the Creative Commons Attribution 4.0 License.
the Creative Commons Attribution 4.0 License.
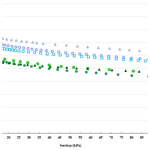
Effects of innovative long-term soil and crop management on topsoil properties of a Mediterranean soil based on detailed water retention curves
Alaitz Aldaz-Lusarreta
Rafael Giménez
Miguel A. Campo-Bescós
Luis M. Arregui
Iñigo Virto
The effectiveness of conservation agriculture (CA) and other soil management strategies implying a reduction of tillage has been shown to be site-dependent (crop, clime and soil), and thus any new soil and crop management should be rigorously evaluated before its implementation. Moreover, farmers are normally reluctant to abandon conventional practices if this means putting their production at risk. This study evaluates an innovative soil and crop management (including no-tillage, cover crops and organic amendments) as an alternative to conventional management for rainfed cereal cropping in a calcareous soil in a semi-arid Mediterranean climatic zone of Navarra (Spain), based on the analysis of soil water retention curves (SWRCs) and soil structure. The study was carried out in a small agricultural area in the municipality of Garínoain (Navarre, Spain) devoted to rainfed cereal cropping. No other agricultural area in the whole region of Navarre exists where soil and crop management as proposed herein is practiced. Climate is temperate Mediterranean, and the dominant soil is Fluventic Haploxerept. Within the study area there is a subarea devoted to the proposed soil and crop management (OPM treatment), while there is another subarea where the soil and crop management is conventional in the zone (CM treatment). OPM includes no-tillage (18 years continuous) after conventional tillage, crop rotation, use of cover crops and occasional application of organic amendments. CM involves continuous conventional tillage (chisel plow), mineral fertilization, no cover crops and a lower diversity of crops in the rotation. Undisturbed soil samples from the topsoil and disturbed samples from the tilled layer were collected for both systems. The undisturbed samples were used to obtain the detailed SWRCs in the low suction range using a HYPROP©device. From the SWRCs, different approaches found in the literature to evaluate soil physical quality were calculated. The pore-size distribution was also estimated from the SWRCs. Disturbed samples were used in the laboratory to assess soil structure by means of an aggregate-size fractionation and to perform complementary analysis from which other indicators related to soil functioning and agricultural sustainability were obtained. The approaches evaluated did not show clear differences between treatments. However, the differences in soil quality between the two forms of management were better observed in the pore size distributions and by the analysis of the size distribution and stability of soil aggregates. There was an overabundance of macropores under CM, while the amount of mesopores (available water) and micropores were similar in both treatments. Likewise, more stable macroaggregates were observed in OPM than in CM, as well as more organic C storage, greater microbial activity, and biomass. The proposed management system is providing good results regarding soil physical quality and contributing also to the enhancement of biodiversity, as well as to the improvement in water-use efficiency. Finally, our findings suggest that the adoption of the proposed practice would not result in a loss in yields compared to conventional management.
- Article
(917 KB) - Full-text XML
- BibTeX
- EndNote
Conservation agriculture (CA) and other soil management strategies implying a reduction of tillage have been reported to reduce soil degradation – preserving soil structure and associated porosity – in different agroecological situations (Verhulst et al., 2010; Sartori et al., 2022), and in many cases they are indeed designed for this purpose (Virto et al., 2015).
The reasons reported for CA's adoption in Europe are several. In northern Europe soil erosion control, soil crusting in loamy soils and the need to increase soil organic C storage, as well as soil trafficability, are widely cited as reasons for CA implementation (Lahmar et al., 2007). In the Mediterranean countries, soil water storage and water-use efficiency can be added to this list of reasons (De Tourdonnet et al., 2007). The most widely reported benefits of CA in southwestern Europe in relation to erosion are the increased soil infiltrability and/or the protective effect of crop residues on the soil surface (Gómez et al., 2009; Espejo-Pérez et al., 2013; Virto et al., 2015). In Spain, the soil water retention capacity has been observed to be greater in semi-arid land under no-tillage (Fernández-Ugalde et al., 2009; Bescansa et al., 2006). Other positive effects of CA on soil quality observed in semi-arid rainfed agricultural systems in Spain are related to soil organic C and nutrient storage (Ordóñez Fernández et al., 2007).
However, different studies show that the effectiveness of CA in solving these problems can be site-dependent (Costantini et al., 2020; Chenu et al., 2019) and variable depending on its effect on crop yields (Virto et al., 2012).
Indeed, since crop performance under no-till is strongly dependent on the crop type, climate (Or et al., 2021) and also soil type, no-till may not be suitable for all conditions (Pittelkow et al., 2015). In fact, in some areas, no-till often results in a reduction in crop yields of ca. 10 % (Or et al., 2021). Conventional tillage – in carefully managed agricultural soils – may be imposed when no-tillage would lead to chronic and unacceptable yield losses.
From the perspective of the effects of CA on the soil, among the existing approaches to assess soil condition (Minasny and McBratney, 2018), Rabot et al. (2018) highlighted the interest of soil structure as an indicator of its performance, as well as the relevance of considering the organization, distribution and stability of aggregates and the characterization of the associated pore system.
There are different types of techniques to characterize the soil pore system (Pires et al., 2013; Taina et al., 2013; Pagliai et al., 2004). The analysis of soil water retention curves (SWRCs) – the relationship between soil water matric potential and soil water content – is one of the most employed methods for characterizing soil pores. It enables an adequate characterization of the effective porous system (interconnected, functional pores), and therefore, SWRCs are a valuable tool to diagnose the physical condition of soils (Dexter, 2004a, b; Pires et al., 2017). In addition, it is a relatively fast and low-cost methodology.
One relevant issue in the assessment of the effects of soil management on soils is the increasing need to co-learn with farmers and other stakeholders (Bouma, 2014) and to identify the consequences of changes in land use in actual field conditions. Likewise, this assessment needs to account for as much soil functions as possible (Bünemann et al., 2018), as recently suggested from the perspective of linking soils with sustainable development goals (SDGs; Lal et al., 2021; Bouma et al., 2021).
In this framework, the objective of this study was to assess the continuous application, throughout 18 years, of an innovative soil and crop management – in comparison with conventional management – for the improvement of the soil physical condition, as well as the optimization of the soil water balance, in rainfed cereal agrosystems in semi-arid land (Navarre, Spain). It has to be emphasized that there is – to our knowledge – no other agricultural area in the whole region of Navarre where soil and crop management as proposed herein is practiced – and even less so for almost two decades – with the exception of precisely our test area.
Based on the analysis of detailed SWRCs and soil structure (i.e., the size distribution of stable macro- and microaggregates) and its consequences for soil water retention, the evaluation includes other complementary aspects relevant to soil functioning and SDGs by assessing soil organic C storage (climate regulation, SDG #13), the soil biological diversity (biodiversity loss, SDG #15) and (as far as available from farmers) yields (food security, SDG #2). This evaluation aims to incorporate therefore real-case field-measured indicators, in line with the recent recommendations of the new European Agricultural Policy (Bouma et al., 2022; Panagos et al., 2022).
2.1 Study zone and treatments
The study was carried out in a small agricultural area in the municipality of Garínoain (Navarre; 42.59843∘ N, 1.64959∘ E). This is an area with a Csb type of climate according to the Köppen–Geiger classification (Gobierno de Navarra Meteorología y Climatología de Navarra, 2022; Peel et al., 2007). The mean annual reference evapotranspiration according to the FAO Penman–Monteith method is 1107 mm yr−1. For crops in the rotation, the mean annual crop evapotranspiration is 326 mm yr−1. The soil – Fluventic Haploxerepts (Soil Survey Staff, 2014) and Fluvic Cambisol (IUSS Working Group WRB, 2015) – is devoted to rainfed cereal cropping. The physical-chemical properties of the soil (Table 1) showed high homogeneity of the material at the study depth (0–30 cm) regarding the most relevant physical-chemical properties related to moisture retention, except for the content of organic C (which can be related to the change in management). In addition, in situ standard soil description corroborated the homogeneity of the topsoil (0–30 cm).
Table 1Physical-chemical properties of the soil (0–30 cm) in OPM and CM treatments and the textural characterization of both treatments. Mean ± standard deviation of the mean (n=3). Statistically significant differences (p<0.05) are in bold.
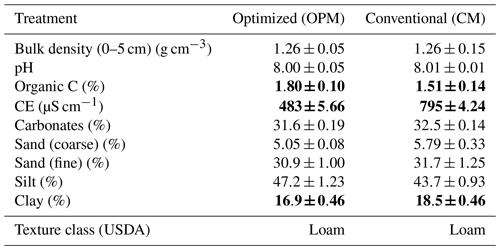
The physical-chemical properties of the soils shown in Table 1 were done using standard methods. In particular, soil pH was analyzed in a 1 : 2.5 soil : water solution as in Hendershot and Lalande (1993), organic C content by wet combustion as in Tiessen and Moir (1993), carbonates in a modified Bernard's calcimeter following Pansu and Gautheyrou (2003a), and the electrical conductivity in a soil : water solution similar to that for pH analysis (Pansu and Gautheyrou, 2003b). The soil texture was determined by the pipette method. All analyses were conducted on air-dried samples ground to 2 mm, collected at 0–30 cm, as proposed, for example, by FAO for organic C storage (FAO, 2020). Finally, the bulk density was determined using the HYPROP© device (see Sect. 2.2) from undisturbed samples extracted from the first 5 cm of the soil profile. However, based on the field standard soil description, it is fairly safe to assume that the bulk density is roughly constant up to 30 cm depth.
Within the study area there is a subarea – to our knowledge, unique in Navarre – devoted to a pioneer optimized soil and crop management (from now on OPM treatment). There is another subarea – adjacent to the OPM one – where the soil and crop management is conventional in the zone (from now on CM treatment).
