the Creative Commons Attribution 4.0 License.
the Creative Commons Attribution 4.0 License.
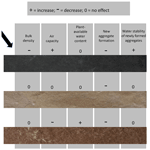
Polyester microplastic fibers affect soil physical properties and erosion as a function of soil type
Rosolino Ingraffia
Gaetano Amato
Vincenzo Bagarello
Francesco G. Carollo
Dario Giambalvo
Massimo Iovino
Anika Lehmann
Matthias C. Rillig
Alfonso S. Frenda
Microplastics are recognized as a factor of global change contaminating many environmental compartments. Agricultural soils are very likely to receive microplastic contamination and are of particular concern due to their role in food production. Microplastic fibers have already been shown to be able to affect soil properties, but their effect on different soil types is poorly understood. Moreover, limited information is available on how the presence of this pollutant can affect soil water erosion processes, which are extremely important issues in many environments. In the light of this, we performed two experiments (carried out on a microscale) to investigate how the presence of polyester microplastic fibers affects soil physical and hydrological parameters and processes such as aggregate formation and soil erosion in three different agricultural soil types (a Vertisol, an Entisol, and an Alfisol).
Our data show that the effects of polyester microplastic fibers on soil physical parameters and erosion are strongly dependent on soil type. We found that microplastic fiber contamination can affect soil bulk density, capacitive indicators of soil physical quality, and decrease the formation of new aggregates (labile in the incubation period applied in our experiments) but did not affect their stability in water. However, we found that polyester microplastic fibers reduced soil loss and sediment concentration, especially in the most erodible soils. In this paper, we provide some hypotheses, but certainly future data are still needed to confirm or disprove our hypotheses.
Overall, our results highlight the importance of broadly exploring soil properties, such as texture, mineralogy, organic carbon content, etc., to better understand how the various soil types respond to microplastic fiber contamination.
- Article
(7011 KB) - Full-text XML
-
Supplement
(153 KB) - BibTeX
- EndNote
Although the production of plastic is relatively recent (∼ 1950), its versatility and low cost have made it one of the most used daily materials today, and it is hard to think of a world without plastic. Global production of plastics has exceeded 350 × 106 t yr−1. Only a modest fraction of it is recycled (6 %–26 %; Alimi et al., 2018), while the vast majority generates plastic waste. Mishandling of plastic waste can lead to environmental contamination, and indeed, small plastic particles have been documented even in the most remote areas of the globe (Bergami et al., 2020; Napper et al., 2020). In the last decade, research on the environmental impacts of plastic has received growing attention, especially through the study of microplastics (defined as plastic particles smaller than 5 mm; Hartmann et al., 2019).
Most studies on plastic pollution concern aquatic environments, although most plastics are likely present in the terrestrial environment (estimated in quantities 4 to 23 times greater than those in the ocean; Horton et al., 2017). Given their role in food production, agricultural ecosystems are of particular concern. Microplastics (MPs) can reach agricultural soils through different routes: (i) incorporation of compost, sewage sludge, coatings of seeds and fertilizers, and other organic compounds often contaminated with MPs (Weithmann et al., 2018; Zubris and Richards, 2005; Zhang et al., 2020), (ii) fragmentation of plastic films used for mulching (Qi et al., 2020), or (iii) atmospheric fallout (Dris et al., 2018). Therefore, it seems clear that agricultural land contains MPs and, considering that the degradation of plastic in the soil is generally very slow (O'Kelly et al., 2021), the current hypothesis is that the MP content in agricultural land will slowly increase over time.
Once the MP particles reach the soil surface, they can be easily incorporated into the soil profile through terrestrial fauna (earthworms; Rillig et al., 2017a; Collembola: Maaß et al., 2017), biopores, mechanical operations (e.g., plowing), soil cracking (Rillig et al., 2017b), cryoturbation, etc. Incorporated MPs in the soil can interact with soil biophysical processes. Indeed, several studies have shown that the presence of MPs can affect soil physical characteristics, with the effects widely differentiated in relation to the soil characteristics and MP type, shape, size, and concentration (Boots et al., 2019; Lozano et al., 2021; Machado et al., 2018; Zhang et al., 2019). Among the various microplastic types, polyester MP fibers are one of the most detected in agroecosystems (Büks and Kaupenjohann, 2020; Crossman et al., 2020) and have been shown to be able to markedly modify (often stronger than other MP types) several soil physical properties. For instance, Machado et al. (2018) found that polyester MP fibers at a concentration of 0.4 % decreased soil bulk density and increased soil water holding capacity in an Albic Luvisol, while Lehmann et al. (2019), in the same soil type, found that this contaminant tends to decrease soil aggregate water stability when present at a concentration of 0.1 %.
