the Creative Commons Attribution 4.0 License.
the Creative Commons Attribution 4.0 License.
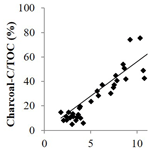
Identification of thermal signature and quantification of charcoal in soil using differential scanning calorimetry and benzene polycarboxylic acid (BPCA) markers
Brieuc Hardy
Nils Borchard
Jens Leifeld
Black carbon (BC) plays an important role in terrestrial carbon storage and can sustainably improve soil fertility. However, the accurate quantification of BC remains critical to fully unravelling the functions and dynamics of BC in soil. In this study, we explored the potential of differential scanning calorimetry (DSC) to identify, characterize and quantify charcoal in the soil of pre-industrial charcoal kiln sites from various forest and cropland areas in Belgium and Germany. Pre-industrial charcoals and uncharred soil organic matter (SOM) demonstrated a distinct thermal signature that could be used to distinguish between them, with charcoal being more thermally stable than SOM. The DSC pattern of charcoals was characterized by one to three specific exothermic peaks, varying in size and position depending on soil conditions. Our data suggest that the thermal moieties within charcoal depend on the strength of chemical bonds of C atoms (increasing with the degree of aromatic condensation and decreasing with weathering) and on the activation energy required to initiate combustion. Despite the specific thermal features of charcoal, its decomposition spans a wide range of temperatures that overlaps with the thermal signature of uncharred SOM. This stresses the challenge of BC quantification in soil and hinders the use of cut-off temperatures to accurately quantify charcoal in soil. Therefore, charcoal-C content was estimated from the relative height of exothermic peaks, attributed either to the combustion of charcoal or SOM. For a selection of 45 soil samples, charcoal-C content estimated by DSC was compared to benzene polycarboxylic acid (BPCA) abundance, a widely used method to quantify BC in soil. The two methods correlated strongly (R2=0.97), with BPCA C representing about one-fifth of DSC-derived charcoal C. This reinforces the view that operationally defined BC content has an absolute quantitative value only if the recovery rate is controlled, which is very complicated for many case studies. Overall, our results demonstrate that dynamic thermal analysis is largely under-exploited despite providing quantitatively interpretable information across the continuum of SOM.
- Article
(2384 KB) - Full-text XML
- BibTeX
- EndNote
Black carbon (BC) is the solid residue of the incomplete combustion of biomass and fossil fuel, comprising a wide range of thermally altered materials from slightly charred biomass to highly recalcitrant condensates such as soot (Goldberg, 1985; Schmidt and Noack, 2000). Most terrestrial BC is stored in soil ((Forbes et al., 2006; Leifeld et al., 2018; Preston and Schmidt, 2006; Reisser et al., 2016; Santín et al., 2016), where it has a longer residence time than uncharred organic matter (e.g. Wang et al., 2016; Wolf et al., 2013). The increased resistance of BC to (a)biotic degradation has been related to its fused aromatic ring structure (Solomon et al., 2007; Wang et al., 2016). BC has received much interest from soil scientists because it plays an important role in the provision of ecosystems services such as terrestrial carbon storage (Czimczik and Masiello, 2007; Knicker, 2011; Masiello, 2004; Preston and Schmidt, 2006; Schmidt and Noack, 2000) and sustainable soil fertility (Glaser et al., 2001, 2002; Glaser and Birk, 2012; Schmidt et al., 2021). However, the accurate quantification of BC in environmental matrices remains a critical issue, making the role of BC in geochemical processes unclear (Mukherjee and Kumar, 2021).
According to the general definition reported earlier, BC comprises a wide range of materials with no clear-cut boundaries, which makes quantification difficult (Kappenberg et al., 2016; Roth et al., 2012; Schmidt et al., 2001). The various forms of BC cover a broad molecular continuum (Hammes et al., 2007; Masiello, 2004) that reflects contrasting conditions of formation (Keiluweit et al., 2010; Wiedemeier et al., 2015a). Moreover, properties of chars produced at relatively low temperature overlap with those of uncharred organic compounds naturally present in soil. Consequently, quantification of BC relies on operational definitions depending on specific objectives defined by researchers from different fields in atmospheric, soil, sediment and palaeoenvironmental sciences (Hammes et al., 2007; Schmidt et al., 2001). The various methods produce systematic differences because they recover different fractions of the BC continuum and do not all completely isolate BC from other carbon compounds (Hammes et al., 2007; Roth et al., 2012). As a result, Schmidt et al. (2001) reported that variation in the BC content estimated by four different methods for one individual sample was more than 2 orders of magnitude.
Typically, five categories of techniques of identification and quantification of BC in soil and sediments are distinguished: physical, thermal, chemical, spectroscopic and molecular marker techniques (Bird et al., 2015; Hammes et al., 2007). Thermal and (thermo-)chemical separation techniques involve exposing the sample to an oxidative treatment under standard conditions. Carbon surviving oxidation is determined by mass loss or elemental analysis and operationally defined as BC. A molecular marker technique that is widely used by soil scientists consists of the quantification of benzene polycarboxylic acid (BPCA) markers liberated by digestion of BC in an acid medium (Brodowski et al., 2005; Glaser et al., 1998). This procedure has two main advantages: (i) it relies on the chemical decomposition of BC into markers that are specific to BC, related to its high aromaticity, and (ii) it provides information on the degree of aromatic condensation of BC according to the number of carboxyl groups on the edge of benzene in the BPCA markers (Glaser et al., 1998). However, this method has known limitations, as soil type and soil organic matter content may affect both the quantification of total BC and the pattern of BPCA markers (Brodowski et al., 2005; Kappenberg et al., 2016). In particular, the recovery of BPCA C may vary depending on the quality of BC (Hammes et al., 2007; Roth et al., 2012). The less-condensed fraction of chars is suspected to be completely decomposed by the strong oxidative attack (Glaser et al., 1998), whereas the most refractory, graphitized forms of BC may resist digestion preceding BPCA analysis (Brodowski et al., 2005; Roth et al., 2012). As a result, BPCA C has been shown to correspond to a maximum of of total C in charcoal under optimal conditions of recovery (Glaser et al., 1998). This factor of 2.27 is often used as a conservative conversion factor to estimate total BC content from BPCA C extracted from soil, although it has been criticized for its validity (Glaser et al., 1998; Schneider et al., 2010).
Among these techniques used for BC quantification, thermal methods are convenient because they are rapid, reproducible, inexpensive and require little sample preparation (Plante et al., 2009). Thermal resistance has been related to the biological availability of chars (Harvey et al., 2012; Plante et al., 2011) and to the residence time of BC in soil, as revealed by 14C measurements (Leifeld et al., 2015; Plante et al., 2013). Static thermal methods rely on cut-off temperatures to distinguish between BC and non-BC components (e.g. Gustafsson et al., 2001). However, the widely used technique of chemo-thermal oxidation at 375 ∘C is calibrated for soot (Elmquist et al., 2004) and has been shown to recover only 0 % to 44 % of C in chars, with no survival for those produced at <850 ∘C (Nguyen et al., 2004). In contrast to static methods, dynamic thermal analysis techniques like thermogravimetry and differential scanning calorimetry (DSC) have the potential to provide information about the entire continuum of materials in soil organic matter (SOM) by scanning a sample over a wide range of temperatures (Leifeld, 2007; Plante et al., 2009). Leifeld (2007) highlighted that BC has a specific thermal signature that allows unambiguous differentiation from uncharred SOM, as BC is systematically more thermally stable. Among materials potentially interfering with the signature of BC, only bituminous coal had a thermal stability comparable with that of chars.
In this study, we explored the analytical potential of DSC to identify and quantify charcoal C in the soil of pre-industrial charcoal kiln sites, also referred to as relict charcoal hearths in the literature. Charcoal kiln sites are platforms of in situ charcoal production by the earth mound kiln method (Schenkel et al., 1998), which operated at maximal temperatures of 400–450 ∘C (Emrich, 1985). They are widespread in the historical forest areas in Europe, as charcoal was the only fuel used for smelting and steel production for a long period of time (Hardy et al., 2016; Samojlik et al., 2013). These sites have received increasing attention in recent years, particularly as proxies for long-term soil amendment with biochar (Borchard et al., 2014; Burgeon et al., 2021; Dehkordi et al., 2020; Hardy et al., 2017a; Hirsch et al., 2018; Kerré et al., 2017; Lasota et al., 2021; Mastrolonardo et al., 2019; Pollet et al., 2022; Schneider et al., 2018; Zanutel et al., 2021), which is a promising technology for sequestering carbon in soil while maintaining or improving soil fertility (Laird, 2008; Lehmann, 2007).
Three specific objectives were addressed here: we aimed to (i) characterize the DSC thermal signature of charcoal in soil relative to that of uncharred SOM, (ii) quantify charcoal C in soil based on specific charcoal features of the thermograms, and (iii) compare the content of charcoal C in soil estimated by DSC to the content of total BC estimated by the BPCA method. To meet these goals, we analysed charcoal-rich soils sampled at various pre-industrial charcoal kiln sites in Wallonia (Belgium) and Siegerland and Eifel (Germany).