OPM is an optimized system, used for 18 consecutive years, which includes direct seeding, an improved crop rotation including wheat (Triticum aestivum L.), barley (Hordeum vulgare L.), legumes (Pisum sativum L., Vicia faba L. and others) and rapeseed (Brassica napus L.), and the occasional use of cover crops and organic amendments. Both grain and straw were removed in the first 11 years of implementation, and only stubble remained on the surface of soil when direct seeding was implemented with minimal soil perturbation. Since then, and for the 7 remaining years, the procedure was slightly modified, and only grain was removed at harvest. Therefore, chopped straw and stubble remained on the surface of the soil before direct seeding with no disruption of the soil surface. At the same time, cover crops were introduced in the system, despite this being a risky practice in rainfed Mediterranean agrosystems characterized by warm and dry summers. As such, summer cover was routinely granted in this system by letting spontaneous vegetation grow in the summer after harvest. This vegetation was controlled with herbicides before seeding the cash crops in the fall. Also, for only one year, the winter crop used was Vicia villosa Roth, and it served as a cover crop for sorghum (Sorghum vulgare L.), which was successfully grown in the spring and fall seasons despite the limiting water availability in the area.
CM is a conventional management, which employs conventional continuous (annual) tillage with a chisel plow down to 15 cm, mineral fertilization without cover crops and a less diverse crop rotation including mostly wheat and occasionally legumes and rapeseed. Crop residues are not returned into the soil (both grain and straw were removed annually): only the non-exported stubble and roots were therefore incorporated into the soil at 10–15 cm depth by vertical tillage.
In both treatments (OPM and CM), mineral fertilization consisted of phosphorus addition before seeding (120–150 kg ha−1 of triple superphosphate 0–46–0) and nitrogen supply of 180 kg N ha−1 (split and distributed into two cover dressings at 60 and 120 kg N ha−1 in January and March, respectively) as urea. Organic fertilization was not used in any of the study treatments until 2021, in which an organic amendment was applied to the soil without disturbing the surface in the OPM treatment. After harvest, pig slurry was applied with an average concentration of 2.5 kg N m−3, by means of a tanker equipped with a system of hanging pipes that deposit the product a few centimeters above the ground and at a time close to a forecasted rainfall event. The application rate was 60 m3 ha−1 of slurry. These rates are within the legal limits established by legislation for groundwater protection against pollution caused by nitrates from agricultural sources (EU Directive 91/676, Council of the European Union, 2008), as the area is within a vulnerable watershed according to this directive.
To avoid the possible influence of the preceding crop, it was ensured that the two last crops of the rotation before the study both in OPM and CM were the same (winter wheat, Triticum aestivum L., and rapeseed, Brassica napus L.).
2.2 Soil sampling and methodological approach
Soil sampling for both treatments (OPM and CM) was carried out in early fall – after harvest and before soil preparation for seeding in CM, approximately 4 months after the last tillage for CM – at three (n=3) randomly selected sampling sites per treatment: undisturbed cylindrical (8 cm diameter, 5 cm height) samples were collected from the first 5 cm of each sampling site.
In addition, in the same points, three disturbed composite samples – comprising three subsamples each – were taken at 0–30 cm depth for further physical-chemical and biological analysis in the lab. Immediately after sampling, part of the composite soil was stored at 5 ∘C for biological analysis, while the remainder was used to assess soil aggregation, as detailed below.
Determination of SWRCs. From the undisturbed cylindrical samples, SWRC tracks were obtained in the laboratory with a HYPROP© device commercialized by METER (Munich, Germany) as described by Schindler et al. (2010). This device uses the Peters and Durner (2008) and Schindler (1980) simplified evaporation method. The procedure is based on the continuous measuring of the matric component of soil water potential from two micro-tensiometers inserted into the saturated soil sample, while the moisture content of the sample is progressively reduced by evaporation. As the experiment advances, the sample loses water by evaporation, and the tensiometers record the variation of suction as a scale measures the weight change. The registries of suction and weight are automated and continuous. Gravimetric water content can be expressed as volumetric content since bulk density is known (Schindler et al., 2010).
After the evaporation experiment concluded, the samples were dried in an oven at 105 ∘C for 24 h to determine the dry weight and the soil bulk density for the subsequent evaluation of the results using the HYPROP-FIT (version: 4.2.2.0) software (Pertassek et al., 2015). In total, around 100 evenly distributed suction water content values between 0 and 150 kPa were measured, with an extra measurement at 1500 kPa (classical wilting point) obtained using a pressure plate (Dirksen, 1999). The classical concept of a permanent wilting point at a suction of 1500 kPa facilitates comparisons since it is widely used in the literature, though it should be taken with caution since it is not a universal wilting limit. Wiecheteck et al.'s (2020) findings when comparing the classical permanent wilting limit with the biological wilting of wheat and barley suggest that wilting depends on soil texture, with an occurrence of wilting at lower suction (i.e., wetter soil conditions) for sandy soils than for clay soils.
2.3 Analysis of the SWRCs and derived indices and functions
First, it should be noted that different mathematical functions to adjust SWRCs are found in the literature depending on the general shape of the SWRC. The SWRC of most soils presents a J form, defined by the presence of the air-entry region, in which the volumetric water content is maintained at saturation values even in suctions slightly over zero; this occurs due to occluded pores (not functional) (Kosugi et al., 2002). Instead, when there is no marked air-entry region, the SWRC adopts an S form. For instance, in the case of fine-textured undisturbed soils, the SWRC usually presents the shape of an S (Kosugi et al., 2002). Following Brooks and Corey (1964), in J-shaped SWRCs the best fit occurs with an exponential function. But, for S-shaped SWRCs, the fit with exponential functions is poor (Milly, 1987; van Genuchten and Nielsen, 1985), and it is recommended to employ sigmoidal-type functions such as the van Genuchten equation (van Genuchten, 1980).
Predicting soil water retention by uni-modal approaches: S index. Dexter (2004a) proposed an S index to estimate the physical condition of soils (changes in soil structure and therefore in porosity) based on the soil SWRC. This index represents the value of the slope of the SWRC at the inflection point when the curve is expressed as the natural logarithm of suction (in hPa) versus the gravimetric moisture content, θg (kg kg−1) (Dexter, 2004a, b). According to Dexter (2004a), this inflection point defines the limit between structural pores (in the range of low suction values) and textural pores (in the range of high suction values). It is assumed that, as S increases, structural pores are more abundant, and, therefore, there are better conditions for water flow and storage in the soil (Dexter, 2004a).
The inflection point can be determined directly by hand from the SWRC if there are enough accurate measurement points (Dexter, 2004a). Alternatively, it would be more appropriate to fit the SWRC to a mathematical function and then to calculate the slope at the inflection point in terms of the parameters of the function. To do this, one of the best-known functions is that proposed by van Genuchten (1980), for which, in turn, pedo-transfer functions are available for the estimation of its parameters (Dexter, 2004a).
The value of S was calculated in two different ways assuming a uni-modal pore size distribution: (i) from a sigmoidal function fitted to experimental data (Eq. 1) and (ii) from the adjusted parameters of the van Genuchten (1980) function (Dexter, 2004a) (Eq. 2). To this end, the whole dataset was used, i.e., 0–150 and 1500 kPa.
where y is the logarithm of suction (hPa), x is the gravimetric moisture (kg kg−1), and a, b and x0 are parameters of the equation.
where h is the soil matric potential (hPa), θh (m3 m−3) is the measured soil water content at matric potential h, θres is the residual water content (m3 m−3), θsat is the saturated water content (m3 m−3), and α (hPa−1), n (–) and (–) are the van Genuchten parameters.
Predicting soil water retention by a bi-modal approach. Likewise, the water retention data were fitted to the double-exponential equation with five adjustable terms proposed by Dexter et al. (2008), in which all the parameters have a different physical meaning (Eq. 3). To this end, the dataset between 0–150 kPa was used.
According to Jensen et al. (2019), this model can reflect better the effects of management systems in the soil properties.
where θ is the gravimetric water content, C is the residual water content (asymptote of the equation), and the amount of matrix and structural pore space are proportional to A1 and A2, respectively. The values of h1 and h2 are the characteristic pore water suctions at which the matrix and structural pore spaces empty, respectively (Dexter et al., 2008).
Numerical integration of SWRCs: water retention energy index. The water retention energy index (WRa) (Armindo and Wendroth, 2016) (Eq. 4) obtained from numerical integration including each SWRC was determined.
where θfc and θpwp are the volumetric water content at field capacity and permanent wilting point, respectively, and h is suction (kPa).
WRa quantifies the total absolute energy that has to be applied by the soil to hold water in its pores between field capacity (θfc) – i.e., after the water drainage process becomes negligible – and wilting point (θpwp) or any moisture point θj, where . The WRa index was determined for the suction range between field capacity (ca. 10 kPa, see below) and a moisture content corresponding to ca. 150 kPa (maximum operating value of the HYPROP© device), which means a dataset of around 100 measured points (see above). It is clear that the accuracy of this index is highly conditioned by the degree of detail of the SWRCs.
This index presents an adequate sensitivity for smaller-scale, high-precision applications and for capturing the dynamic evolution of the soil physical state (Armindo and Wendroth, 2016). More precisely, in the case of two SWRCs measured before and after some natural or anthropogenic changes (e.g., tillage), these energy indices can be used to quantify the change in soil physical quality status (Armindo and Wendroth, 2016).
Estimation of field capacity. The HYPROP© device, besides determining the SWRC, provides values for soil unsaturated hydraulic conductivity at different water contents. From this, it is possible to estimate the moisture content of the soil at field capacity – i.e., once gravitational water is drained.