As soil physical characteristics are related to its susceptibility to erosion (Bradford et al., 1987; Lowery et al., 1995; Mamedov and Levy, 2019), it is reasonable to expect that MPs in the soil can influence water erosion processes, which are extremely important issues in many environments (Bagarello et al., 2018). However, to the best of our knowledge, limited research has been conducted to evaluate if and to what extent MP contamination of the soil affects water erosion processes.
In light of the above, plastic likely represents a risk for the functionality and resilience of ecosystems. Moreover, due to erosion processes, microplastic particles embedded in the upper soil layer can be transported, eventually reaching water bodies such as rivers, lakes, or the sea (Rehm et al., 2021). To better manage this situation, it is necessary to understand the sources, movements, fate, and impacts of plastic when it reaches the different environmental compartments.
The main objective of this research is to expand the knowledge on the effects of MP contamination on the physical properties of three different soil types and evaluate the impact of polyester MP fibers on water erosion processes. The underlying hypotheses are as follows: (i) polyester MP fiber contamination negatively affects the structural state of the soil and increases soil erosion, and (ii) the adverse impact of polyester MP fiber contamination on physical soil properties and hydrological processes differs with soil type, with more marked effects on soils that are characterized by a low aggregation ability. In these soils, the presence of MP fibers could substantially increase the susceptibility to erosion processes. Collecting this information is essential for identifying the damages and developing solutions that can reduce the impact of microplastics on ecosystems and, at the same time, concentrate efforts in terms of resources and interventions in the most vulnerable areas.
2.1 Soils and polyester microplastic fiber treatment
For our experiment, we chose the following three different soils, which are widely spread in the Mediterranean area:
- i.
Typic Haploxerert (Vertisol). This soil type is widespread in areas with a flat or slightly sloping morphology. The sampled soil is well-structured, with a clay texture, good water and nutrient accessibility, sub-alkaline reaction, and fair or high presence of organic matter and other elements of fertility (P, K, N, etc). Smectite (montmorillonite) is the dominant clay mineral. This soil is characterized by large and deep cracks along the profile during the dry season. It has a medium–high production potential.
- ii.
Typic Xerorthent (Entisol). It is a light brown type of soil with a sub-alkaline reaction, widespread in areas with steep and uneven morphologies, frequently affected by erosion phenomena; it generally has a limited thickness with an abundant skeleton presence. It contains illite as the dominant clay mineral, followed by kaolinite. The structure is not very stable, the organic matter content is modest, and the production potential is medium–low.
- iii.
Typic Rhodoxeralfs (Alfisol). It is a typical soil widespread on the carbonate platforms of many Mediterranean environments; it can also be found in mountain contexts that are carbonated, and when located in high slopes, they are susceptible to severe erosion. The color tends to be red due to the considerable presence of iron oxides linked to the leached clays. Kaolinite is the dominant clay mineral. This soil is characterized by strong pedological aridity, due to its calcareous nature, and low amounts of organic matter and fertility elements.
Table 1Physical and chemical properties of the three different soils used in the experiment.
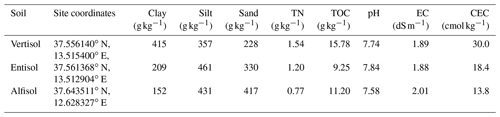
Clay, silt, and sand were classified according to the United States Department of Agriculture (USDA; clay < 2 µm, silt 2–50 µm, and sand 50–2000 µm). TN is total nitrogen, TOC is total organic carbon, EC is electrical conductivity, and CEC is cation exchange capacity.
The soils were sampled at the end of October 2019 from the upper 30 cm of agricultural fields. After sampling, the soil was air dried, sieved at 600 µm and stored at 4 ∘C until the beginning of the experiments in December 2019. This was to minimize changes in the natural microbial community. At the sampling time, we checked and ensured that the three soil types were not contaminated with meso- and/or macroplastic particles; however, we did not carry out analytical procedures to assay the contamination of smaller plastic particles, and therefore, we cannot exclude that the control treatments might already contain a detectable amount of micro- and/or nanoplastic particles.
Soils were characterized as follows: particle size distribution was determined using conventional methods, and soil texture was classified according to the United States Department of Agriculture (USDA; Gee and Bauder, 1986); total nitrogen (TN; Kjeldhal), total organic carbon (TOC; Walkley–Black procedure according to Nelson and Sommers, 1996), pH, saturated electrical conductivity at 25 ∘C (EC), and cation exchange capacity (CEC). Soil properties are listed in Table 1.
