: Elegant Graphics for Data Analysis, Use R!, Springer International Publishing, https://doi.org/10.1007/978-3-319-24277-4, 2016.
Zhang, Z.-S., Song, X.-L., Lu, X.-G., and Xue, Z.-S.: Ecological stoichiometry of carbon, nitrogen, and phosphorus in estuarine wetland soils: influences of vegetation coverage, plant communities, geomorphology, and seawalls, J. Soil. Sediment., 13, 1043–1051, https://doi.org/10.1007/s11368-013-0693-3, 2013.