Estimation of pore size distribution. The soil pore size distribution was estimated from the equivalent radius obtained from the suction values of SWRCs, using the equation formulated by Young and Laplace (Warrick, 2003) (Eq. 5):
where h is the height of the liquid (m), T is the surface tension (N m−1), θ is the contact angle of the liquid, ρ is the density of the liquid (kg m−3), g is the gravitational acceleration (m s−2), and r is the equivalent radius of the pores (m) retaining water at a suction equivalent to h (m).
2.4 Indicators of soil structure
Aggregate size fractionation. Firstly, field-moist soil samples were gently passed through a 5 mm sieve, without forcing the aggregates, and left to dry naturally. Then 50 g were collected from each soil sample and subjected to humidification with deionized water vapor at room temperature until saturation.
Water-stable aggregate fractionation followed the step-wise protocol described by Oliveira et al. (2019) as follows. Firstly, each moist soil sample was sequentially sieved (250 and 50 µm) to obtain three aggregate fraction sizes (Elliott, 1986): macroaggregates (Maggs; >250 µm), microaggregates (maggs; 50–250 µm), and the silt and clay fraction ((s+c); <50 µm). To this end, initially, 50 g of saturated soil sample was spread over a 250 µm sieve. The soil was then submerged in deionized water for approximately 30 s and then manually sieved by moving the sieve upwards and downwards 15 times for a distance of 1.5 cm during 30 s. The sieved material was then placed on a 50 µm sieve and submerged again for 30 s in deionized water, and the manual sifting was repeated. The sieved material was then transferred to a 500 mL centrifuge bottle and centrifuged at × 13 000 g for 10 min to recover the silt and clay fraction. The aggregates retained by the sieves (>250 and 50–250 µm) were gathered and dried in an oven at 50 ∘C along with the fraction <50 µm and stored at ambient temperature for subsequent analysis.
The second step consisted in the fractionation of the > 250 µm fraction (Magg) into three new fractions: coarse particulate organic matter >250 µm (cPOM + sand), microaggregates within macroaggregates (mMaggs; 50–250 µm) and particles <50 µm within macroaggregates (M(s+c)). To this end, an ad hoc device adapted from Six et al. (2002), which consists of a block formed by a 250 µm sieve located above a 50 µm sieve, was employed. This block was placed on an agitator, and 10 g of Magg (>250 µm) and 50 glass beads (4 mm in diameter) were poured on the 250 µm sieve. The block was horizontally agitated for approximately 2 min at 125 rpm while deionized water was poured until Magg disaggregated completely. The material retained in the 250 µm and 50 µm sieves corresponded to the fractions of >250 µm (cPOM + sand) and mMagg (50–250 µm), respectively. Similar to the first step, the M(s+c) fraction was recovered by centrifugation. The three fractions were dried at 50 ∘C and stored at ambient temperature.
2.5 Other soil indicators
As a complement of the detailed study of water retention, soil porosity and structure, other indicators related to soil functioning and agricultural sustainability were analyzed. First, the distribution of organic C among aggregate fractions was determined by analyzing the organic C concentration in every fraction by wet oxidation following (Tiessen and Moir, 1993). Second, microbial biomass C (MBC) was measured by fumigation extraction as described by Vance et al. (1987), and the functional diversity of the soil microbial populations was carried out following Preston-Mafham et al. (2002) from fresh samples and by a study of the utilization patterns of different C sources with EcoPlates™ (Biolog, Hayward, CA, USA). The average well color development (AWCD) and the number of substrates used by the microbial community within the soil (NSU) were determined from the EcoPlates™ as quantitative indicators of the soil functional diversity based on community-level physiological profiles (Zak et al., 1994).
2.6 Statistical analysis
Three (n=3) replicates of each study treatment (OPM and CM) were used in the statistical analysis. A one-factor analysis of variance (ANOVA) with significance level p<0.05 was performed for the different indicators to examine the significant influence of OPM. All statistical analyses were performed using IBM SPSS Statistics 27.0 (IBM Corp, 2021).
3.1 Analysis of the SWRCs
A clear difference between the SWRCs of the two treatments was observed: the variability between treatments was remarkably superior to the one existing between the replicates of the same treatment (Fig. 1).
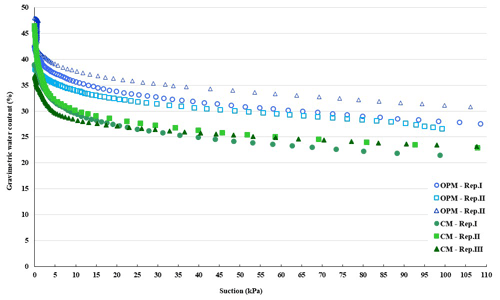
Figure 1Soil water retention curves for each replicate (n=3) for the two treatments: optimized management (OPM) vs. conventional management (CM).
The saturation water content in both treatments was similar (p>0.05), which indicates that there was no significant compaction (and therefore, reduction of the total porous space) because of management for the studied depth. This is consistent with the observation of soil in both treatments presenting the same bulk density (Table 1).
In relation to the shape of the SWRCs, both corresponded to the S-shaped type (Kosugi et al., 2002): a relevant presence of occluded or non-functional pores was not observed (the air-entry region was negligible; Fig. 1).
Nonetheless, the specific water capacity – change in the moisture content per unit of suction, , as defined by Klute (1952) – in the suction range between saturation (0 kPa) and near field capacity (10.5 ± 0.56 kPa) was significantly higher for CM () than for OPM (). However, when suction was greater than 10 kPa, the value of specific water capacity tended to be similar for both treatments, with no significant differences (p>0.05) above 32 kPa () (Fig. 1).
3.2 S index
The S index obtained from both the van Genuchten equation (Table 2) and the ad hoc sigmoidal equation (Table 3) showed no significant differences (p>0.05) between both treatments. However, it should be noted that the S values obtained from the van Genuchten equation showed a better performance, with a dispersion 1 order of magnitude smaller than that obtained from the ad hoc sigmoidal equation.
The S value for the two study treatments reflected good soil physical quality () for the van Genuchten equation (Table 2) and very good (≥0.050) for the sigmoidal equation (Table 3) (Bacher et al., 2019; Dexter, 2004b; Reynolds et al., 2009).
Table 2S-index values, contents of water (θ) and suction (Ψ) corresponding to the inflection point, obtained with the van Genuchten equation and van Genuchten parameters. Mean ± standard deviation of the mean (n=3). All the differences are not statistically significant (p>0.05).

3.3 Bi-modal approach
Experimental results were plotted as differential functions ( vs. log h(hPa)) to look for a multimodal behavior: all the curves analyzed seemed to be of the uni-modal type (data not shown). However, it should be noted that suction values did not exceed 150 kPa, and according to Dexter et al.'s (2008) (cf. their Fig. 3) and Jensen et al.'s (2019) (cf. their Fig. 2) findings, the second peak defining a bimodal behavior seems to appear at suction around 1000 kPa. Then, we tried again incorporating the water content-suction measurements at 1500 kPa to the dataset with the same result, i.e., uni-modal behavior. But this could be an artifact of the dataset since there is a wide experimental gap between 150 and 1500 kPa, i.e., no measurements in between.
Despite this, the double-exponential equation for soil water retention proposed by Dexter et al. (2008) was explored (Eq. 3) (Table 4). The structural pore space would have been reduced by 35 % as a result of no-tillage (OPM) (cf. A2 values, Table 4), while the matrix pore space values remain rather constant in both treatments (cf. A1 values in Table 4).
3.4 WRa index
The soil under OPM (WRa = 4.6±0.5; average ± standard deviation) seemed to have a better structure than the soils under CM (WRa = 4.1 ± 1.1) because the former held the same relative fraction of water with more absolute energy in its porous system (Armindo and Wendroth, 2016). However, this difference between treatments was not statistically significant due to the large variability observed in the CM treatment.
3.5 Analysis of the pore size distribution
Figure 2 depicts the probability distribution function of pore size (mean of the three replicates) for the study soil under OPM and CM, as well as the classification of pore sizes according to the Soil Science Society of America (Weil and Brady, 2017).
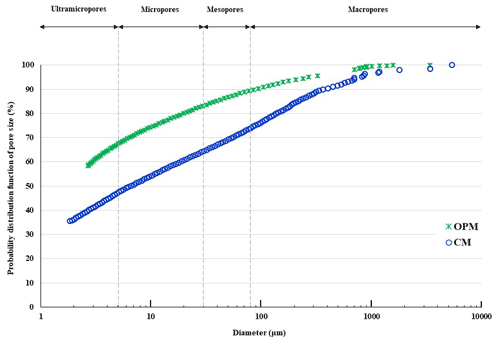
Figure 2Probability distribution function of pore size (mean of three replicates) of the soil under the two studied treatments (OPM and CM) and pore size classification (Weil and Brady, 2017). Note: x axis in logarithmic scale.
For both treatments, the percentage of mesopores (equivalent diameter between 30 and 80 µm) was similar (5.6 ± 0.7 in OPM and 8.0 ± 1.3 in CM) (p>0.05). Similarly, the population of smaller pores (micropores, with equivalent diameter between 5 and 30 µm) did not present significant differences for both treatments (15.5 ± 1.1 % in OPM and 16.4 ± 2.3 % in CM; Fig. 2) (p>0.05), which confirmed the textural homogeneity of the soil in both treatments (Table 1), as this porosity is more associated with soil texture than the soil structure (Pagliai et al., 2004).