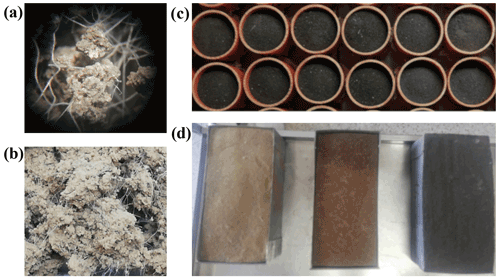
Figure 1(a, b) Contaminated soils. (c) Experimental units in experiment I. (d) Experimental units in experiment II.
For microplastic contamination, a 100 % polyester white rope (Marlow Ropes; Marlowbraid classic rope) was manually cut to produce secondary microplastic fibers. These fibers were characterized by scanning at least 200 fibers on PVC trays 10 times (Epson Perfection V800 scanner, 8 bit grayscale, 800 dpi) and then analyzing the scans with WinRHIZO (Pro v. 2007d software; Regent Instruments Inc., Quebec, Canada). The mean and the standard deviation, SD, of fiber length were 2.87 and 0.31 mm, respectively, and the mean and SD of fiber diameter were 87 and 3 µm, respectively. The polyester fibers were incorporated into the soil at a concentration of 0.5 % of the dry soil weight. This microplastic level was similar to that used in previous studies, which reported noticeable changes in the soil biophysical environment and plant response (Lehmann et al., 2020; Lozano et al., 2021; Machado et al., 2018, 2019; Ingraffia et al., 2022). To contaminate the soils, the fibers were added into a blender (Waring® WSG30; Waring Commercial, Torrington, Connecticut, USA) as a band sandwiched between two layers of soil. We chose to incorporate the fibers into the soil using a blender to provide a more homogeneous distribution of the fibers in the soil. We tested the impact of the mixing time to establish a protocol which ensured a homogeneous distribution of the polyester MP fibers into the soil and that preserved the integrity of the MP fibers (which was evaluated through visual inspection using a stereo microscope Zeiss Stemi 2000-C; Fig. 1a and b). The mixture of soil and fibers was mixed five times for 5 s each. The same disturbance was also applied to the soil of the control treatment.
The three soil types, both treated and untreated, were used to fill 36 cylinders in total (height = 0.05 m; diameter = 0.05 m; 80 g of soil; Fig. 1c) to evaluate the impact of microplastics on soil properties (experiment I) and 36 soil trays of 0.30 m × 0.15 m × 0.10 m perforated at the bottom (Fig. 1d) for the rainfall simulation tests (experiment II).
For both treatments, that is the control (non-treated, Ctr) and the soils contaminated with polyester MP fibers, the soil samples were watered with distilled water to near-field capacity by capillarity and then incubated in a growth chamber in the dark at 23 ± 2 ∘C and 60 ± 5 % relative humidity for nearly 6 months. During the incubation period, the soil samples were watered once a week with distilled water to field capacity by capillarity. The experimental units were re-randomized at each irrigation event.
2.2 Experiment I: impact of polyester microplastic fibers on soil properties
For each soil type, the Ctr treatment was set up in eight replicates while the MP treatment consisted of four replicates (for a total of 36 cylindrical samples).
The soil water retention curve was determined after incubation, using the hanging water column apparatus (Dane et al., 2002a) for the pressure head, h, with values ranging from −0.03 to −1 m, and the pressure plate extractor (Dane et al., 2002b), for h values ranging from −3.3 to −150 m.
Briefly, the soil samples were placed on the porous plate of a glass funnel and saturated from the bottom by progressively raising the water level in a graduated burette (height was adjustable). Equilibration at h values of −0.2, −0.1, and −0.05 m was obtained successively in steps of 24 h each. Finally, the samples were fully saturated by submersion for 2 h. From saturation, soil samples were desorbed by imposing a sequence of eight h values (h = −0.03, −0.05, −0.10, −0.20, −0.30, −0.50, −0.70, and −1.0 m). At each h level, the volume of drained water into the burette was recorded. The volumetric water content, θ (m3 m−3), at each equilibrium stage was calculated by adding the drained volumes to the final θ value corresponding to h = −1 m that was determined by oven-drying the samples at 105 ∘C. The dry soil bulk density, BD (g cm−3), was calculated by the measured volume at the end of the experiment (i.e., h = −1 m) and using the oven-dried weight of the soil sample. Given that polyester can withstand temperatures as high as 150 ∘C and the added amount of fibers (0.5 %) was low, the drying process was not expected to have a significant effect on the measured BD values.
Then, the oven-dried soil samples were gently crushed and split into two subsamples, where one was used to determine the soil water content down to −150 m, while the other was used to determine the soil structure and the soil water stable aggregates.