2.1 Soil samples
2.1.1 Soils of Belgium
Two series of organo-mineral topsoil samples of kiln and adjacent reference soils from forest (sampled by (sub)horizons of variable depth; N=38; described by Hardy et al., 2016) and cropland (0–25 cm depth; N=34; described by Hardy et al., 2017a) were analysed. Briefly, forest soils cover a wide range of textural classes, from sand to clay loam. Reference soil types are Arenosols, Cambisols, Luvisols or Podzols according to the World Reference Base (WRB) 2014 classification (IUSS Working Group WRB, 2014). They are mainly strongly acidic, except for three Cambisols developed on calcareous parent rocks (limestone, dolostone and marl). In cropland, the main reference soil types are haplic Luvisols (15 sites) according to the WRB 2014 classification (IUSS Working Group WRB, 2014). One site was identified as a eutric Cambisol and one as a colluvic Regosol. The particle-size analysis of topsoil (USDA texture; IUSS Working Group WRB, 2014) indicated that the soil texture was silt loam at 15 sites and loam at 2 sites. In Wallonia (southern Belgium), the climate is oceanic and cold temperate, with a mean annual temperature of between 6.4 and 9.5 ∘C and rainfall of 750 to 1400 mm. Charcoal production virtually ceased in the early 19th century, when coke replaced charcoal as an industrial fuel in ironworking, and had completely stopped by 1860 (Evrard, 1956). Therefore, we can reasonably assume that charcoal was deposited >150 years ago.
To investigate the effect of the mineralogical background on the thermal signature of soil, we also selected five subsoil samples from relatively clay-rich argic horizons from forested and cultivated Luvisols. Subsoil samples were treated with 6 % H2O2 at 70 ∘C for 30 d to oxidize SOM with a limited effect on soil mineralogy. The content of soil organic carbon (SOC) that survived oxidation ranged from 0.50 to 0.91 g kg−1 according to elemental analysis.
2.1.2 Soils of Germany
For the comparison of DSC results with BPCA biomarkers, we also analysed 45 kiln and reference forest topsoil samples (0–5, 5–20 and occasionally 20–25 cm) from 10 sites previously benchmarked with BPCA markers (Borchard et al., 2014) BPCA markers were determined according to the procedure of Brodowski et al. (2005). Five sites were located in the Siegerland region and five in the Eifel region of Germany. In Siegerland, the soils were leptic Cambisols (IUSS Working Group WRB, 2014) developed on acidic rock, whereas the soils from the Eifel region were haplic Luvisols, mollic Leptosols and leptic Cambisols (IUSS Working Group WRB, 2014) formed from the weathering of calcareous rock (Borchard et al., 2014). Soils in Siegerland were very acidic, with median pH values of 3.9, whereas soils in Eifel were base-rich, with a median pH value of 5.5 (Borchard et al., 2014). In both regions (Siegerland and Eifel), the climate is cold temperate with mean annual temperatures of 8.9 and 7.7 ∘C and mean annual precipitation totals of 946 and 717 mm respectively. The sites were abandoned >60 years ago (Borchard et al., 2014).
2.2 Sample preparation and carbon analysis
All soils were air-dried at a maximum of 40 ∘C until constant weight was reached. They were then gently ground and sieved to 2 mm. Before the DSC and BPCA analyses, the <2 mm fraction of each soil was ground to powder with an oscillating ring mill (samples from Belgium) or a ball mill (samples from Germany). The inorganic-C content was measured by the modified-pressure calcimeter method (Sherrod et al., 2002). The total content of C was determined by dry combustion and was corrected for inorganic C to obtain the total organic carbon (TOC) content, which includes uncharred SOC and charcoal C. Prior to DSC analysis, samples exceeding 60 g kg−1 of TOC were diluted with Al2O3 and homogenized in a ball mill.
2.3 Charcoal pieces
In order to constrain the thermal signature of aged charcoal in soil, macro-fragments of charcoal were extracted from kiln soil for a selection of 20 sites from Belgium (Hardy et al., 2017b). This approach was based on the assumption that large charcoal particles have a thermal signature representative of charcoal residues in soil regardless of their size, provided that weathering over time has not completely erased the aromatic character specific to chars. Charcoal particles >1 mm were separated from about 2 kg of soil by wet sieving. The residue, containing charcoal particles, was rinsed abundantly with demineralized water and air-dried. Charcoal pieces were separated from inorganic material by flotation in water and then rinsed again several times with demineralized water in a 500 mL beaker until the water was clear. Plant residues were removed manually. Between 50 and >400 charcoal pieces were collected for each site.
To compare the thermal characteristics of aged charcoal to those of charcoal that had never been deposited in soil (referred to here as “fresh” charcoal), we also analysed birch charcoal that was produced in a traditional mound kiln in August 2012 (Hardy et al., 2016). Prior to analysis, charcoal particles were ground to a powder with an agate pestle and mortar. In total, 21 charcoal samples were analysed.
2.4 Differential scanning calorimetry analysis
Soils and charcoals were analysed by heat flux DSC with a DSC Q100 (TA Instruments), which measures the temperature difference between the sample and an empty reference next to it that is subjected to the same heating programme. A heat flow rate is calculated based on the voltage signal corresponding to the difference in temperature between the sample and the empty reference (Plante et al., 2009). Between 15 and 25 mg of soil ground to powder was weighed into an aluminium pan and scanned under a flow of 50 mL min−1 synthetic air from room temperature to 600 ∘C, at a heating rate of 10 ∘C min−1 (Leifeld, 2007).
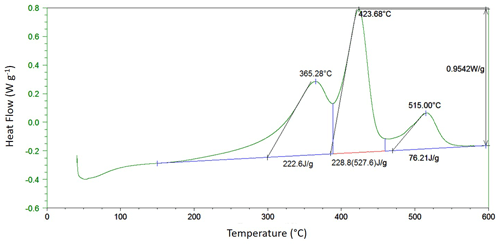
Figure 1Measurement of characteristics from differential scanning calorimetry thermograms (green line). After identification of peak maxima, peak height (W g−1) was measured as the maximum deviation from a linear baseline drawn between 150 and 600 ∘C, as illustrated for the main peak of the thermogram (0.9542 W g−1). Total heat release (527.6 J g−1) corresponds to the surface of the graph delimited by the linear baseline. Peak area (J g−1) was obtained by identifying the minimum between two adjacent peaks and splitting the peaks perpendicularly to the baseline. The black lines indicate the maximum rate of reaction associated with each maximum, and they cross the baseline at the onset point (the temperature at which oxidation of the material starts).
After subtraction of a linear baseline drawn between 150 and 600 ∘C, peak temperatures (∘C), peak heights (W g−1), temperature at 50 % heat release (T50) and total heat of reaction (J g−1) were measured for each DSC thermogram with the Universal Analysis 2000 software (TA Instruments). Peak area (J g−1) was measured for charcoal pieces only, by identifying the minimum between two peaks and splitting the peaks perpendicularly to the baseline (Fig. 1). From repeated measurements (n=6) on one sample, we estimated a 95 % confidence interval for each DSC characteristic that was systematically <2 % of the measured value.
The content of charcoal C was estimated from DSC thermograms using the method described by Hardy et al. (2017a), based on the relative height of the peaks derived from the combustion of charcoal and of that from the combustion of uncharred SOM (Leifeld, 2007). For acidic forest soils, only one peak was systematically discernible for charcoal and was, therefore, used for quantification. In contrast, in cropland and Ca-rich forest soils, three peaks were clearly identifiable for charcoal and used for quantification. The relationship between the thermal signature of charcoal and soil environmental conditions was attributed to the presence of abundant Ca2+ adsorbed to carboxylate groups at the surface of aged charcoal under neutral pH conditions, which decreases the thermal resistance of the O-rich, aged fraction of charcoal (Hardy et al., 2017b).
2.5 Sensitivity analysis
To test the influence of the pattern of heat fluxes related to the combustion of charcoal on the estimation of charcoal-C content, we mathematically simulated soil–charcoal mixtures (n=18) over a representative range of charcoal-C concentrations (from 5 % to 90 % of TOC) based on the DSC pattern of heat release from nine pre-industrial charcoals from different kiln sites. Simulated mixtures were obtained by adding the thermogram of pure charcoals, at different doses, to the thermogram of a charcoal-free soil. By comparison of predicted values with calculated values, we obtained a root mean square error (RMSE) of 1.39 % of the amount of charcoal C added.
2.6 BPCA analyses
Prior to analysis, samples were dried at 40 ∘C to a constant weight and sieved to 2 mm. Benzene polycarboxylic acids were extracted from the 45 soils from Germany as specific markers for BC in soil, according to the procedure of Brodowski et al. (2005). An estimation of total BC content was obtained by multiplying the total BPCA-C content by 2.27 (Brodowski et al., 2005).