In contrast, the proportion of pores with equivalent diameters >80 µm (macropores) differed between treatments (p<0.05). For CM, macropores represented 27.7 ± 4.8 % of total porosity and only 11.6 ± 2.3 % for OPM. As such, in CM, the population of pores with equivalent diameter 500–1000 and >1000 µm represented 5.5 ± 1.3 % and 4.4 ± 2.2 %, respectively. For OPM, the population of pores larger than 500 µm – considered mainly as fissures (Pagliai et al., 2004) – was 2.8 ± 1.3 %, with no apparent presence of pores larger than 1000 µm (<1.3 ± 0.7 %). As macropores drain quickly at low suctions, when these macropores become empty, the volume of soil capable of storing available water is then reduced. Therefore, up to 100 kPa, the soil under OPM could eventually store a higher amount of water (ca. 10 %–15 %) per unit of volume than under CM (Fig. 1).
3.6 Analysis of the size distribution of stable aggregates
Mass losses during fractionation accounted for 3.6 ± 0.2 % of the initial samples, with no differences between treatments (data not shown), which means that the differences found (Fig. 3) can be considered as a response to the studied treatments.
For both treatments, the percentage of soil within water-stable macroaggregates (Maggs) and microaggregates (maggs) was 92.2 ± 0.3 %, and the non-aggregated (s+c) fraction presented 5.8 ± 0.4 % of the initial mass (Fig. 3). Within the aggregated fractions (Magg + magg), clear differences were observed in the size distribution of aggregates (p<0.05): the soil under OPM had 75.9 ± 2.6 % of stable macroaggregates (Magg, >250 µm), while this percentage was 57.5 ± 2.1 % for CM.
In relation to the composition of Magg, both cPOM and mMagg represented a greater proportion of Magg in OPM in comparison to CM (where M(s+c) represented a greater proportion of total Magg mass) (Fig. 3). It has to be noted that both cPOM and mMagg included an undetermined percentage of sand particles. However, the similar texture of the soil for both treatments (Table 1) allows us to consider that the observed differences cannot be attributed to differences in the sand content.
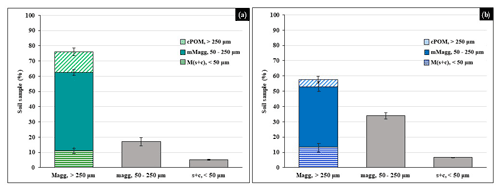
Figure 3Size distribution of stable aggregates and individual particles in the soil under OPM (a) and CM (b). Magg: Macroaggregates; magg: microaggregates; mMagg: microaggregates within macroaggregates; s+c: silt + clay fraction; cPOM: coarse particulate organic matter >250 µm and sand particles. The error bars represent the standard error, which is the standard deviation divided by the square root of the sample size. All aggregate fractions are significantly different (p<0.05) between OPM and CM, with the exception of mMagg.
3.7 Organic C storage and soil microbial diversity
The distribution of soil organic C (SOC) among aggregate fractions is shown in Fig. 4. It is worth mentioning that, for the two management systems, the carbon recovery data after fractionation were satisfactory, since no more than 10 % of the initial soil C was lost during the fractionation procedure (data not shown).
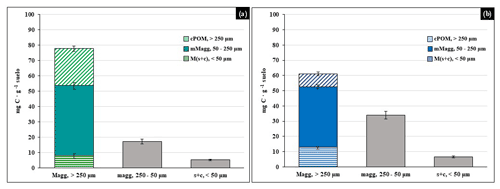
Figure 4Distribution of organic C in stable aggregates and individual particles in soil under OPM (a) and CM (b). Magg: Macroaggregates; magg: microaggregates; mMagg: microaggregates within macroaggregates; s+c: silt + clay fraction; cPOM: coarse particulate organic matter >250 µm and sand particles. The error bars represent the standard error, which is the standard deviation divided by the square root of the sample size. All aggregate fractions are significantly different (p<0.05) between OPM and CM, with the exception of s+c and mMagg.
In our study, soil management resulted not only in higher SOC concentration under OPM (Table 1) but also in a different distribution of SOC among aggregate size fractions. As such, OPM resulted in a higher proportion of SOC stored in Magg (77.7 ± 2.9 g C 100 g−1 soil C) than CM (61.1 ± 2.2 g C 100 g−1 soil C). Conversely, CM contained proportionally more SOC in magg and s+c <50 µm fractions. The greater proportion of SOC accumulated in Magg corresponded to that found in cPOM > 250 µm (30.2 ± 2.2 g C 100 g−1 Magg-C in OPM for 11.1 ± 1.4 g C 100 g−1 Magg-C in CM).
In relation to the soil microbiological indicators, OPM did not only result in more MBC but also in a higher efficiency for the degradation of organic substrates (degrading 29.17 % more substrates than in the conventional system, NSU; Table 5). Likewise, a more intense degradation of the substrates (> AWCD) was observed under OPM than CM.
Table 5Biological indicators. Mean ± standard deviation of the mean (n=3). Statistically significant differences (p<0.05) are in bold.
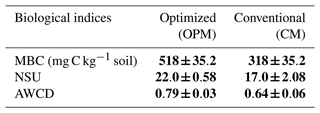
Finally, since this work was conducted in farmers' plots, yields were not explicitly measured, as is usually done in experimental fields, but some basic data are available from the farmers managing the fields (see Table 6 below). From these data, no apparent differences between treatments in crop yields occurred in the study area.
Dexter and Bird (2001) stated that one of the applications of the S index was to identify the optimal water content for tillage, which would correspond to the inflection point of the SWRC. This is in agreement with our results: moisture contents corresponding to S values were all near field capacity (Tables 2 and 3), the water content at which tillage produces the greatest proportion of small clods, which can be considered an achieved tillage.
Despite this observation, the S index was not sensitive enough to reflect differences in the soil physical quality due to the different soil and crop managements assessed. This, despite the high degree of detail of the SWRCs used, facilitates an optimal adjustment of the different mathematical functions applied. Alonso et al. (2022) also found no significant differences in S-index values between silt loam and sandy loam soils subjected to moldboard plowing, deep loosening and minimum tillage managements, while other soil physical quality variables did show significant differences between those soils. The S index is probably aimed at comparing soils in more contrasting conditions, especially in terms of bulk density, texture and organic matter content, as inferred from the case studies presented by Dexter (2004a).
The differences in soil quality between the two forms of management (OPM vs. CM) were better observed from the pore size distribution – obtained from the SWRCs – and by the analysis of size distribution and stability of soil aggregates. To this respect, our results showed an overabundance of macropores (>80 µm) under CM, while the amount of mesopores (available water) and micropores were similar in both treatments (Fig. 2). In other works in which SWRCs were used for the long-term study of pore size distribution in no-tillage (NT) and conventional tillage (CT) management, it was found that there is no unanimity in the results obtained (Wardak et al., 2022). Pires et al. (2017) evaluated the effect of tillage and direct seeding on the structure of an Oxisol through the analysis of SWRCs and micromorphological assessments. From their results, it can be observed (see Figs. 1 and 2; Pires et al., 2017) that the soil under conventional tillage reduced its water content (starting from saturation) by 15 % when a suction of approximately 20 cm was applied. For the soil under direct seeding, the decrease was only of 5 %. For the depth range 10–30 cm, the changes in moisture content with suction were similar for both treatments. In addition, in the soil under direct seeding, pores within the size range 50–500 µm – responsible for draining excess water (Greenland and Pereira, 1977) – occupied 39 % of the total porous space, while for tilled soil the percentage was slightly over 60 %. Lipiec et al. (2006) observed that the pore system of a silty clay loam soil under CT presented greater macroporosity, with the differences between tillage treatments being more pronounced in the 0–10 cm depth than in the 10–20 cm depth. Similar results were obtained in clayey soils by Tuzzin de Moraes et al. (2016) and Borges et al. (2019), who identified significantly higher macroporosity in CT treatment compared to NT. In contrast, Imhoff et al. (2010) and Gao et al. (2019) observed increased macroporosity in the NT treatment in a silty loam soil and a sandy loam soil, respectively.
In addition, in the study of micro- and mesopores, most studies have observed an increase in these pores under NT compared to CT. Examples are the works of Borges et al. (2019), Lipiec et al. (2006), and Tuzzin de Moraes et al. (2016), whose analysis of SWRCs showed a higher volume of micropores and mesopores under NT than under CT. However, in the study by Imhoff et al. (2010) a decrease in micro- and mesopore volume under NT was recorded. Similarly, Gao et al. (2019) saw reduced mesoporosity in NT soil, observing no significant effect of such a reduction on the soil hydraulic properties. It has to be noted that these pores are relevant for soil functioning, as mesopores are associated with water retention after free drainage, with a suction that enables easy extraction by plants (available water), transmitting water by capillarity to the radicular zone (Weil and Brady, 2017).
In any event, it seems from this variability of results that the impact on soil management on soil porosity is site-dependent. In agronomic and climatic conditions closer to the soil studied here, Pagliai et al. (1984) studied the size distribution and shape of pores in a clay loamy vertic soil under CT and NT, using micromorphological image analysis of soil thin sections. The size distribution of pores was more regular in the soil under direct seeding than under conventional tillage. For direct seeding, 7 % of the total pores identified (= 145) were macropores (500–1000 µm), occupying 25 % of the porous space (image area). For conventional tillage, in turn, the bias was considerable: 22 % of total pores (= 45) corresponded to macropores (500–1000 µm and >1000 µm), occupying approximately 85 % of the porous space (Fig. 1; Pagliai et al., 1984). This greater macroporosity in OPM can explain the fast desorption rate at low suction values (high specific water capacity) observed in CM compared with OPM (Fig. 1).