To determine the soil water content at lower pressure head values, the soil was packed to the same BD value of the larger samples, in rings, with a diameter of 0.05 m and a height of 0.01 m. These repacked soil samples were then used to determine the soil water content corresponding to h = −3.3, −10, −33, and −150 m, using the pressure plate apparatus (Dane et al., 2002b).
The water retention model proposed by van Genuchten (1980) was then fitted to experimental data obtained for each soil sample. The water content values corresponding to saturation, θs, and to the pressure heads h = −0.10, −1.0, and −150 m (θ−0.1, θ−1.0, and θ−150) were then estimated from the model. The following capacitive indicators of soil physical quality were considered that are directly linked to the soil water retention properties (Iovino et al., 2016; Reynolds et al., 2009):
The Pmac indicator gives the volume of large (macro) pores (i.e., > 0.3 mm equivalent pore diameter), which indicates the soil's ability to quickly drain excess water and facilitate root proliferation (Reynolds et al., 2009). The AC index represents the ability of soil to store and provide essential soil air (Topp et al., 1997). The PAWC indicator is a measure of the ability of the soil to store and provide soil water that is available to crop roots.
To investigate soil aggregation and soil water stable aggregates, we used a wet sieving apparatus (Retsch AS 200) with a set of stacked sieves mesh of 2, 1, 0.6, 0.5, 0.25, and 0.106 mm. For the soil aggregation, we closed the water valve, and we performed a dry sieving using a 40 g subsample of air-dried soil from each treatment; the sieves were moved vertically at rate of 30 cycles per minute over a period of 3 min, and after recording the weight of each sieved fraction, the whole sample was reconstructed and used to assess water stable aggregates by using the same procedure with the opened water valve. All the analyses were conducted in a laboratory under controlled conditions of temperature (mean T = 22 ∘C).
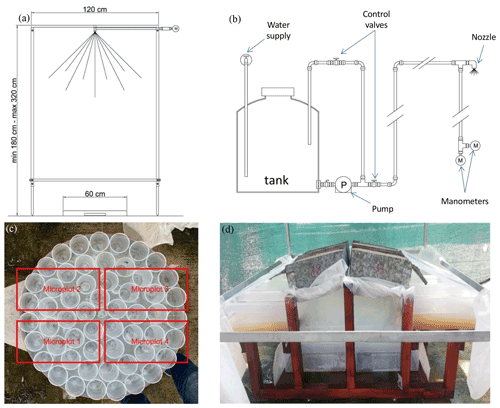
Figure 2(a) Scheme of the rainfall simulator and (b) the hydraulic setup. (c) Arrangement of the plastic cups to evaluate rainfall intensity uniformity and overlap between the plastic cups and the soil trays (in red). (d) View of the experimental soil trays during a rainfall simulation event.
Data obtained from dry and wet sieving were used to calculate the following:
-
The percentage of newly formed aggregates (> 600 µm; NFA), according to Lehmann et al. (2021):
where W>600 is the weight of the > 600 µm fraction after the air drying or wet sieving process, and Wsample is the total weight of the sample.
As the soil at the beginning of the experiment was sieved at 600 µm, this metric represents the formation of new macroaggregates (> 600 µm), certainly built from smaller-sized aggregates, representing a shift in aggregate size distribution.
-
The mean weight diameter (MWD; mm) calculated on data derived from dry (MWDdry) and wet (MWDwet) sieving, using the following equation (Deviren Saygın et al., 2012):
where i is the fraction size (> 2, 1–2, 1–0.6, 0.6–0.5, 0.5–0.25, 0.25–0.106, and < 0.106 mm), Wi is the oven-dried weight of each fraction after sieving process (dry and wet), di is the mean diameter of each size fraction (i.e., the mean inter-sieved size; for the fraction > 2.0 mm that was extremely small and always less than 1 %, it was considered to be 2.2 mm).
2.3 Experiment II: rainfall simulation
Soil with (MP) and without (Ctr) polyester MP fibers was used to perform the experiments with the rainfall simulator. For a given treatment, air-dried soil was used to fill the soil tray after placing a cotton guard cloth along its bottom to avoid soil loss through the draining holes. The soil was gradually poured into the tray, and it was compacted manually by repeatedly dropping the tray from a height of approximately 0.05–0.10 m until soil compaction ceased. Each soil sample had an initial bulk volume of 0.30 m × 0.15 m × 0.10 m = 4.50 × 10−3 m3. For a given soil and a given treatment (MP and Ctr), six soil trays were prepared (total sample size, N = 3 soils × 2 treatments × 6 soil trays = 36).