3.1 Thermal analysis of soils
Irrespective of soil conditions, reference soils had a characteristic DSC pattern, with a main maximum between 300 and 330 ∘C, at 310.3±10.0 (mean ± SD) ∘C on average for forest reference soils and at 319.2±3.7 ∘C for cropland reference soils (Fig. 2). This peak was asymmetrical and spread systematically towards higher temperatures. A smaller peak was sometimes visible in the range of 400–450 ∘C, particularly in cropland reference soils (Fig. 2).
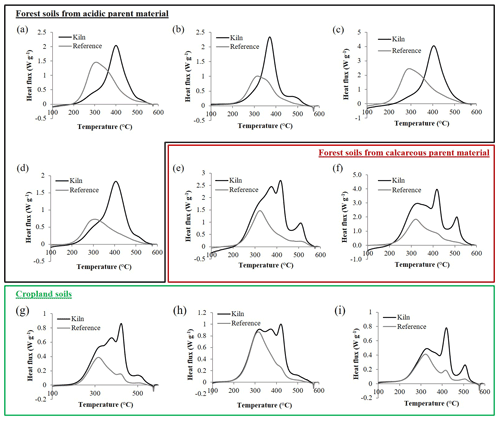
Figure 2Differential scanning calorimetry thermograms of a representative selection of soils from pre-industrial charcoal kiln sites (black curves) and adjacent reference soils (grey curves). Four sites were located on (very) acidic forest soil (a–d), two sites were located on calcareous forest soil (e–f) and three sites were located on cropland soils (g–i).
Soils from pre-industrial charcoal kiln sites had a more variable signature. In addition to the signal in the 300–330 ∘C range, they had from one to three additional exothermic peaks of higher thermal stability (Fig. 2). We observed two main types of DSC signature for kiln soils. In the first category, (very) acidic forest soils were pooled (Fig. 2a–d). These soils showed a characteristic main exothermic peak at 391.8±14.7 ∘C and a small peak of higher thermal stability at around 494.8±19.2 ∘C that was not always clearly visible. The second category comprised calcareous forest soils (Fig. 2e, f) and cropland soils (Fig. 2g–i). The main difference from the thermal pattern of forest soils of the first group was the presence of multiple peaks, with an exothermic peak at 374.7±6.3 ∘C and another at 422.6±2.7 ∘C instead of a single peak at around 400 ∘C. Regardless of the presence of charcoal, most thermograms had a small, sharp endothermic peak at about 575 ∘C.
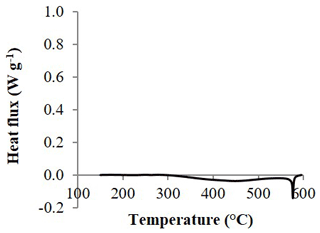
Figure 3Differential scanning calorimetry thermograms of the subsoil (argic horizon) of a haplic Luvisol, H2O2 treated.
The deep argic horizons that were H2O2 treated and, therefore, contained almost no residual organic carbon (OC content <0.91 g kg−1) showed very limited heat fluxes under DSC analysis (Fig. 3). In contrast to organo-mineral soils, no heat was released, and a small endothermic peak was even recorded between 350 and 600 ∘C, in addition to the same sharp endothermic peak at ∼575 ∘C as observed in most soils. This result suggests that, for the soils of this study, soil minerals have minor effects on the DSC signature. Accordingly, regression of total heat of reaction against TOC content provided a very high determination coefficient (R2≥0.97), regardless of the dataset (Fig. 4a–c), which highlighted the close relationship between heat released during DSC analysis and the combustion of soil organic materials. Small intercepts of the linear regressions may express some influence of mineralogy on heat fluxes.
3.2 Thermal analysis of charcoals
When the DSC thermogram of charcoal particles is superimposed on that of the kiln soil from which particles were extracted, it is clear that peaks recorded at temperatures higher than 350 ∘C were related to the combustion of charcoal (Fig. 5a). However, as suggested by the variability in the thermal signature of kiln soils, the pattern of heat release of pre-industrial charcoals varied to some extent. From one to three main peaks were visible on the thermograms of pure charcoals (Fig. 5b). The least stable peak (Peak 1) showed the highest variability, with temperatures ranging from 360.5 to 415.6 ∘C and an average value of 378.1±15.6 ∘C (mean ± SD). The temperature of the second and third peaks was less variable, with average values of 424.4±3.0 and 506.6±9.2 ∘C respectively.
The pattern of heat release of pre-industrial charcoals was also compared to that of birch charcoal that had never been aged in soil, produced in a traditional mound kiln (Fig. 5c). It is interesting to note that fresh charcoal had a thermal signature very different from that of pre-industrial charcoals. Fresh charcoal had a main peak at 477 ∘C with a shoulder at 329 ∘C, which was lower in temperature than the temperature of the lowest maxima recorded in pre-industrial charcoals. Additionally, the T50 of the fresh charcoal was 438 ∘C compared with that of the pre-industrial charcoals, which ranged from 388 to 418 ∘C with average values of 400.3±7.9 ∘C.
3.3 Quantification of charcoal C in soil with DSC and comparison with BPCA-C content
Data describing the selection of 45 soil samples from Germany used for the methodological comparison between the quantification of BC content by DSC and by BPCA molecular markers are presented in the Appendix. By means of the quantitative analysis of the DSC thermograms based on relative heights of peaks attributed to charcoal and uncharred SOM, we estimated a charcoal-C content of 13.5±7.8 g kg−1 for kiln soils in cropland and 0.9±0.7 g kg−1 for the respective reference soils. In forest soil samples, charcoal contents estimated with DSC were much higher, as the sites had never been diluted laterally by tillage. Forest kiln soil samples from Belgium contained 68.7±35.3 g kg−1 of charcoal C on average and up to 173.2 g kg−1; in the kiln soil samples from Germany, in comparison, the charcoal-C content was 79.7±68.9 g kg−1 on average and up to 199.7 g kg−1.
The thermal characteristics of the soils from Germany were compared to their total BPCA-C content. We found a strong positive correlation (r=0.935) between T50 and the total amount of BPCA C in soil (Fig. 6). However, the BPCA-C content recovered for the forest kiln soils from Germany of 13.2±10.8 g kg−1 was far lower than the charcoal-C content estimated by DSC of 79.7±68.9 g kg−1. After multiplication by the conversion factor of 2.27, the total BC content estimated from BPCA was 30.3±24.4 g kg−1, which was still less than half of the charcoal-C content estimated by DSC. However, the two variables were strongly correlated. A significant linear relationship between DSC-estimated charcoal-C content and the BPCA-C content recovered from the soil was found, both expressed as a fraction of the TOC content (Fig. 7a) or in absolute terms (Fig. 7b), with coefficients of determination of 0.89 and 0.97 respectively. The slope of the regression lines (Fig. 7a, b) showed that the total BPCA-C content underestimated the amount of charcoal C predicted by DSC by a factor of around 5. Accordingly, the BPCA-C : charcoal-C ratio was 0.18±0.03 on average.
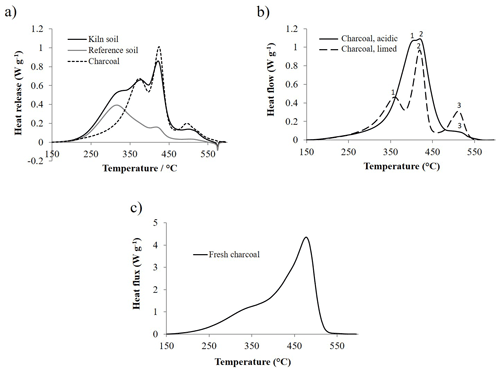
Figure 5Differential scanning calorimetry thermograms of several charcoal samples: (a) thermogram of charcoal particles superimposed on the thermogram of the soil of the pre-industrial charcoal kiln site from which particles were extracted and on the thermogram of adjacent reference soil (adapted from Hardy et al., 2017a); (b) thermograms of charcoals extracted from an acidic forest soil and from a limed cropland soil. Numbers on the graph identify the maxima of exothermic peaks specific to the combustion of charcoal; (c) thermogram of birch charcoal that was not aged in soil (produced in a traditional mound kiln).
4.1 Thermal analysis of soils
For the soils of this study, the strong correlation between total heat release measured by DSC and the TOC content supports the idea that the mineral background of soil has little effect on the shape of exothermic peaks from the combustion of SOM. Therefore, in the present case, exothermic peaks can be directly related to reactions involving the combustion of organic components of soil. However, in other soils, the mineralogy may interfere strongly with exothermic peaks from SOM combustion. In addition to the inversion of quartz-α to quartz-β at 573 ∘C (visible on most thermograms in Fig. 2), gibbsite, kaolinite and halloysite generate endothermic peaks between 300 and 550 ∘C (Tan et al., 1986). These minerals, which were either absent or present in relatively small amounts in the temperate soils of this study, are expected to be present in large amounts in many clay-rich tropical soils (Uehara and Gillman, 1981). However, the direct measurement of CO2 emissions by evolved gas analysis rather than heat fluxes recorded by DSC, alone or in combination with thermogravimetry, has the potential to eliminate most interference from soil minerals (Peltre et al., 2013) and to generalize the use of dynamic thermal analysis for the characterization and quantification of SOM pools.