The overabundance of macropores in soils under CM in our study could be to some extent explained by an increase in soil fragments rather than soil aggregates in the CM in comparison with OPM treatment. Soil aggregates and fragments may look similar but are formed by different processes and have different properties (Or et al., 2021): soil fragments form by mechanical forces of tillage, and they tend to be mechanically weak and coalesce upon wetting with macroporosity collapsing within a single season. Instead, soil aggregation is stimulated by biological activity with biopolymers and hyphae that stabilize and bind soil particles. In short, soil aggregates are more stable than soil fragments. Borges et al. (2019) observed significantly higher macroporosity in a soil under conventional tillage compared to a soil under minimum tillage; they explained this by the mechanical action of tillage. The non-bimodal behavior of our SWRCs did not allow us to verify this extent from the A2 values (Dexter et al., 2008), theoretically corresponding to the structural pore space (Table 4).
In relation to aggregation, Fuentes-Guevara et al. (2022) found a significant correlation between hydraulic-energy-based indices – including WRa – and some physical properties before and after land-leveling operations, indicating their capacity to capture soil structure changes. The high variability observed for this index in CM (Sect. 3.4) hindered, however, their use for such an assessment in our case. However, the preponderance of Magg under OPM (Fig. 3) can be understood as a consequence of better soil condition (or lower degradation) than under CM in terms of aggregates stability. As conceptualized in the hierarchical model of soil aggregation (Angers et al., 1997; Beare et al., 1994; Golchin et al., 1994; Oades, 1984; Six et al., 1999, 2004; Tisdall and Oades, 1982), while maggs are formed within Maggs and stabilized mostly by the action of persistent agents (e.g., cationic complexes, humidified organic matter), Maggs are stabilized by the action of transitory agglutinating agents (hyphae and mycorrhizae, microbial and vegetable derivatives). The main implication of this hierarchy is that agricultural management primarily affects the less stable macroaggregates, while the more stable microaggregates are less influenced. Implicit in this concept is the fact that aggregates form sequentially (Jarvis, 2012). According to this hierarchical vision of soil aggregation, these agglutinating agents are, in turn, widely conditioned by soil management: the formation of (macro-)aggregates is thus favored by the lower degree of soil disturbance by tillage, higher inputs of crop (organic) residues in the soil organic matter pool and the punctual organic amendments used in OPM (Jastrow, 1996; Lehmann and Kleber, 2015; Six et al., 2004; Tisdall and Oades, 1982). This observation is supported by the higher proportion of cPOM (Fig. 3) and total organic C (Table 1) found under OPM, as explained below.
Although the relationship between organic matter cycling and soil structural stabilization has been observed to be soil-dependent (Rasmussen et al., 2018) and the calcareous nature of the studied soil may interact with it by stabilizing Magg and magg to a greater extent than in Ca-free soils (Fernández-Ugalde et al., 2011; Rowley et al., 2018, 2021), the greater accumulation of SOC within stable Magg in OPM than CM (Fig. 4) suggests that the response of soil structure to the reduction of tillage and the increase in organic C inputs corresponded to that observed previously in other soil types (Six et al., 2004; Fernández-Ugalde et al., 2016) and in soils of the same type in the region (Virto et al., 2007; Yagüe et al., 2016).
In relation to our objectives, these results indicate that the changes observed in the physical soil indicators studied above can be related to a more positive SOC balance, very likely related to more inputs from vegetation cover and fewer interruptions of the SOC cycling due to tillage. Soil C storage is generally observed as a key soil property, related to both soil functioning and the global C cycle. As such, it has been proposed as an indicator for several soil functions, including nutrient recycling, functioning of soil ecosystems, pollution control, food security and global change (Paul, 2016).
In addition, the accumulation of cPOM, which has been repeatedly identified as a fast cycling pool and a precocious indicator of changes in SOC cycling (Cotrufo et al., 2019), can be understood as the result of SOC cycling being more active and resulting in a greater proportional accumulation of labile forms of SOC under OPM than CM (Lehmann and Kleber, 2015).
The idea of a more active SOC cycle under OPM was supported by the observed higher microbial activity and microbial biomass C under OPM compared to CM (Table 5), which can be associated with better conditions for SOC degradation and stabilization under OPM (Six et al., 2002).
Other relevant consequences of the observed results in the topsoil of the studied sites can be those related to the control of soil losses through erosion. This depends, among other factors such as ground cover (granted by OPM), on the soil's own resistance to slaking and aggregate breakdown, as well as on the infiltration rates. The greater resistance of aggregates was clearly observed in OPM in our study, suggesting reduced erodibility. This supports the view of the use of cover crops in sensitive areas (Panagos et al., 2021) as a useful tool for the involvement of farmers in the reduction of erosion rates (Panagos et al., 2021; Mosavi et al., 2020; Grillakis et al., 2020; Eekhout and De Vente, 2020; Paroissien et al., 2015). In addition, although the assessment of water infiltration and hydraulic conductivity of the soil in field conditions are beyond the scope of this work and without other considerations such as the possible existence of compacted layers at depth caused by tillage (Fernández-Ugalde et al., 2009), this suggests a faster infiltration of water under this treatment. Considering the vulnerable character of this area with respect to groundwater pollution by nitrates, this would indicate a worse condition of soils in the area under conventional practices in terms of reaching the environmental goals in relation to fresh water quality set by the EU (Fetting, 2020), the UN (Lal et al., 2018), and other national and regional environmental policies.
Finally, it has to be noted that agricultural sustainability cannot forget the interest of farmers. Although yield data were available only from indirect sources (Table 6), they suggest that the implementation of OPM did not imply a relevant reduction in yields in the study area, as is often observed when reduced input strategies are introduced in some agrosystems. For instance, Or et al. (2021) have reported an average reduction of 10 % yields upon NT adoption.
In summary, and from a general point of view of the sustainability of agricultural management and the multifunctionality of soils (Bouma et al., 2019), these results indicate that OPM did not only result in differences in water retention and soil structure that can contribute to improve water-use efficiency and crop productivity but also in enhanced biodiversity and increased SOC storage. OPM seems from this perspective to be a useful tool in the face of the present challenges and commitments of agriculture in Europe and worldwide (Bouma et al., 2022; Panagos et al., 2022).
A pioneering and regionally unique (Navarre, Spain) optimized soil and crop management system that includes, among other techniques, reduced tillage, crop rotations and the occasional application of organic amendments was assessed for the soil physical quality after 18 years of its implementation. Our findings suggest, first, that some classical approaches to the assessment of SWRCs cannot capture the actual consequences of the use of these optimized management strategies on soil quality. However, detailed SWRCs were seen to be useful in identifying relevant changes in soil porosity.
In relation to the physical quality of the soil, the innovative management tested here provided good results after 18 years – highlighting the proportion and size of water-stable soil macroaggregates. It also contributed to a more abundant and diverse soil microbial population and could contribute to an improvement in water-use efficiency. This is especially relevant for rainfed agriculture where water is the most limiting factor for crop growth, such as in the study zone.
The optimized management analyzed herein can therefore be recommended for higher soil sustainability in Mediterranean agrosystems. However, it is not currently a widespread practice in the region most likely because the high initial investment and the farmer's concern that crop yields would be reduced. This work illustrates the need for an adequate assessment and dissemination to overcome these hesitations of farmers and other potential barriers to the adoption of this type of system.
Further analysis at deeper soil layers – at least to the rooting depth – are necessary for a more complete assessment of the proposed optimized management. Moreover, to better understand changes in the soil hydrology, it is necessary to carry out experiments to determine infiltration rates, preferably under controlled suction. Finally, future studies should take a dynamic approach to soil water regimes by taking advantage of the widely available dynamic simulation models of the soil–water–atmosphere–plant system.
The data that support the findings of this study are available from the corresponding author upon reasonable request.
RG and IV conceptualized and supervised the paper. LMA designed the experiments, and AAL carried them out. RG, MACB and AAL visualized the project, did the formal analysis and conducted the investigation with IV, who also collected the resources. AAL prepared the manuscript with contributions from all co-authors.
The contact author has declared that none of the authors has any competing interests.
Publisher's note: Copernicus Publications remains neutral with regard to jurisdictional claims in published maps and institutional affiliations.
This study was partially supported by the Ministerio de Economía y Competitividad (Government of Spain) via research project CGL2015-64284-C2-1-R and PID2020-112908RB-I00 funded by 568 MCIN/AEI/10.13039/501100011033/FEDER “Una manera de hacer Europa”.
This study was developed within the framework of project 011-1365-2020-000075 CropStick: sentinel of salts, pH, nitrogen and nutrients, and deep percolation, financed by the Government of Navarre.
This paper was edited by Simeon Materechera and reviewed by six anonymous referees.
Alonso, A., Froidevaux, M., Javaux, M., Laloy, E., Mattern, S., Roisin, C., Vanclooster, M., and Bielders, C.: A hybrid method for characterizing tillage-induced soil physical quality at the profile scale with fine spatial details, Soil Till. Res., 216, 105236, https://doi.org/10.1016/j.still.2021.105236, 2022.
Angers, D. A., Recous, S., and Aita, C.: Fate of carbon and nitrogen in water-stable aggregates during decomposition of 13C15N-labelled wheat straw in situ, Eur. J. Soil Sci., 48, 295–300, https://doi.org/10.1111/j.1365-2389.1997.tb00549.x, 1997.
Armindo, R. A. and Wendroth, O.: Physical Soil Structure Evaluation based on Hydraulic Energy Functions, Soil Sci. Soc. Am. J., 80, 1167–1180, https://doi.org/10.2136/sssaj2016.03.0058, 2016.
Bacher, M. G., Schmidt, O., Bondi, G., Creamer, R., and Fenton, O.: Comparison of Soil Physical Quality Indicators Using Direct and Indirect Data Inputs Derived from a Combination of In-Situ and Ex-Situ Methods, Soil Sci. Soc. Am. J., 83, 5–17, https://doi.org/10.2136/sssaj2018.06.0218, 2019.