The rainfall simulator is a nozzle-type rainfall simulator, very similar to those described by Iserloh et al. (2012) and Ries et al. (2009). The major parts of the rainfall simulator (Fig. 2a) are a square metal frame (120 cm × 120 cm) with a nozzle located at its center and four telescopic steel legs in order to position the nozzle at different heights above the ground surface. A tank ensures the supply of water which is pressurized by a low-pressure 12 V bilge pump (Shurflo 2088-713-515; Fig. 2b). The water supply to the tank assures a steady flow. The flow rate is regulated by the control valve located on a recirculation circuit, and it is checked by a digital manometer (PCE-DMM 10) characterized by an accuracy equal to ± 0.5 %. In order to check the pressure measure, a further analog manometer is installed.
Rainfall intensity and its uniformity over the wetted area were checked before performing the runoff and soil loss measurement experiment. Initially, a total of 55 plastic cups, each with an upper surface area of 35.3 cm2, were placed on a circular metal frame, with a diameter of 60 cm, that was placed at a distance from the nozzle equal to the average distance between the nozzle and the soil trays and centered exactly below the nozzle of the rainfall simulator (Fig. 2c). Simulated rainfall was collected for 30 min, and a rainfall intensity value was then determined for each cup. Taking into account that four trays were planned to simultaneously be subjected to a given rainfall event, rainfall uniformity among these trays was verified. In particular, the data obtained on the cups corresponding to each tray were averaged to obtain four mean rainfall intensity values, that is, a value to be associated with each soil tray. Rainfall uniformity was assessed by calculating the following uniformity coefficient, CU (Christiansen, 1942):
with n = 55 being the number of cups, Ri = the rainfall intensity recorded by each cup, and R = the mean rainfall intensity.
Each individual rainfall simulation experiment was carried out by placing four soil trays on a frame imposing a steepness of 15 % to each try (Fig. 2d). This steepness value was chosen to consider a potentially favorable condition to appreciable soil erosion processes and also with the idea to replicate the experiment with natural rainfall events and larger plots in the future. For each soil try, surface runoff and the associated sediment load was conveyed through a gutter to a plastic container, and the same was done with reference to deep percolation. Lids and plastic sheets were used in order to only expose soil surface to rainfall during the experiment. The soil tray with the soil was weighed before and immediately after the rainfall event to obtain the change in the stored water in the soil volume. The nozzle was placed at a mean height of 1.70 m (measured from the middle of each tray). The whole experiment was carried out with a mean rainfall intensity of 33.4 mm h−1 (coefficient of variation, CV = 3.0 %) for 2 h. The rainfall simulation experiment was completed in 9 d. At the beginning of each working day, the rainfall intensity was measured by collecting water for 10 min in a cylindrical bucket with a diameter of 30 cm and at a distance from the nozzle equal to the average distance between the nozzle and the soil trays.
At the end of the experiment, all containers were transported to the laboratory and dried at 105 ∘C to constant weight to determine the total surface runoff volume, the total drained water volume, and the associated load of solids. For the drained water volume, this last quantity was always small and practically under the detectable quantity. Therefore, drained water was essentially clear in all cases. The data were then used to calculate, for each soil tray, total runoff per unit area, Ve (mm), total percolation per unit area, Pe (mm), total soil loss per unit area, Ae (g m−2), and sediment concentration in the surface runoff volume, Ce (g m−3). A check of the reliability of the Ve and Pe data was made, taking into account that the sum of total runoff, total percolation, and change in the stored water in the soil volume, denoted as Se (mm), was expected to nearly coincide with the rainfall depth for the simulated event, he.
2.4 Statistical analysis
The data of both experiments were analyzed in R (R Core Team, 2020), using a generalized least square models in the “nlme” package (Pinheiro et al., 2021), with the implemented “varIdDent()” function to account for the heterogeneity of variance. Model residuals were checked for heteroscedasticity and normal distribution.
All response variables within each soil type were compared between the two groups (MP minus Ctr), using the package “dabestr” (Ho et al., 2019) to calculate the effect sizes as unpaired mean differences and generate a bias-corrected and accelerated bootstrapped 95 % confidence intervals (CIs). We used this combined approach based on the expanding recognition of the limitation of using only p-value statistic approach and avoiding dichotomous cutoffs (Ho et al., 2019; Wasserstein and Lazar, 2020).