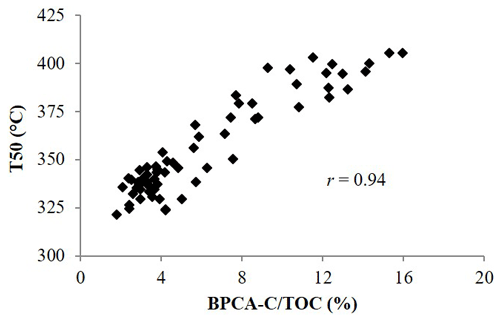
Figure 6Temperature of 50 % heat release of bulk soils from Germany against the fraction of C from BPCA (denoted as BPCA-C/TOC in the figure).
Thermal analysis of charcoal-rich kiln soils, adjacent reference soils and individual charcoals has highlighted that both charcoal and uncharred SOM are composed of a continuum of materials. Reference soils are dominated by thermally labile uncharred SOM compounds that degrade at around 300–330 ∘C. The spreading of the thermograms towards higher temperatures (Fig. 2) suggests that more stable compounds are also present in smaller amounts. The degradation of SOM in the 300–350 ∘C range has been related to the decomposition of aliphatic C molecules such as carbohydrates (Dell'Abate et al., 2002; Kucerík et al., 2004), whereas it has been related to the combustion of aromatic C in the 400–450 ∘C range (Satoh, 1984). Accordingly, Li et al. (2002) reported that the thermal stability of lignin is higher than that of cellulose but depends on its structure. More recently, Sanderman and Grandy (2020) confirmed (by combining thermal analysis and analytical pyrolysis of molecules released in different thermal windows) that polysaccharides and lipids are thermally more labile, whereas higher-temperature volatiles comprise phenols, aromatics, and N-containing compounds. Therefore, the pattern of heat release of reference soils suggests that they are composed mainly of aliphatic C from microbial residues as well as cellulose- and hemicellulose-derived SOM, mixed with a small amount of aromatic C that decomposes at higher temperature. The small differences in the shape of the thermograms from cropland and forest reference soils probably result from a difference in the composition of SOM, possibly related to the varying quality of organic matter inputs. Reference cropland soils systematically showed a small peak at ∼400 ∘C, which corresponds to the temperature of the main peak attributed to charcoal in kiln soil. By visual inspection of bulk soils, black particles that looked like charcoal were identified in each of them. These particles may result from (i) contamination of charcoal from the kiln site or (ii) burning that generally followed deforestation when land was converted from forest to agricultural land in the past (Hoyois, 1953). Moreover, plant residues left on the field after the harvest were commonly burnt until the 1980s (personal communication from Joseph Dufey, 2015).
4.2 Thermal analysis of charcoals
The properties and composition of charcoal are known to depend largely on the production conditions, such as the temperature and heating rate, that control the degree of aromaticity and crystallinity of chars (Keiluweit et al., 2010). Accordingly, the high thermal stability of BC has been attributed to its polycondensed aromatic structure (De la Rosa et al., 2008). Consistently, the content of aromatic C estimated by 13C nuclear magnetic resonance spectroscopy correlates positively with the proportion of thermally refractory SOM (Harvey et al., 2012; Leifeld, 2007). The thermal stability of aromatic compounds, which is greater than that of aliphatic compounds or O- and H-rich C functionalities (Leifeld, 2007), is consistent with the binding energy of C=C bonds (520 kJ mol−1) higher than that of C−C, C−O or C−H bonds (350–412 kJ mol−1) (Plante et al., 2009). However, both the degree of aromatic condensation and the presence of crystalline structures (Wiedemeier et al., 2015b) may control thermal resistance in addition to aromaticity. Leifeld (2007) showed that hexane soot, charred wood and charred rice straw had the same aromaticity but different thermal stabilities. At comparable aromaticity, thermal resistance depends mainly on the degree of aromatic condensation of char (Harvey et al., 2012; Leifeld, 2007) and can be further influenced by other factors such as ash content (McBeath et al., 2015).
The temperature of the thermally most stable peak has been proposed as the most reliable feature to assess the thermal stability of charcoal (Leifeld, 2007). Leifeld (2007) showed that the thermal stability of pine wood charred under N2 increases with charring temperature, and he recorded a complete loss of thermally labile compounds at 400 ∘C, in line with the process of aromatization of charcoal that occurs in the 280–400 ∘C range (Antal and Grønli, 2003; Bird et al., 2015). Leifeld (2007) also found that the most stable peak of charcoals and charred plant biomass occurred at a temperature >500 ∘C. In contrast, fresh charcoal in this study had a main maximum at 477 ∘C and exhibited a signal in the low range of temperatures (<350 ∘C). These discrepancies may be explained by (i) differences in the quality of charcoal and (ii) differences in the experimental parameters of the DSC analysis. Leifeld (2007) analysed his samples with a heating rate of 20 ∘C min−1, whereas we used a heating rate of 10 ∘C min−1 in this study. The temperature of heat release is decreased by slower heating rates (Fernández et al., 2010; Leifeld, 2007), which may explain the lower temperature of the most stable peak measured for our fresh charcoal. On the other hand, the survival of thermally labile compounds in charcoal produced in a traditional mound kiln suggests that the temperature of charring was <400 ∘C. This is not surprising, as wood pyrolysis by the traditional mound kiln method is expected to reach a maximum of 400–450 ∘C (Emrich, 1985), and the local maximum temperature of pyrolysis varies within the mound according to the distance from the hearth. In contrast, no signal was recorded in the lower range of temperatures (<350 ∘C) for pre-industrial charcoals. The presence of aliphatic C compounds, such as proteins and sugars, was detected in chars produced at low temperature and was related to the biological accessibility of chars, with the amount of labile compounds decreasing with the temperature of pyrolysis (Fabbri et al., 2012). The disappearance of the thermally labile fraction for pre-industrial charcoals aged in soil suggests that this fraction was biologically reactive and probably made of residual incompletely transformed organic molecules dominated by aliphatic C. Therefore, it was more subject to (a)biotic decomposition than the thermally stable fraction of charcoal and had become completely degraded since the time of charcoal production (over 150 years ago). Similarly, the presence of a labile fraction in engineered biochars has been found to contribute to early emissions of CO2 after introduction into soil (Sagrilo et al., 2014).
Interestingly, the thermal signature of fresh and aged charcoals is quite different. Aged charcoals are thermally less stable than fresh charcoals and generally have multiple exothermic peaks in the 360–525 ∘C range. The main process in ageing of charcoal is oxygenation, starting from the surface and propagating to the core of the particle (Lehmann et al., 2005). Charcoal was produced at relatively low temperature at kiln sites (400–450 ∘C; Emrich, 1985) and, therefore, may contain small aliphatic C and amorphous aromatic clusters that would not be recovered as BC by the majority of existing BC quantification procedures. Moreover, physical, chemical and biological weathering occurring over time in soil is supposed to decrease the stability of charcoal (Ascough et al., 2011) and create H- and O-rich C functionalities (Cheng et al., 2008; Hardy et al., 2017b; Lehmann et al., 2005) that have a decreased resistance to thermal oxidation. By relating thermal characteristics to the elemental composition of charcoal, Hardy et al. (2017b) highlighted that the O-rich fraction of charcoal has a specific thermal signature, corresponding to the peak of least thermal stability of charcoal (Peak 1, Fig. 5b). This is in line with the lower binding energy of C−O bonds compared with C=C bonds of aromatic clusters in charcoal (Plante et al., 2009). As a result of weathering, the overall thermal stability of aged charcoal is lower than that of fresh charcoal. Hardy et al. (2017b) also found that the temperature of Peak 1 was strongly negatively correlated with the content of Ca in charcoal. The most abundant O-rich functional groups in aged charcoals are carboxyl groups (Hardy et al., 2017b; Lehmann et al., 2005; Mao et al., 2012). These are known to have a strong affinity with Ca2+ (Kalinichev and Kirkpatrick, 2007). The presence of Ca2+ adsorbed to (poly-)carboxylate groups of charcoal may catalyse thermal decomposition by decreasing the binding energy of C−O bonds (e.g. Hu et al., 2018). Similarly, the presence of Al and Fe in the form of trivalent cations complexed to humic compounds of Podzols has been shown to alter the thermal stability of SOM (Schnitzer et al., 1964). This highlights the importance of soil conditions for the thermal signature of BC and, more generally, SOM.