Beare, M. H., Hendrix, P. F., Cabrera, M. L., and Coleman, D. C.: Aggregate-Protected and Unprotected Organic Matter Pools in Conventional- and No-Tillage Soils, Soil Sci. Soc. Am. J., 58, 787–795, https://doi.org/10.2136/sssaj1994.03615995005800030021x, 1994.
Bescansa, P., Imaz, M. J., Virto, I., Enrique, A., and Hoogmoed, W. B.: Soil water retention as affected by tillage and residue management in semiarid Spain, Soil Till. Res., 87, 19–27, https://doi.org/10.1016/j.still.2005.02.028, 2006.
Borges, J. A. R., Pires, L. F., Cássaro, F. A. M., Auler, A. C., Rosa, J. A., Heck, R. J., and Roque, W. L.: X-ray computed tomography for assessing the effect of tillage systems on topsoil morphological attributes, Soil Till. Res., 189, 25–35, https://doi.org/10.1016/j.still.2018.12.019, 2019.
Bouma, J.: Soil science contributions towards Sustainable Development Goals and their implementation: Linking soil functions with ecosystem services, J. Plant Nutr. Soil Sc., 177, 111–120, https://doi.org/10.1002/jpln.201300646, 2014.
Bouma, J., Montanarella, L., and Evanylo, G.: The challenge for the soil science community to contribute to the implementation of the UN Sustainable Development Goals, Soil Use Manage., 35, 538–546, https://doi.org/10.1111/sum.12518, 2019.
Bouma, J., Pinto-correia, T., and Veerman, C.: Assessing the role of soils when developing sustainable agricultural production systems focused on achieving the un-sdgs and the eu green deal, Soil Syst., 5, https://doi.org/10.3390/soilsystems5030056, 2021.
Bouma, J., de Haan, J., and Dekkers, M. F. S.: Exploring Operational Procedures to Assess Ecosystem Services at Farm Level, including the Role of Soil Health, Soil Syst., 6, 34, https://doi.org/10.3390/soilsystems6020034, 2022.
Brooks, R. H. and Corey, A. T.: Hidraulic properties of porous media, edited by: Corey, A. T., Dils, R. E., and Yevdjevich, V. M., Colorado State University, Fort Collins, CO, https://mountainscholar.org/bitstream/handle/10217/61288/HydrologyPapers_n3.pdf (last access: 18 October 2022) 1964.
Bünemann, E. K., Bongiorno, G., Bai, Z., Creamer, R. E., De Deyn, G., de Goede, R., Fleskens, L., Geissen, V., Kuyper, T. W., Mäder, P., Pulleman, M., Sukkel, W., van Groenigen, J. W., and Brussaard, L.: Soil quality – A critical review, Soil Biol. Biochem., 120, 105–125, https://doi.org/10.1016/j.soilbio.2018.01.030, 2018.
Chenu, C., Angers, D. A., Barré, P., Derrien, D., Arrouays, D., and Balesdent, J.: Increasing organic stocks in agricultural soils: Knowledge gaps and potential innovations, Soil Till. Res., 188, 41–52, https://doi.org/10.1016/j.still.2018.04.011, 2019.
Costantini, E. A. C., Antichi, D., Almagro, M., Hedlund, K., Sarno, G., and Virto, I.: Local adaptation strategies to increase or maintain soil organic carbon content under arable farming in Europe: Inspirational ideas for setting operational groups within the European innovation partnership, J. Rural Stud., 79, 102–115, https://doi.org/10.1016/j.jrurstud.2020.08.005, 2020.
Cotrufo, M. F., Ranalli, M. G., Haddix, M. L., Six, J., and Lugato, E.: Soil carbon storage informed by particulate and mineral-associated organic matter, Nat. Geosci., 12, 989–994, https://doi.org/10.1038/s41561-019-0484-6, 2019.
Council of the European Union: Council Directive 91/676/EEC of 12 December 1991 concerning the protection of waters against pollution caused by nitrates from agricultural sources, L 269, 1–15, https://eur-lex.europa.eu/legal-content/EN/TXT/PDF/?uri=CELEX:01991L0676-20081211&from=EN (last access: 18 October 2022), 2008.
De Tourdonnet, S., Nozières, A., Barz, P., Chenu, C., Düring, R. A., Frielinghaus, M., Kõlli, R., Kubat, J., Magid, J., Medvedev, V., Michels, A., Müller, L., Netland, J., Nielsen, N. E., Nieves Mortensen, C., Picard, D., Quillet, J. C., Saulas, P., Tessier, D., Thinggaard, K., and Vandeputte, E.: Comprehensive inventory and assessment of existing knowledge on sustainable agriculture in the European platform of KASSA, edited by: Lahmar, R., Arrue, J. L., Denardin, J. E., Gupta, R. K., Ribeiro, M. F. F., and De Tourdonnet, S., CIRAD, Montpellier, 54 pp., ISBN 978-2-87614-646-4, 2007.
Dexter, A. R.: Soil physical quality: Part I. Theory, effects of soil texture, density, and organic matter, and effects on root growth, Geoderma, 120, 201–214, https://doi.org/10.1016/j.geoderma.2003.09.004, 2004a.
Dexter, A. R.: Soil physical quality: Part II. Friability, tillage, tilth and hard-setting, Geoderma, 120, 215–225, https://doi.org/10.1016/j.geoderma.2003.09.005, 2004b.
Dexter, A. R. and Bird, N. R. A.: Methods for predicting the optimum and the range of soil water contents for tillage based on the water retention curve, Soil Till. Res., 57, 203–212, https://doi.org/10.1016/S0167-1987(00)00154-9, 2001.
Dexter, A. R., Czyz, E. A., Richard, G., and Reszkowska, A.: A user-friendly water retention function that takes account of the textural and structural pore spaces in soil, Geoderma, 143, 243–253, https://doi.org/10.1016/j.geoderma.2007.11.010, 2008.
Dirksen, C. (Ed.): Soil physics measurements, Catena Verlag, Reiskirchen, Germany, 154 pp., https://www.cabdirect.org/cabdirect/abstract/20013083835 (last access: 18 October 2022), 1999.
Eekhout, J. P. C. and De Vente, J.: How soil erosion model conceptualization affects soil loss projections under climate change, Prog. Phys. Geogr., 44, 212–232, https://doi.org/10.1177/0309133319871937, 2020.
Elliott, E. T.: Aggregate Structure and Carbon, Nitrogen, and Phosphorus in Native and Cultivated Soils, Soil Sci. Soc. Am. J., 50, 627–633, https://doi.org/10.2136/sssaj1986.03615995005000030017x, 1986.
Espejo-Pérez, A. J., Rodríguez-Lizana, A., Ordóñez, R., and Giráldez, J. V.: Soil Loss and Runoff Reduction in Olive-Tree Dry-Farming with Cover Crops, Soil Sci. Soc. Am. J., 77, 2140–2148, https://doi.org/10.2136/sssaj2013.06.0250, 2013.
FAO: A protocol for measurement, monitoring, reporting and verification of soil organic carbon in agricultural landscapes – GSOC-MRV Protocol, FAO, Rome, 140 pp., https://doi.org/10.4060/cb0509en, 2020.
Fernández-Ugalde, O., Virto, I., Bescansa, P., Imaz, M. J., Enrique, A., and Karlen, D. L.: No-tillage improvement of soil physical quality in calcareous, degradation-prone, semiarid soils, Soil Till. Res., 106, 29–35, https://doi.org/10.1016/j.still.2009.09.012, 2009.
Fernández-Ugalde, O., Virto, I., Barré, P., Gartzia-Bengoetxea, N., Enrique, A., Imaz, M. J., and Bescansa, P.: Effect of carbonates on the hierarchical model of aggregation in calcareous semi-arid Mediterranean soils, Geoderma, 164, 203–214, https://doi.org/10.1016/j.geoderma.2011.06.008, 2011.
Fernández-Ugalde, O., Barré, P., Virto, I., Hubert, F., Billiou, D., and Chenu, C.: Does phyllosilicate mineralogy explain organic matter stabilization in different particle-size fractions in a 19-year C3/C4 chronosequence in a temperate Cambisol?, Geoderma, 264, 171–178, https://doi.org/10.1016/j.geoderma.2015.10.017, 2016.
Fetting, C.: The European Green Deal, ESDN Report, ESDN Office, Vienna, https://www.esdn.eu/fileadmin/ESDN_Reports/ESDN_Report_2_2020.pdf (last access: 18 October 2022), 2020.
Fuentes-Guevara, M. D., Armindo, R. A., Timm, L. C., and Faria, L. C.: Examining the land leveling impacts on the physical quality of lowland soils in Southern Brazil, Soil Till. Res., 215, 105217, https://doi.org/10.1016/j.still.2021.105217, 2022.
Gao, L., Wang, B., Li, S., Wu, H., Wu, X., Liang, G., Gong, D., Zhang, X., Cai, D., and Degré, A.: Soil wet aggregate distribution and pore size distribution under different tillage systems after 16 years in the Loess Plateau of China, Catena, 173, 38–47, https://doi.org/10.1016/j.catena.2018.09.043, 2019.
Gobierno de Navarra Meteorología y Climatología de Navarra: Meteorología y Climatología de Navarra, http://meteo.navarra.es/climatologia/selfichaclima.cfm?IDEstacion=81&tipo=MAN, last access: 30 August 2022.
Golchin, A., Oades, J. M., Skjemstad, J. O., and Clarke, P.: Soil structure and carbon cycling, Aust. J. Soil Res., 32, 1043–1063, https://doi.org/10.1071/SR9941043, 1994.