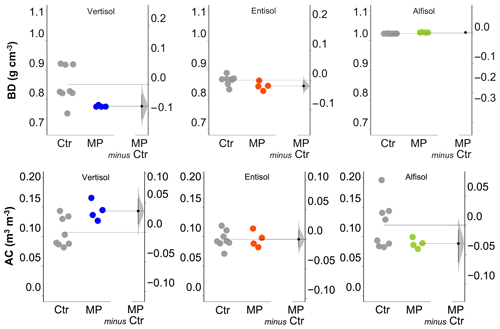
Figure 3Soil bulk density (BD) and air capacity (AC). Raw data of the control (Ctr, gray dots) and polyester MP fibers added to the treatment (MP, colored dots) are shown in the plot for each soil. The filled curve indicates the resampled distribution of the mean differences (Δ), given the observed data. Horizontally aligned with the mean of the test group, Δ is indicated by the black circle. The 95 % confidence interval of Δ is illustrated by the black vertical line.
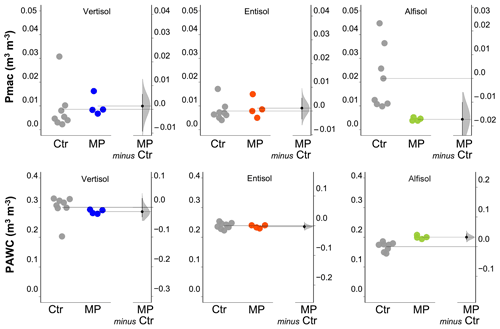
Figure 4Soil macroporosity index (Pmac) and plant-available water content (PAWC). Raw data of the control (Ctr, gray dots) and polyester MP fibers added to the treatment (MP, colored dots) are shown in the plot for each soil. The filled curve indicates the resampled distribution of the mean differences (Δ), given the observed data. Horizontally aligned with the mean of the test group, Δ is indicated by the black circle. The 95 % confidence interval of Δ is illustrated by the black vertical line.
3.1 Experiment I
The physical and hydrological effects of the treatment with polyester MP fibers differed with the soil type (Table S1 in the Supplement). In particular, for the Vertisol, polyester MP fibers caused a decrease in bulk density (−9 %) and an increase in air capacity (+34 %; Fig. 3), whereas no effect was found on soil macroporosity and plant-available water content (Fig. 4). In the Entisol, the contamination with polyester MP fibers did not cause any evident effect on the physical and hydrological characteristics. In the Alfisol, polyester MP fibers did not exert effects on BD but induced pronounced decreases in both AC (−26 %) and Pmac (−85 %) and an increase in PAWC (+19 %).
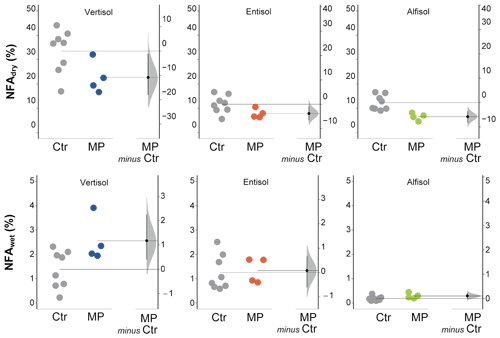
Figure 5Newly formed aggregates (> 600 µm) calculated from dry (NFAdry) and wet (NFAwet) sieving. Raw data of control (Ctr, gray dots) and polyester MP fibers added to the treatment (MP, colored dots) are shown in the plot for each soil. The filled curve indicates the resampled distribution of the mean differences (Δ), given the observed data. Horizontally aligned with the mean of the test group, Δ is indicated by the black circle. The 95 % confidence interval of Δ is illustrated by the black vertical line.
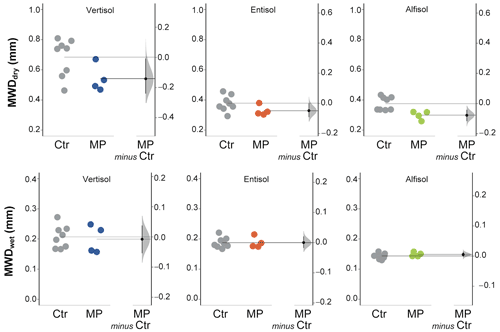
Figure 6Mean weight diameter (MWD, mm) calculated from dry (MWDdry) and wet (MWDwet) sieving. Raw data of control (Ctr, gray dots) and polyester MP fibers added to the treatment (MP, colored dots) are shown in the plot for each soil. The filled curve indicates the resampled distribution of the mean differences (Δ), given the observed data. Horizontally aligned with the mean of the test group, Δ is indicated by the black circle. The 95 % confidence interval of Δ is illustrated by the black vertical line.