Transmission electron micrographs of modern and fossil charcoals have provided evidence of organized and disorganized domains in the matrix of charcoal (Cohen-Ofri et al., 2006). The degree of organization, or aromatic condensation (McBeath and Smernik, 2009; Wiedemeier et al., 2015a), refers to the size and arrangement of aromatic clusters in BC, which increases when the temperature of pyrolysis is >350 ∘C, once the aromatization of BC is complete or nearly complete (Keiluweit et al., 2010; McBeath et al., 2015). Bird et al. (2015) proposed the existence of three pools of different resistance (labile C, semi-labile aromatic C and stable aromatic polycyclic C) to explain the reactivity of BC. According to the conceptual model of Bird et al. (2015), labile C corresponds to the fraction of BC that is composed of minor pyrolysis products such as anhydrosugars and methoxylated phenols, which are mineralizable in the (very) short term; semi-labile aromatic C corresponds to aromatic C with a low degree of aromatic condensation; and stable aromatic polycyclic C corresponds to polycyclic aromatic clusters with a ring size >7. Unfortunately, this view of charcoal stability, made of three pools of distinct chemistry and reactivity, cannot be directly translated to the presence of three exothermic peaks measured in the aged charcoals of this study, as the fresh and aged charcoals in this study were produced at a comparable temperature of about 400 ∘C (and were, therefore, both thought to be dominated by amorphous aromatic C). However, they had very contrasting DSC patterns of heat release. Thus, we hypothesize that the thermal resistance of charcoal may rather be controlled by (1) the binding energy of C bonds, which is driven by both pyrolysis conditions (aromaticity and aromatic condensation) and the degree of weathering (oxygenation and hydrogenation) of charcoal; and (2) the accessibility to combustion of C moieties, or the activation energy necessary to initiate combustion. As the reaction is partially surface controlled, it is expected that highly weathered outer surfaces of aged charcoal will oxidize more readily at lower temperatures, whereas the inner parts have no access to O2 despite the temperature being high enough for reaction. In contrast, an onion-shaped soot particle can only be oxidized layer by layer, and it probably requires a higher activation energy to initiate combustion. According to this view, the DSC peaks of contrasting thermal resistance found in aged charcoals may correspond to (i) C bonds that are weakened by the presence of O in their direct proximity (first peak, less thermally stable), (ii) aromatic C in small clusters that are partially weathered but that are not directly bound to oxygen (second peak), and (iii) unweathered aromatic C in large clusters (third peak, high thermal resistance). The process of BC ageing in the environment is still incompletely understood and is of prime importance to unravelling the role that BC plays in geochemical cycles. In that sense, dynamic thermal analysis has the potential to offer rapid, inexpensive continuous information on the complete BC continuum related to the binding energy of C bonds, which may provide useful new information on the degree of weathering of BC aged in soil.
4.3 Quantification of charcoal C by DSC: advantages and limitations
Thermal analysis of charcoal-rich kiln soils, adjacent reference soils and individual charcoals has highlighted that both charcoal and uncharred SOM are composed of a continuum of materials. These two continuums largely overlap, which stresses the issue of BC quantification in soil by static thermal methods: the choice of a cut-off temperature to differentiate quantitatively between charcoal C and uncharred SOC is not reliable. Therefore, the relative heights of peaks attributed either to charcoal or SOM were used here to quantify charcoal C in the soil of pre-industrial kiln sites. For cropland soils, the DSC-derived content of charcoal C stored in the topsoil of kiln sites correlated strongly (r=0.98) with the excess of OC (ΔOC) accumulated in the kiln soil relative to adjacent reference soil, as shown by Hardy et al. (2017a). The slope of the relationship between the two variables is 0.80. Using a similar approach for forest soils from Germany, we obtained a slope of 1.0 for the regression line between ΔOC and charcoal-C content estimated by DSC (r=0.94). For forest soils from Belgium, sampling by soil subhorizons of varying depth prevented us from making a comparison between charcoal-C content and ΔOC. The consistency between estimates of charcoal-C content by DSC and ΔOC provided confidence about the reliability of our DSC quantification procedure. However, the slope of 1 for forest soils from Germany supports the view that ΔOC consists exclusively of charcoal C, whereas the slope of ∼0.8 for cropland soils from Belgium indicates that only ∼80 % of ΔOC is charcoal C. This result suggests that the presence of aged charcoal had promoted the stabilization of a small amount of extra uncharred SOM in the cropland soils from Belgium, which is consistent with other findings on similar sites (Burgeon et al., 2021; Hernandez-Soriano et al., 2015; Kerré et al., 2016). The fact that this increase in uncharred SOM was not found in forest soils from Germany may be due to factors such as (i) the younger age (>60 years) of the sites, (ii) a varying effect of charcoal on biomass production or the dynamics of natural SOM depending on soil conditions, and (iii) the effect of repeated tillage over time in cropland that has diluted charcoal laterally (Hardy et al., 2017a) and may have accelerated the reconstitution of the natural SOM pool and its incorporation into soil.
Although the relationship between BPCA C and DSC-derived charcoal C was strongly linear (Fig. 7), the content of charcoal C obtained by DSC was more than 5 times higher than the content of BPCA C in soil. This result is in line with the findings of Brodowski et al. (2005), who stated that BPCA C may underestimate total charcoal-C content by a factor of up to 4.5 or higher, either due to the complete digestion of the less-condensed moieties of char or to the inaccessibility of the most refractory, condensed moieties (Brodowski et al., 2005; Glaser et al., 1998). This confirms, for the charcoal kiln soils of this study, that the 2.27 multiplicative factor for the estimation of BC content from BPCA C is overly conservative. Overall, this result reminds us that the quantitative interpretation of operationally defined BC measurements, regardless of the method, must be done with great caution and must be presented as an absolute value only if the recovery rate is controlled, which is very complicated in practice for most field case studies. Another source of uncertainty comes from DSC, particularly because of the variability in the shape of soil thermograms according to mineralogy and SOM quality. The shape of thermograms from pre-industrial charcoals did not seem to have much effect on the estimates, given that we obtained very accurate estimates (RMSE = 1.39 %) of charcoal-C content with our peak index for soil–charcoal mixtures numerically simulated from the thermograms of nine different pre-industrial charcoals with different shapes. However, we were unable to test how the variability in thermal properties of uncharred SOM affects the accuracy of the estimation because of the difficulty involved with finding soils completely free of BC. This point should be addressed in the future by setting up a strong calibration and validation dataset, which could be achieved by adding known amounts of charcoal to a variety of soils initially free of charcoal (or to artificial mixtures of minerals and SOM), as done by studies such as Hammes et al. (2007) or Roth et al. (2012) for other methods of BC quantification. To overcome the issue raised by the variability in both SOM and BC depending on their composition and interactions with soil minerals, further improvement of the method could be made possible by using peak decomposition of thermograms (Plante et al., 2005). This approach would require the identification of the thermal patterns associated with the different C moieties in SOM and BC and the modelling of the shape of each associated exothermic peak in order to decompose the thermograms on a rational basis.
The main advantage of dynamic thermal analysis for characterizing SOM comes from the fact that it provides a complete view of the continuum of organic materials present in soil. DSC analysis of the soil of pre-industrial charcoal kiln sites and adjacent charcoal-unaffected soils has stressed the complexity of BC quantification by highlighting the fact that the thermal properties of charcoal and uncharred SOM are variable and overlap to a large extent, invalidating the use of cut-off values for accurate differentiation between aged charcoal and uncharred SOM. Thermal analysis by DSC was found to be a very useful tool for identifying and characterizing charcoal in the soil. Aged charcoal was shown to have a characteristic thermal signature, generally remaining more thermally resistant than uncharred SOM despite the decrease in thermal stability due to ageing in soil. This thermal pattern was successfully used to quantify charcoal C in cropland and forest soils. We found a strong linear relationship (R2=0.97) between the DSC-derived charcoal-C content and BPCA-C content in the soils of this study, with BPCA C representing about one-fifth of DSC-derived charcoal C. Despite the successful use of DSC to quantify charcoal C in the soils of this study, our approach, based on peak height and position, cannot be generalized because of the high variability in the pattern of heat release of BC and SOM depending on their composition. The use of peak decomposition may help to overcome this issue, while the use of evolved gas analysis rather than DSC has the potential to get rid of the interference of soil minerals with exothermic peaks from SOM combustion. Overall, we believe that the potential of dynamic thermal analysis for characterizing and quantifying soil organic materials is largely under-exploited, despite the fact that it provides information on the whole range of organic materials present in soil. With a view to amending soil with biochar on a large scale in order to mitigate climate change, dynamic thermal analysis could be a very useful tool to assess biochar stability prior to application and to quantitatively and qualitatively trace its evolution in soil.
Data describing the selection of 45 soil samples from Germany used for the methodological comparison between the quantification of BC content by DSC and by BPCA molecular markers are presented in the following table.
Table A1TOC content, PLFA (phospholipid fatty acid) C content and DSC-derived charcoal-C content for the selection of 45 soil samples from Germany.
The data used for method comparison between DSC and BPCA are presented in Appendix A. The chemical properties of soils (https://doi.org/10.1111/ejss.12324, https://doi.org/10.1111/ejss.12395, Hardy et al., 2016, 2017a) and charcoals (https://doi.org/10.1016/j.orggeochem.2017.02.008, Hardy et al., 2017b) have been presented as supporting information in previous studies. To access raw DSC data, please contact the corresponding author.