Gómez, J. A., Sobrinho, T. A., Giráldez, J. V., and Fereres, E.: Soil management effects on runoff, erosion and soil properties in an olive grove of Southern Spain, Soil Till. Res., 102, 5–13, https://doi.org/10.1016/j.still.2008.05.005, 2009.
Greenland, D. J. and Pereira, H. C.: Soil damage by intensive arable cultivation: temporary or permanent?, Philos. T. Roy. Soc. B, 281, 193–208, https://doi.org/10.1098/rstb.1977.0133, 1977.
Grillakis, M. G., Polykretis, C., and Alexakis, D. D.: Past and projected climate change impacts on rainfall erosivity: Advancing our knowledge for the eastern Mediterranean island of Crete, Catena, 193, 104625, https://doi.org/10.1016/j.catena.2020.104625, 2020.
Hendershot, W. H. and Lalande, H.: Chapter 16. Soil Reactionand Exchangeable Acidity, in: Soil Sampling and Methods of Analysis, edited by: Carter, M. R., CRC Press LLC, Boca Ratón, FL, USA, 141–142, 1993.
IBM Corp: IBM SPSS Statistics for Windows, Version 28.0. Armonk, NY, IBM Corp, 2021.
Imhoff, S., Ghiberto, P. J., Grioni, A., and Gay, J. P.: Porosity characterization of Argiudolls under different management systems in the Argentine Flat Pampa, Geoderma, 158, 268–274, https://doi.org/10.1016/j.geoderma.2010.05.005, 2010.
IUSS Working Group WRB: World Reference Base for Soil Resources 2014, update 2015 International soil classification system for naming soils and creating legends for soil maps, World Soil Resources Reports No. 106. FAO, Rome, 2015.
Jarvis, S.: Landmark papers, Eur. J. Soil Sci., 63, 1–21, https://doi.org/10.1111/j.1365-2389.2011.01408.x, 2012.
Jastrow, J. D.: Soil aggregate formation and the accrual of particulate and mineral-associated organic matter, Soil Biol. Biochem., 28, 665–676, https://doi.org/10.1016/0038-0717(95)00159-X, 1996.
Jensen, J. L., Schjønning, P., Watts, C. W., Christensen, B. T., and Munkholm, L. J.: Soil Water Retention: Uni-Modal Models of Pore-Size Distribution Neglect Impacts of Soil Management, Soil Sci. Soc. Am. J., 83, 18–26, https://doi.org/10.2136/sssaj2018.06.0238, 2019.
Klute, A.: Some Theoretical Aspects of the Flow of Water in Unsaturated Soils, Soil Sci. Soc. Am. J., 16, 144–148, https://doi.org/10.2136/sssaj1952.03615995001600020008x, 1952.
Kosugi, K., Hopmans, J. W., and Dane, J. H.: Parametric Models, in: Methods of Soil Analysis. Part 4 – Physical Methods, edited by: Dane, J. H. and Clarke Topp, G., SSSA, Madison Winsconsin, 739–757, 2002.
Lahmar, R., Arrúe, J. L., Denardin, J. E., Gupta, R. K., Ribeiro, M. F. S., de Tourdonnet, S., Abrol, I. P., Barz, P., de Benito, A., Bianchini, A., and Al, E.: Knowledge Assessment and Sharing on Sustainable Agriculture. Synthesis Report;, Montpellier, France, 125 pp., 2007.
Lal, R., Horn, R., and Kosaki, T. (Eds.): Soil and Sustainable Development Goals, Catena Soils Science, Stuttgart, Germany, 196 pp., https://www.schweizerbart.de/publications/detail/isbn/9783510654253/Soil%5c_and%5c_Sustainable%5c_Development%5c_Goals%5c (last access: 18 October 2022), 2018.
Lal, R., Bouma, J., Brevik, E., Dawson, L., Field, D. J., Glaser, B., Hatano, R., Hartemink, A. E., Kosaki, T., Lascelles, B., Monger, C., Muggler, C., Ndzana, G. M., Norra, S., Pan, X., Paradelo, R., Reyes-Sánchez, L. B., Sandén, T., Singh, B. R., Spiegel, H., Yanai, J., and Zhang, J.: Soils and sustainable development goals of the United Nations: An International Union of Soil Sciences perspective, Geoderma Reg., 25, e00398, https://doi.org/10.1016/j.geodrs.2021.e00398, 2021.
Lehmann, J. and Kleber, M.: The contentious nature of soil organic matter, Nature, 528, 60–68, https://doi.org/10.1038/nature16069, 2015.
Lipiec, J., Kuś, J., Słowińska-Jurkiewicz, A., and Nosalewicz, A.: Soil porosity and water infiltration as influenced by tillage methods, Soil Till. Res., 89, 210–220, https://doi.org/10.1016/j.still.2005.07.012, 2006.
Milly, P. C. D.: Estimation of Brooks-Corey Parameters, Water Resour. Res., 23, 1085–1089, 1987.
Minasny, B. and McBratney, A. B.: Limited effect of organic matter on soil available water capacity, Eur. J. Soil Sci., 69, 39–47, https://doi.org/10.1111/ejss.12475, 2018.
Mosavi, A., Sajedi-Hosseini, F., Choubin, B., Taromideh, F., Rahi, G., and Dineva, A. A.: Susceptibility mapping of soil water erosion using machine learning models, Water, 12, 1–17, https://doi.org/10.3390/w12071995, 2020.
Oades, J. M.: Soil organic matter and structural stability: mechanisms and implications for management, Plant Soil, 76, 319–337, https://doi.org/10.1007/BF02205590, 1984.
Oliveira, M., Barré, P., Trindade, H., and Virto, I.: Different efficiencies of grain legumes in crop rotations to improve soil aggregation and organic carbon in the short-term in a sandy Cambisol, Soil Till. Res., 186, 23–35, https://doi.org/10.1016/j.still.2018.10.003, 2019.
Or, D., Keller, T., and Schlesinger, W. H.: Natural and managed soil structure: On the fragile scaffolding for soil functioning, Soil Till. Res., 208, 104912, https://doi.org/10.1016/j.still.2020.104912, 2021.
Ordóñez Fernández, R., González Fernández, P., Giráldez Cervera, J. V., and Perea Torres, F.: Soil properties and crop yields after 21 years of direct drilling trials in southern Spain, Soil Till. Res., 94, 47–54, https://doi.org/10.1016/j.still.2006.07.003, 2007.
Pagliai, M., La Marca, M., Lucamante, G., and Genovese, L.: Effects of zero and conventional tillage on the length and irregularity of elongated pores in a clay loam soil under viticulture, Soil Till. Res., 4, 433–444, https://doi.org/10.1016/0167-1987(84)90051-5, 1984.
Pagliai, M., Vignozzi, N., and Pellegrini, S.: Soil structure and the effect of management practices, Soil Till. Res., 79, 131–143, https://doi.org/10.1016/j.still.2004.07.002, 2004.
Panagos, P., Ballabio, C., Himics, M., Scarpa, S., Matthews, F., Bogonos, M., Poesen, J., and Borrelli, P.: Projections of soil loss by water erosion in Europe by 2050, Environ. Sci. Policy, 124, 380–392, https://doi.org/10.1016/j.envsci.2021.07.012, 2021.
Panagos, P., Montanarella, L., Barbero, M., Schneegans, A., Aguglia, L., and Jones, A.: Soil priorities in the European Union, Geoderma Reg., 29, e00510, https://doi.org/10.1016/j.geodrs.2022.e00510, 2022.
Pansu, M. and Gautheyrou, J. (Eds.): Chapter 17. Carbonates, in: Handbook of Soil Analysis. Mineralogical, Organic and Inorganic Methods, Springer Netherlands, Dordrecht, the Netherlands, 593–601, https://doi.org/10.1007/978-3-540-31211-6, 2003a.
Pansu, M. and Gautheyrou, J. (Eds.): Chapter 18. Soluble Salts, in: Handbook of Soil Analysis. Mineralogical, Organic and Inorganic Methods, Springer Netherlands, Dordrecht, the Netherlands, 608–609, https://doi.org/10.1007/978-3-540-31211-6, 2003b.
Paroissien, J. B., Darboux, F., Couturier, A., Devillers, B., Mouillot, F., Raclot, D., and Le Bissonnais, Y.: A method for modeling the effects of climate and land use changes on erosion and sustainability of soil in a Mediterranean watershed (Languedoc, France), J. Environ. Manage., 150, 57–68, https://doi.org/10.1016/j.jenvman.2014.10.034, 2015.
Paul, E. A.: The nature and dynamics of soil organic matter: Plant inputs, microbial transformations, and organic matter stabilization, Soil Biol. Biochem., 98, 109–126, https://doi.org/10.1016/j.soilbio.2016.04.001, 2016.
Peel, M. C., Finlayson, B. L., and McMahon, T. A.: Updated world map of the Köppen-Geiger climate classification, Hydrol. Earth Syst. Sci., 11, 1633–1644, https://doi.org/10.5194/hess-11-1633-2007, 2007.
Pertassek, T., Peters, A., and Durner, W.: HYPROP-FIT Software User's Manual, UMS GmbH, Gmunder Str. 37, 81379 München, Germany, 66 pp., 2015.
Peters, A. and Durner, W.: Simplified evaporation method for determining soil hydraulic properties, J. Hydrol., 356, 147–162, https://doi.org/10.1016/j.jhydrol.2008.04.016, 2008.
Pires, L. F., Borges, F. S., Passoni, S., and Pereira, A. B.: Soil Pore Characterization Using Free Software and a Portable Optical Microscope, Pedosphere, 23, 503–510, https://doi.org/10.1016/S1002-0160(13)60043-0, 2013.