The data obtained from dry sieving show how the contamination with polyester MP fibers negatively affected the percentage of newly formed aggregates (> 600 µm; −32 % in Vertisol; −47 % in Entisol; −33 % in Alfisol; Fig. 5). Only a small fraction of the newly formed aggregates was stable subsequent to the wet sieving (Fig. 5). In addition, MP had a minimal positive effect on NFAwet for the Vertisol, but it was uninfluential for the Entisol and the Alfisol (Fig. 5). Polyester MP fiber contamination determined a general decrease in MWDdry (−21 % in Vertisol and Alfisol; −13 % in Entisol; Fig. 6), while no effects due to polyester MP fibers were observed on MWD when soil samples were wet sieved (Fig. 6; Tables S2 and S3 in the Supplement).
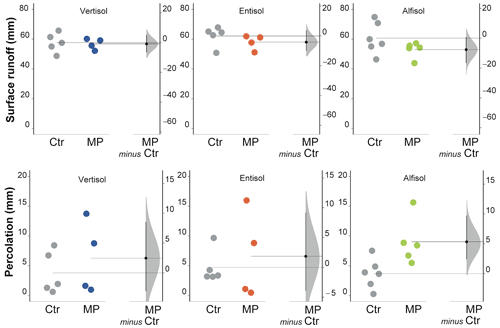
Figure 7Surface runoff and percolation. Raw data of control (Ctr, gray dots) and polyester MP fibers added treatment (MP, colored dots) are shown in the plot for each soil. The filled curve indicates the resampled distribution of the mean differences (Δ), given the observed data. Horizontally aligned with the mean of the test group, Δ is indicated by the black circle. The 95 % confidence interval of Δ is illustrated by the black vertical line.
3.2 Experiment II
3.2.1 Preliminary check of the simulated rainfall
Rainfall intensities, RIs, measured by 55 cups ranging from 31.3 to 37.4 mm h−1, with a mean of 33.8 mm h−1, a coefficient of variation, CV = 4.7 %, and a uniformity coefficient, CU, equal to 96.1 %. Considering only the cups corresponding to the area of the soil trays, RI = 33.1 mm h−1 and CU = 97.1 % were obtained. At the individual soil tray scale, the mean rainfall intensity varied from 32.5 to 34.0 mm h−1, depending on the tray, and the CU values were in the range 96.8 %–98.2 %. As compared with the performances of other rainfall simulators (Iserloh et al., 2013), these results suggested that rainfall intensities were reasonably uniform among the four simultaneously tested soil trays and also on each individual tray.
Mean rainfall intensities were overall stable in the 9 d of the rainfall simulator experiment (from 32.1 to 34.9 mm h−1). The whole experiment was carried out with a mean rainfall intensity of 33.4 mm h−1 (CV = 3.0 %), and it had a duration of 2 h. Therefore, the mean rainfall depth, he, was 66.8 mm.
On average, ) was equal to 5.3 mm. However, neglecting the seven cases characterized by values of ) greater than 20 mm, for which some anomaly occurred in the experiment, the mean of the remaining 29 values of ) is very close to zero (0.14 mm). Considering the consistency between he and (), the seven suspect runs were excluded from the considered dataset to check the soil treatment effect on Ve, Pe, Ae, and Ce
3.2.2 Runoff, percolation, soil erosion, and sediment concentration
Soil polyester MP fiber contamination did not induce changes in surface runoff (Fig. 7) in all soil types, although a slight decrease in Ve was perceived for both the Entisol and the Alfisol (unpaired mean difference −4.19 and −7.65 for Entisol and Alfisol, respectively; 95 % CIs of −10 to 3.74 for the Entisol and −16.7 to 0.69 for the Alfisol; Table S1). However, it affected percolation, which varied with the soil type. In particular, the analysis carried out separately by soil type allowed the highlighting of some different responses of the soils under evaluation; in the Alfisol, the contamination with polyester MP fibers increased percolation by 144 % (Fig. 7) as compared with the Ctr treatment. No effects were observed in the Vertisol and in the Entisol.
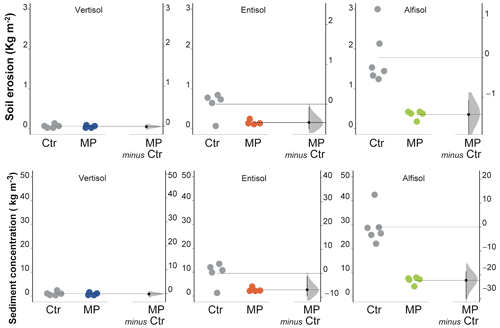
Figure 8Soil erosion and sediment concentration. Raw data of control (Ctr, gray dots) and polyester MP fibers added treatment (MP, colored dots) are shown in the plot for each soil. The filled curve indicates the resampled distribution of the mean differences (Δ), given the observed data. Horizontally aligned with the mean of the test group, Δ is indicated by the black circle. The 95 % confidence interval of Δ is illustrated by the black vertical line.