BH characterized soil samples from Belgium, and NB characterized soil samples from Germany. BH and JL ran DSC analyses, made the calculations from the DSC graphs and interpreted DSC data. NB ran BPCA analyses for the selection of samples from Germany. BH drafted the text, and JL and NB revised the text.
The contact author has declared that neither they nor their co-authors have any competing interests.
Publisher's note: Copernicus Publications remains neutral with regard to jurisdictional claims in published maps and institutional affiliations.
The authors are grateful to Alain Plante from the University of Pennsylvania for thermogravimetry, DSC and evolved gas analysis preliminary tests made on a selection of samples and to Robin Giger and Roman Hüppi from Agroscope, Zurich, for their indispensable help with DSC and elemental analyses.
This research has been supported by the Direction Générale Opérationnelle Agriculture, Ressources Naturelles et Environnement du Service Public de Wallonie (grant no. 11/13342) and the Fonds Spéciaux de Recherche, Université Catholique de Louvain (grant no. 1C.31200.007). BPCA analyses were part of a study funded by the German Federal Institute for Geosciences and Natural Resources (BGR).
This paper was edited by Raphael Viscarra Rossel and reviewed by Alexander Bonhage and two anonymous referees.
Antal, M. J. and Grønli, M.: The Art, Science, and Technology of Charcoal Production, Ind. Eng. Chem. Res., 42, 1619–1640, https://doi.org/10.1021/ie0207919, 2003.
Ascough, P. L., Bird, M. I., Francis, S. M., Thornton, B., Midwood, A. J., Scott, A. C., and Apperley, D.: Variability in oxidative degradation of charcoal: Influence of production conditions and environmental exposure, Geochim. Cosmochim. Ac., 75, 2361–2378, https://doi.org/10.1016/j.gca.2011.02.002, 2011.
Bird, M. I., Wynn, J. G., Saiz, G., Wurster, C. M., and McBeath, A.: The Pyrogenic Carbon Cycle, Annu. Rev. Earth Planet. Sci., 43, 273–298, https://doi.org/10.1146/annurev-earth-060614-105038, 2015.
Borchard, N., Ladd, B., Eschemann, S., Hegenberg, D., Möseler, B. M., and Amelung, W.: Black carbon and soil properties at historical charcoal production sites in Germany, Geoderma, 232, 236–242, https://doi.org/10.1016/j.geoderma.2014.05.007, 2014.
Brodowski, S., Rodionov, A., Haumaier, L., Glaser, B., and Amelung, W.: Revised black carbon assessment using benzene polycarboxylic acids, Org. Geochem., 36, 1299–1310, 2005.
Burgeon, V., Fouché, J., Leifeld, J., Chenu, C., and Cornélis, J. T.: Organo-mineral associations largely contribute to the stabilization of century-old pyrogenic organic matter in cropland soils, Geoderma, 388, 114841, https://doi.org/10.1016/j.geoderma.2020.114841, 2021.
Cheng, C.-H., Lehmann, J., and Engelhard, M. H.: Natural oxidation of black carbon in soils: Changes in molecular form and surface charge along a climosequence, Geochim. Cosmochim. Ac., 72, 1598–1610, https://doi.org/10.1016/j.gca.2008.01.010, 2008.
Cohen-Ofri, I., Weiner, L., Boaretto, E., Mintz, G., and Weiner, S.: Modern and fossil charcoal: aspects of structure and diagenesis, J. Archaeol. Sci., 33, 428–439, https://doi.org/10.1016/j.jas.2005.08.008, 2006.
Czimczik, C. I. and Masiello, C. A.: Controls on black carbon storage in soils, Global Biogeochem. Cy., 21, GB3005, https://doi.org/10.1029/2006GB002798, 2007.
De la Rosa, J. M., Knicker, H., López-Capel, E., Manning, D. A. C., González-Perez, J. A., and González-Vila, F. J.: Direct Detection of Black Carbon in Soils by Py-GC/MS, Carbon-13 NMR Spectroscopy and Thermogravimetric Techniques, Soil Sci. Soc. Am. J., 72, 258, https://doi.org/10.2136/sssaj2007.0031, 2008.
Dehkordi, R. H., Burgeon, V., Fouche, J., Gomez, E. P., Cornelis, J. T., Nguyen, F., Denis, A., and Meersmans, J.: Using UAV collected RGB and multispectral images to evaluate winter wheat performance across a site characterized by century-old biochar patches in Belgium, Remote Sens., 12, 2504, https://doi.org/10.3390/RS12152504, 2020.
Dell'Abate, M. T., Benedetti, A., Trinchera, A., and Dazzi, C.: Humic substances along the profile of two Typic Haploxerert, Geoderma, 107, 281–296, https://doi.org/10.1016/S0016-7061(01)00153-7, 2002.
Elmquist, M., Gustafsson, O., and Andersson, P.: Quantification of sedimentary black carbon using the chemothermal oxidation method: an evaluation of ex situ pretreatments and standard additions approaches, Limnol. Oceanogr.-Meth., 2, 417–427, 2004.
Emrich, W.: Handbook of Charcoal Making, D. Reidel Publishing Company, Dordrecht, The Netherlands, ISBN 978-90-481-8411-8, https://doi.org/10.1007/978-94-017-0450-2, 1985.
Evrard, R.: Forges anciennes, Editions Solédi, Liège, Belgium, 1956.
Fabbri, D., Torri, C., and Spokas, K. A.: Analytical pyrolysis of synthetic chars derived from biomass with potential agronomic application (biochar). Relationships with impacts on microbial carbon dioxide production, J. Anal. Appl. Pyrolysis, 93, 77–84, https://doi.org/10.1016/j.jaap.2011.09.012, 2012.
Fernández, J. M., Plante, A. F., Leifeld, J., and Rasmussen, C.: Methodological considerations for using thermal analysis in the characterization of soil organic matter, J. Therm. Anal. Calorim., 104, 389–398, https://doi.org/10.1007/s10973-010-1145-6, 2010.
Forbes, M. S., Raison, R. J., and Skjemstad, J. O.: Formation, transformation and transport of black carbon (charcoal) in terrestrial and aquatic ecosystems, Sci. Total Environ., 370, 190–206, https://doi.org/10.1016/j.scitotenv.2006.06.007, 2006.
Glaser, B. and Birk, J. J.: State of the scientific knowledge on properties and genesis of Anthropogenic Dark Earths in Central Amazonia (terra preta de Índio), Geochim. Cosmochim. Ac., 82, 39–51, https://doi.org/10.1016/j.gca.2010.11.029, 2012.
Glaser, B., Haumaier, L., Guggenberger, G., and Zech, W.: Black carbon in soils: the use of benzenecarboxylic acids as specific markers, Org. Geochem., 29, 811–819, 1998.
Glaser, B., Haumaier, L., Guggenberger, G., and Zech, W.: The “Terra Preta” phenomenon: a model for sustainable agriculture in the humid tropics, Naturwissenschaften, 88, 37–41, https://doi.org/10.1007/s001140000193, 2001.
Glaser, B., Lehmann, J., and Zech, W.: Ameliorating physical and chemical properties of highly weathered soils in the tropics with charcoal – a review, Biol. Fertil. Soils, 35, 219–230, https://doi.org/10.1007/s00374-002-0466-4, 2002.
Goldberg, E. D.: Black carbon in the environment, John Wiley & Sons, New York, ISBN 0471819794, 1985.
Gustafsson, Ö., Bucheli, T. D., Kukulska, Z., Andersson, M., Largeau, C., Rouzaud, J. N., Reddy, C. M., and Eglinton, T. I.: Evaluation of a protocol for the quantification of black carbon in sediments., Global Biogeochem. Cy., 15, 881–890, 2001.
Hammes, K., Schmidt, M. W. I., Smernik, R. J., Currie, L. A., Ball, W. P., Nguyen, T. H., Louchouarn, P., Houel, S., Gustafsson, Ö., Elmquist, M., Cornelissen, G., Skjemstad, J. O., Masiello, C. a., Song, J., Peng, P., Mitra, S., Dunn, J. C., Hatcher, P. G., Hockaday, W. C., Smith, D. M., Hartkopf-Fröder, C., Böhmer, A., Lüer, B., Huebert, B. J., Amelung, W., Brodowski, S., Huang, L., Zhang, W., Gschwend, P. M., Flores-Cervantes, D. X., Largeau, C., Rouzaud, J.-N., Rumpel, C., Guggenberger, G., Kaiser, K., Rodionov, A., Gonzalez-Vila, F. J., Gonzalez-Perez, J. A., De la Rosa, J. M., Manning, D. A. C., López-Capél, E., and Ding, L.: Comparison of quantification methods to measure fire-derived (black/elemental) carbon in soils and sediments using reference materials from soil, water, sediment and the atmosphere, Global Biogeochem. Cy., 21, GB3016, https://doi.org/10.1029/2006GB002914, 2007.