Pires, L. F., Borges, J. A. R., Rosa, J. A., Cooper, M., Heck, R. J., Passoni, S., and Roque, W. L.: Soil structure changes induced by tillage systems, Soil Till. Res., 165, 66–79, https://doi.org/10.1016/j.still.2016.07.010, 2017.
Pittelkow, C. M., Liang, X., Linquist, B. A., Van Groenigen, L. J., Lee, J., Lundy, M. E., Van Gestel, N., Six, J., Venterea, R. T., and Van Kessel, C.: Productivity limits and potentials of the principles of conservation agriculture, Nature, 517, 365–368, https://doi.org/10.1038/nature13809, 2015.
Preston-Mafham, J., Boddy, L., and Randerson, P. F.: Analysis of microbial community functional diversity using sole-carbon-source utilisation profiles – A critique, FEMS Microbiol. Ecol., 42, 1–14, https://doi.org/10.1016/S0168-6496(02)00324-0, 2002.
Rabot, E., Wiesmeier, M., Schlüter, S., and Vogel, H. J.: Soil structure as an indicator of soil functions: A review, Geoderma, 314, 122–137, https://doi.org/10.1016/j.geoderma.2017.11.009, 2018.
Rasmussen, C., Heckman, K., Wieder, W. R., Keiluweit, M., Lawrence, C. R., Berhe, A. A., Blankinship, J. C., Crow, S. E., Druhan, J. L., Hicks Pries, C. E., Marin-Spiotta, E., Plante, A. F., Schädel, C., Schimel, J. P., Sierra, C. A., Thompson, A., and Wagai, R.: Beyond clay: towards an improved set of variables for predicting soil organic matter content, Biogeochemistry, 137, 297–306, https://doi.org/10.1007/s10533-018-0424-3, 2018.
Reynolds, W. D., Drury, C. F., Tan, C. S., Fox, C. A., and Yang, X. M.: Use of indicators and pore volume-function characteristics to quantify soil physical quality, Geoderma, 152, 252–263, https://doi.org/10.1016/j.geoderma.2009.06.009, 2009.
Rowley, M. C., Grand, S., and Verrecchia, É. P.: Calcium-mediated stabilisation of soil organic carbon, Biogeochemistry, 137, 27–49, https://doi.org/10.1007/s10533-017-0410-1, 2018.
Rowley, M. C., Grand, S., Spangenberg, J. E., and Verrecchia, E. P.: Evidence linking calcium to increased organo-mineral association in soils, Biogeochemistry, 153, 223–241, https://doi.org/10.1007/s10533-021-00779-7, 2021.
Sartori, F., Piccoli, I., Polese, R., and Berti, A.: Transition to conservation agriculture: How tillage intensity and covering affect soil physical parameters, Soil, 8, 213–222, https://doi.org/10.5194/soil-8-213-2022, 2022.
Schindler, U.: Ein Schnellverfahren zur Messung der Wasserleitfahigkeit im teilgesattigten Boden and Stechzylinderproben, Arch. fur Acker- und Pflanzenbau und Bodenkd., 24, 1–7, 1980.
Schindler, U., Durner, W., von Unold, G., Mueller, L., and Wieland, R.: The evaporation method: Extending the measurement range of soil hydraulic properties using the air-entry pressure of the ceramic cup, J. Plant Nutr. Soil Sc., 173, 563–572, https://doi.org/10.1002/jpln.200900201, 2010.
Six, J., Elliot, E. T., and Paustian, K.: Aggregate and Soil Organic Matter Dynamics under Conventional and No-Tillage Systems, Soil Sci. Soc. Am. J., 63, 1350–1358, 1999.
Six, J., Callewaert, P., Lenders, S., De Gryze, S., Morris, S. J., Gregorich, E. G., Paul, E. A., and Paustian, K.: Measuring and Understanding Carbon Storage in Afforested Soils by Physical Fractionation, Soil Sci. Soc. Am. J., 66, 1981–1987, https://doi.org/10.2136/sssaj2002.1981, 2002.
Six, J., Bossuyt, H., Degryze, S., and Denef, K.: A history of research on the link between (micro)aggregates, soil biota, and soil organic matter dynamics, Soil Till. Res., 79, 7–31, https://doi.org/10.1016/j.still.2004.03.008, 2004.
Soil Survey Staff: Keys to soil taxonomy, 12th Edn., USDA-Natural Resour. Conserv. Serv. Washington, DC., 360, https://www.nrcs.usda.gov/wps/portal/nrcs/detail/soils/survey/class/taxonomy/?cid=nrcs142p2_053580 (last access: 18 October 2022), 2014.
Taina, I. A., Heck, R. J., Deen, W., and Ma, E. Y. T.: Quantification of freeze-thaw related structure in cultivated topsoils using X-ray computer tomography, Can. J. Soil Sci., 93, 533–553, https://doi.org/10.4141/CJSS2012-044, 2013.
Tiessen, H. and Moir, J. O.: Total and organic C, in: Soil Sampling and Methods of Analysis, edited by: Carter, M., CRC Press LLC, Boca Raton, FL, USA, 187–191, https://doi.org/10.1201/9781420005271, 1993.
Tisdall, J. M. and Oades, J. M.: Organic matter and water-stable aggregates in soils, J. Soil Sci., 33, 141–163, https://doi.org/10.1111/j.1365-2389.1982.tb01755.x, 1982.
Tuzzin de Moraes, M., Debiasi, H., Carlesso, R., Cezar Franchini, J., Rodrigues da Silva, V., and Bonini da Luz, F.: Soil physical quality on tillage and cropping systems after two decades in the subtropical region of Brazil, Soil Till. Res., 155, 351–362, https://doi.org/10.1016/j.still.2015.07.015, 2016.
Vance, E. D., Brookes, D. S., and Jenkinson, D. S.: An extraction method for measuring soil microbial biomass C, Soil Biol. Biochem., 19, 703–707, https://doi.org/10.1016/0038-0717(87)90052-6, 1987.
van Genuchten, M. T.: A Closed-form Equation for Predicting the Hydraulic Conductivity of Unsaturated Soils, Soil Sci. Soc. Am. J., 44, 892–898, https://doi.org/10.2136/sssaj1980.03615995004400050002x, 1980.
van Genuchten, M. T. and Nielsen, D. R.: On describing and predicting the hydraulic properties of unsaturated soils, Ann. Geophys., 3, 615–628, 1985.
Verhulst, N., Govaerts, B., Verachtert, E., Mezzalama, M., Wall, P. C., Chocobar, A., Deckers, J., and Sayre, K. D.: Conservation agriculture, improving soil quality for sustainable production systems?, in: Food Security and Soil Quality, edited by: Lal, R. and Stewart, B. A., CRC Press, 137–208, https://doi.org/10.1201/EBK1439800577, 2010.
Virto, I., Imaz, M. J., Enrique, A., Hoogmoed, W., and Bescansa, P.: Burning crop residues under no-till in semi-arid land, Northern Spain – Effects on soil organic matter, aggregation, and earthworm populations, Aust. J. Soil Res., 45, 414–421, https://doi.org/10.1071/SR07021, 2007.
Virto, I., Barré, P., Burlot, A., and Chenu, C.: Carbon input differences as the main factor explaining the variability in soil organic C storage in no-tilled compared to inversion tilled agrosystems, Biogeochemistry, 108, 17–26, https://doi.org/10.1007/s10533-011-9600-4, 2012.
Virto, I., Imaz, M. J., Fernández-Ugalde, O., Gartzia-Bengoetxea, N., Enrique, A., and Bescansa, P.: Soil degradation and soil quality in Western Europe: Current situation and future perspectives, Sustain., 7, 313–365, https://doi.org/10.3390/su7010313, 2015.
Wardak, D. L. R., Padia, F. N., de Heer, M. I., Sturrock, C. J., and Mooney, S. J.: Zero tillage has important consequences for soil pore architecture and hydraulic transport: A review, Geoderma, 422, 115927, https://doi.org/10.1016/j.geoderma.2022.115927, 2022.
Warrick, A. W. (Ed.): Soil Water Dynamics, Oxford University Press, Oxford, UK, https://books.google.es/books?id=WV3nCwAAQBAJ&printsec=frontcover&hl=es&source=gbs_ge_summary_r&cad=0#v=onepage&q&f=false (last access: 18 October 2022), 2003.
Weil, R. and Brady, N.: The Nature and Properties of Soils, 15th Edn., edited by: Fox, D., Pearson, https://www.pearson.com/en-gb/subject-catalog/p/nature-and-properties-of-soils-the-global-edition/P200000005713/9781292162249 (last access: 18 October 2022), 2017.
Wiecheteck, L. H., Giarola, N. F. B., de Lima, R. P., Tormena, C. A., Torres, L. C., and de Paula, A. L.: Comparing the classical permanent wilting point concept of soil (−15,000 hPa) to biological wilting of wheat and barley plants under contrasting soil textures, Agr. Water Manage., 230, 105965, https://doi.org/10.1016/j.agwat.2019.105965, 2020.
Yagüe, M. R., Domingo-Olivé, F., Bosch-Serra, À. D., Poch, R. M., and Boixadera, J.: Dairy Cattle Manure Effects on Soil Quality: Porosity, Earthworms, Aggregates and Soil Organic Carbon Fractions, Land Degrad. Dev., 27, 1753–1762, https://doi.org/10.1002/ldr.2477, 2016.
Zak, J. C., Willig, M. R., Moorhead, D. L., and Wildman, H. G.: Functional diversity of microbial communities: A quantitative approach, Soil Biol. Biochem., 26, 1101–1108, https://doi.org/10.1016/0038-0717(94)90131-7, 1994.