Hardy, B., Cornelis, J.-T., Houben, D., Lambert, R., and Dufey, J. E.: The effect of pre-industrial charcoal kilns on chemical properties of forest soil of Wallonia, Belgium, [data set], Eur. J. Soil Sci., 67, 206–216, https://doi.org/10.1111/ejss.12324, 2016.
Hardy, B., Cornelis, J.-T., Houben, D., Leifeld, J., Lambert, R., and Dufey, J.: Evaluation of the long-term effect of biochar on properties of temperate agricultural soil at pre-industrial charcoal kiln sites in Wallonia, Belgium, Eur. J. Soil Sci., 68, 80–89, https://doi.org/10.1111/ejss.12395, 2017a.
Hardy, B., Leifeld, J., Knicker, H., Dufey, J. E., Deforce, K., and Cornélis, J. T.: Long term change in chemical properties of preindustrial charcoal particles aged in forest and agricultural temperate soil, [data set], Org. Geochem., 107, 33–45, https://doi.org/10.1016/j.orggeochem.2017.02.008, 2017b.
Harvey, O. R., Kuo, L.-J., Zimmerman, A. R., Louchouarn, P., Amonette, J. E., and Herbert, B. E.: An index-based approach to assessing recalcitrance and soil carbon sequestration potential of engineered black carbons (biochars), Environ. Sci. Technol., 46, 1415–21, https://doi.org/10.1021/es2040398, 2012.
Hernandez-Soriano, M. C., Kerré, B., Goos, P., Hardy, B., Dufey, J., and Smolders, E.: Long-term effect of biochar on the stabilization of recent carbon: soils with historical inputs of charcoal, GCB Bioenergy, 8, 371–381, https://doi.org/10.1111/gcbb.12250, 2015.
Hirsch, F., Schneider, A., Bauriegel, A., Raab, A., and Raab, T.: Formation, classification, and properties of soils at two relict charcoal hearth sites in Brandenburg, Germany, Front. Environ. Sci., 6, https://doi.org/10.3389/fenvs.2018.00094, 2018.
Hoyois, G.: L'Ardenne et l'Ardennais, L'Evolution Economique et Sociale d'une région, Tome 2, Bruxelles-Paris: Éditions universitaires; Gembloux: J. Duculot, imprimeur-éditeur, 1953.
Hu, Y., Wang, Z., Cheng, X., Liu, M., and Ma, C.: Effects of catalysts on combustion characteristics and kinetics of coal-char blends, IOP Conf. Ser. Earth Environ. Sci., 133, 012023, https://doi.org/10.1088/1755-1315/133/1/012023, 2018.
IUSS Working Group WRB: World reference base for soil resources 2014, International soil classification system for naming soils and creating legends for soil maps, World Soil Resources Reports No 106, Food and Agriculture Organization of the United Nations, Rome, Italy, ISBN 978-92-5-108369-7, 2014.
Kalinichev, A. G. and Kirkpatrick, R. J.: Molecular dynamics simulation of cationic complexation with natural organic matter, Eur. J. Soil Sci., 58, 909–917, https://doi.org/10.1111/j.1365-2389.2007.00929.x, 2007.
Kappenberg, A., Bläsing, M., Lehndorff, E., and Amelung, W.: Black carbon assessment using benzene polycarboxylic acids: Limitations for organic-rich matrices, Org. Geochem., 94, 47–51, doi:doi:https://doi.org/10.1016/j.orggeochem, 2016.
Keiluweit, M., Nico, P. S., Johnson, M. G., and Kleber, M.: Dynamic molecular structure of plant biomass-derived black carbon (biochar), Environ. Sci. Technol., 44, 1247–53, https://doi.org/10.1021/es9031419, 2010.
Kerré, B., Bravo, C. T., Leifeld, J., Cornelissen, G., and Smolders, E.: Historical soil amendment with charcoal increases sequestration of non-charcoal carbon: a comparison among methods of black carbon quantification, Eur. J. Soil Sci., 67, 324–331, https://doi.org/10.1111/ejss.12338, 2016.
Kerré, B., Willaert, B., and Smolders, E.: Lower residue decomposition in historically charcoal-enriched soils is related to increased adsorption of organic matter, Soil Biol. Biochem., 104, 1–7, https://doi.org/10.1016/j.soilbio.2016.10.007, 2017.
Knicker, H.: Pyrogenic organic matter in soil: Its origin and occurrence, its chemistry and survival in soil environments, Quat. Int., 243, 251–263, https://doi.org/10.1016/j.quaint.2011.02.037, 2011.
Kucerík, J., Kovár, J., and Pekar, M.: Thermoanalytical investigation of lignite humic acids fractions, J. Therm. Anal. Calorim., 76, 55–65, https://doi.org/10.1023/B:JTAN.0000027803.24266.48, 2004.
Laird, D. A.: The charcoal vision: a win-win-win scenario for simultaneously producing bioenergy, permanently sequestering carbon, while improving soil and water quality, Agron. J., 100, 178–181, https://doi.org/10.2134/agronj2007.0161, 2008.
Lasota, J., Błońska, E., Babiak, T., Piaszczyk, W., Stępniewska, H., Jankowiak, R., Boroń, P., and Lenart-Boroń, A.: Effect of Charcoal on the Properties, Enzyme Activities and Microbial Diversity of Temperate Pine Forest Soils, Forests, 12, 1488, https://doi.org/10.3390/f12111488, 2021.
Lehmann, J.: Bio-energy in the black, Front. Ecol. Environ., 5, 381–387, 2007.
Lehmann, J., Liang, B., Solomon, D., Lerotic, M., Luizão, F., Kinyangi, J., Schäfer, T., Wirick, S., and Jacobsen, C.: Near-edge X-ray absorption fine structure (NEXAFS) spectroscopy for mapping nano-scale distribution of organic carbon forms in soil: Application to black carbon particles, Global Biogeochem. Cy., 19, GB1013, https://doi.org/10.1029/2004GB002435, 2005.
Leifeld, J.: Thermal stability of black carbon characterised by oxidative differential scanning calorimetry, Org. Geochem., 38, 112–127, https://doi.org/10.1016/j.orggeochem.2006.08.004, 2007.
Leifeld, J., Heiling, M., and Hajdas, I.: Age and thermal stability of particulate organic matter fractions indicate the presence of black carbon in soil, Radiocarbon, 57, 99–107, https://doi.org/10.2458/azu, 2015.
Leifeld, J., Alewell, C., Bader, C., Krüger, J. P., Mueller, C. W., Sommer, M., Steffens, M., and Szidat, S.: Pyrogenic Carbon Contributes Substantially to Carbon Storage in Intact and Degraded Northern Peatlands, L. Degrad. Dev., 29, 2082–2091, https://doi.org/10.1002/ldr.2812, 2018.
Li, J., Li, B., and Zhang, X. C.: Comparative studies of thermal degradation between Larch lignin and Manchurian Ash lignin, Polym. Degrad. Stab., 78, 279–285, 2002.
Liang, B., Lehmann, J., Sohi, S. P., Thies, J. E., O'Neill, B., Trujillo, L., Gaunt, J., Solomon, D., Grossman, J., Neves, E. G., and Luizão, F. J.: Black carbon affects the cycling of non-black carbon in soil, Org. Geochem., 41, 206–213, https://doi.org/10.1016/j.orggeochem.2009.09.007, 2010.
Mao, J.-D., Johnson, R. L., Lehmann, J., Olk, D. C., Neves, E. G., Thompson, M. L., and Schmidt-Rohr, K.: Abundant and stable char residues in soils: implications for soil fertility and carbon sequestration, Environ. Sci. Technol., 46, 9571–9576, https://doi.org/10.1021/es301107c, 2012.
Masiello, C. A.: New directions in black carbon organic geochemistry, Mar. Chem., 92, 201–213, https://doi.org/10.1016/j.marchem.2004.06.043, 2004.
Mastrolonardo, G., Calderaro, C., Cocozza, C., Hardy, B., Dufey, J., and Cornelis, J. T.: Long-term effect of charcoal accumulation in hearth soils on tree growth and nutrient cycling, Front. Environ. Sci., 7, 1–15, https://doi.org/10.3389/fenvs.2019.00051, 2019.
McBeath, A. V. and Smernik, R. J.: Variation in the degree of aromatic condensation of chars, Org. Geochem., 40, 1161–1168, https://doi.org/10.1016/j.orggeochem.2009.09.006, 2009.
McBeath, A. V., Wurster, C. M., and Bird, M. I.: Influence of feedstock properties and pyrolysis conditions on biochar carbon stability as determined by hydrogen pyrolysis, Biomass Bioener., 73, 155–173, https://doi.org/10.1016/j.biombioe.2014.12.022, 2015.
Mukherjee, S. and Kumar, M.: Cycling of black carbon and black nitrogen in the hydro-geosphere: Insights on the paradigm, pathway, and processes, Sci. Total Environ., 770, 144711, https://doi.org/10.1016/j.scitotenv.2020.144711., 2021.
Nguyen, T. H., Brown, R., and Ball, W. P.: An evaluation of thermal resistance as a measure of black carbon content in diesel soot, wood char, and sediment, Org. Geochem., 35, 217–234, https://doi.org/10.1016/j.orggeochem.2003.09.005, 2004.
Peltre, C., Fernández, J. M., Craine, J. M., and Plante, A. F.: Relationships between Biological and Thermal Indices of Soil Organic Matter Stability Differ with Soil Organic Carbon Level, Soil Sci. Soc. Am. J., 77, 2020, https://doi.org/10.2136/sssaj2013.02.0081, 2013.
Plante, a. F., Pernes, M., and Chenu, C.: Changes in clay-associated organic matter quality in a C depletion sequence as measured by differential thermal analyses, Geoderma, 129, 186–199, https://doi.org/10.1016/j.geoderma.2004.12.043, 2005.
Plante, A. F., Fernández, J. M., and Leifeld, J.: Application of thermal analysis techniques in soil science, Geoderma, 153, 1–10, https://doi.org/10.1016/j.geoderma.2009.08.016, 2009.
Plante, A. F., Fernández, J. M., Haddix, M. L., Steinweg, J. M., and Conant, R. T.: Biological, chemical and thermal indices of soil organic matter stability in four grassland soils, Soil Biol. Biochem., 43, 1051–1058, https://doi.org/10.1016/j.soilbio.2011.01.024, 2011.
Plante, A. F., Beaupré, S. R., Roberts, M. L., and Baisden, T.: Distribution of Radiocarbon Ages in Soil Organic Matter by Thermal Fractionation, Radiocarbon, 55, 1077–1083, https://doi.org/10.1017/s0033822200058215, 2013.
Pollet, S., Chabert, A., Burgeon, V., Cornélis, J.-T., Fouché, J., Gers, C., Hardy, B., and Pey, B.: Limited effects of century-old biochar on taxonomic and functional diversities of collembolan communities across land-uses, Soil Biol. Biochem., 164, 108484, https://doi.org/10.1016/j.soilbio.2021.108484, 2022.
Preston, C. M. and Schmidt, M. W. I.: Black (pyrogenic) carbon: a synthesis of current knowledge and uncertainties with special consideration of boreal regions, Biogeosciences, 3, 397–420, https://doi.org/10.5194/bg-3-397-2006, 2006.
Reisser, M., Purves, R. S., Schmidt, M. W. I., and Abiven, S.: Pyrogenic Carbon in Soils: A Literature-Based Inventory and a Global Estimation of Its Content in Soil Organic Carbon and Stocks, Front. Earth Sci., 4, 1–14, https://doi.org/10.3389/feart.2016.00080, 2016.
Roth, P. J., Lehndorff, E., Brodowski, S., Bornemann, L., Sanchez-García, L., Gustafsson, Ö., and Amelung, W.: Differentiation of charcoal, soot and diagenetic carbon in soil: Method comparison and perspectives, Org. Geochem., 46, 66–75, https://doi.org/10.1016/j.orggeochem.2012.01.012, 2012.
Sagrilo, E., Jeffery, S., Hoffland, E., and Kuyper, T. W.: Emission of CO2 from biochar-amended soils and implications for soil organic carbon, GCB Bioener., 7, 1294–1304, https://doi.org/10.1111/gcbb.12234, 2014.
Samojlik, T., Jȩdrzejewska, B., Michniewicz, M., Krasnodȩbski, D., Dulinicz, M., Olczak, H., Karczewski, A., and Rotherham, I. D.: Tree species used for low-intensity production of charcoal and wood-tar in the 18th-century Białowieza Primeval Forest, Poland, Phytocoenologia, 43, 1–12, https://doi.org/10.1127/0340-269X/2013/0043-0511, 2013.
Sanderman, J. and Grandy, A. S.: Ramped thermal analysis for isolating biologically meaningful soil organic matter fractions with distinct residence times, SOIL, 6, 131–144, https://doi.org/10.5194/soil-6-131-2020, 2020.
Santín, C., Doerr, S. H., Kane, E. S., Masiello, C. A., Ohlson, M., de la Rosa, J. M., Preston, C. M., and Dittmar, T.: Towards a global assessment of pyrogenic carbon from vegetation fires, Glob. Chang. Biol., 22, 76–91, https://doi.org/10.1111/gcb.12985, 2016.
Schenkel, Y., Bertaux, P., Vanwijnsberghe, S., and Carre, J.: An evaluation of the mound kiln carbonization technique, Biomass Bioenerg., 14, 505–516, 1998.
Schmidt, H. P., Kammann, C., Hagemann, N., Leifeld, J., Bucheli, T. D., Sánchez Monedero, M. A., and Cayuela, M. L.: Biochar in agriculture – A systematic review of 26 global meta-analyses, GCB Bioenerg., 13, 1708–1730, https://doi.org/10.1111/gcbb.12889, 2021.
Schmidt, M. W. I. and Noack, A. G.: Black carbon in soils and sediments: Analysis, distribution, implications, and current challenges, Global Biogeochem. Cy., 14, 777–793, https://doi.org/10.1029/1999GB001208, 2000.
Schmidt, M. W. I., Skjemstad, J. O., Czimczik, C. I., Glaser, B., Prentice, K. M., Gelinas, Y., and Kuhlbusch, T. A. J.: Comparative analysis of black carbon in soils, Global Biogeochem. Cy., 15, 163–167, 2001.
Schneider, A., Hirsch, F., Raab, A., and Raab, T.: Dye tracer visualization of infiltration patterns in soils on relict charcoal hearths, Front. Environ. Sci., 6, 1–14, https://doi.org/10.3389/fenvs.2018.00143, 2018.
Schneider, M. P. W., Hilf, M., Vogt, U. F., and Schmidt, M. W. I.: The benzene polycarboxylic acid (BPCA) pattern of wood pyrolyzed between 200 ∘C and 1000 ∘C, Org. Geochem., 41, 1082–1088, https://doi.org/10.1016/j.orggeochem.2010.07.001, 2010.
Schnitzer, M., Turner, R. C., and Hoffman, I.: A thermo-gravimetric study of organic matter of representative Canadian podzol soils, Can. J. Soil Sci., 44, 7–13, 1964.
Sherrod, L. A., Dunn, G., Peterson, G. A., and Kolberg, R. L.: Inorganic Carbon Analysis by Modified Pressure-Calcimeter Method, Soil Sci. Soc. Am. J., 66, 299–305, 2002.
Solomon, D., Lehmann, J., Thies, J., Schäfer, T., Liang, B., Kinyangi, J., Neves, E., Petersen, J., Luizão, F., and Skjemstad, J.: Molecular signature and sources of biochemical recalcitrance of organic C in Amazonian Dark Earths, Geochim. Cosmochim. Ac., 71, 2285–2298, https://doi.org/10.1016/j.gca.2007.02.014, 2007.
Tan, K. H., Hajek, B. F., and Barshad, I.: Thermal analysis techniques., in Methods of Soil Analysis, Part I. Physical and Mineralogical Methods, Agronomy Monograph, Vol. 9, edited by: Klute, A., American Society of Agronomy, Madison, WI Todor., 151–183, 1986.
Uehara, G. and Gillman, P. G.: The Mineralogy, Chemistry, and Physics of Tropical Soils with Variable Charge Clays, Editor: Boulder, C., Westview Press, Ann Arbor, MI, ISBN 0891584846, 1981.
Wang, J., Xiong, Z., and Kuzyakov, Y.: Biochar stability in soil: Meta-analysis of decomposition and priming effects, GCB Bioenerg., 8, 512–523, https://doi.org/10.1111/gcbb.12266, 2016.
Wiedemeier, D. B., Abiven, S., Hockaday, W. C., Keiluweit, M., Kleber, M., Masiello, C. A., McBeath, A. V., Nico, P. S., Pyle, L. A., Schneider, M. P. W., Smernik, R. J., Wiesenberg, G. L. B., and Schmidt, M. W. I.: Aromaticity and degree of aromatic condensation of char, Org. Geochem., 78, 135–143, https://doi.org/10.1016/j.orggeochem.2014.10.002, 2015a.
Wiedemeier, D. B., Brodowski, S., and Wiesenberg, G. L. B.: Pyrogenic molecular markers: Linking PAH with BPCA analysis, Chemosphere, 119, 432–437, https://doi.org/10.1016/j.chemosphere.2014.06.046, 2015b.
Wolf, M., Lehndorff, E., Wiesenberg, G. L. B., Stockhausen, M., Schwark, L., and Amelung, W.: Towards reconstruction of past fire regimes from geochemical analysis of charcoal, Org. Geochem., 55, 11–21, https://doi.org/10.1016/j.orggeochem.2012.11.002, 2013.
Zanutel, M., Garré, S., and Bielders, C. L.: Long-term effect of biochar on physical properties of agricultural soils with different textures at pre-industrial charcoal kiln sites in Wallonia (Belgium), Eur. J. Soil Sci., 73, 1–17, https://doi.org/10.1111/ejss.13157, 2021.