Soil erosion varied widely among the soils under evaluation, resulting in a decidedly limited amount in Vertisol and a particularly high one in Alfisol (Fig. 8; Table S1). Contamination with polyester MP fibers did not cause variations in the amount of eroded soil in the Vertisol (unpaired mean difference −0.01; 95 % CIs of −0.06 to 0.06), while it resulted in substantial reductions in both Entisol (−75 %; unpaired mean difference −0.46; 95 % CIs of −0.63 to −0.07) and Alfisol (−80 %; unpaired mean difference −1.43; 95 % CIs of −2.11 to −1.05). Similar results were observed for sediment concentration, as shown in Fig. 8 and in Table S1.
As hypothesized, the contamination with polyester MP fibers generally resulted in differentiated effects on soil aggregation and hydrological characteristics among the tested soils. In particular, the presence of polyester MP fibers determined a decrease in the soil bulk density on the Vertisol, a slight decrease in the Entisol, and no effects on the Alfisol. The data available in the literature about the impact of polyester MP fibers on soil bulk density are limited and not always concordant. For instance, Machado et al. (2018, 2019) observed a decrease in bulk density of a loamy sand soil at an increasing concentration of polyester MP fibers, while Zhang et al. (2019), in a study conducted on a clay loam soil (in field and in greenhouse conditions), found no differences in soil bulk density.
Polyester MP fiber contamination negatively affected the air capacity and macroporosity index of the Alfisol, maybe as a consequence of the smaller mean diameter of the newly formed aggregates. Given that the BD of this soil was unaffected by polyester MP fiber contamination, it can be supposed that total porosity was redistributed from macropores to meso-/micropores, as confirmed by the increase in PAWC. No modification of the capacitive indicators of soil physical quality was observed on the Entisol. For the Vertisol, the increase in both total porosity (decrease of BD) and AC suggested that the MP addition favored macroporosity. Meso-/micropores did not change appreciably, as indicated by the similar PAWC results for the Ctr and MP treatments.
Our data obtained from dry sieving show that the presence of polyester MP fibers changed the cohesion between aggregate-forming particles with a general reduction (although with different effect size on the three soil types) in the formation of new macroaggregates (> 600 µm). Similar results were reported by Boots et al. (2019) and Lozano et al. (2021). Zhang and Liu (2018), in a survey study of microplastic contamination in agricultural soils, found that the abundance of aggregate associated with plastic fibers is greater in the microaggregates than in the macroaggregates, and this, according to the same authors, would suggest that the presence of fibers in the microaggregate limits their possibility to be combined into macroaggregate. Machado et al. (2018) found that contamination of a loamy sand soil with polyester MP fibers decreased the fraction of soil forming dry aggregates larger than 1 mm but, at the same time, increased the formation of large soil clumps and therefore potentially provided additional macro-structures, absent in the non-contaminated soil. In contrast, Zhang et al. (2019) found that polyester MP fibers improve soil aggregation, helping to entangle soil particles more efficiently to form aggregates. Interestingly, in this experiment, the negative effects induced by the addition of polyester MP fibers on the aggregation capacity of the soils were, to a certain extent, of a decreasing magnitude as TOC increased (Vertisol>Alfisol>Entisol; according to the results of the dry sieving; Fig. 5, NFAdry; Table 1). Therefore, it would seem that the addition of polyester MP fibers interfered with the formation of macroaggregates by altering the binding mechanism in the soil. In particular, high organic matter levels made the MP contamination effects smaller. Similar results were also obtained by Liang et al. (2021), who found that the effects of polyester MP fibers on soil aggregation were organic matter dependent.
The presence of the contaminant, although determining a general decrease in newly formed aggregates, did not affect the formation of water stable aggregates, since we generally did not observe any appreciable effect of the polyester MP fibers on the MWD and NFA values obtained with the wet sieving. In contrast, Zhang et al. (2019) observed a significant increase in the stability of the macroaggregates (> 2 mm) in water and in the volume of the macropores (> 30 µm) after the addition of polyester MP fibers in a clay soil. On the other hand, Machado et al. (2018) found a significant decrease in water stable aggregates with increasing polyester MP fiber concentration in a sandy loam soil. Evidently, soil properties play an important role in guiding the effects of polyester MP fibers in the formation of macroaggregates and their water stability. The interactions that can occur between polyester MP fibers and fine soil particles are still poorly studied. Our results show that the effects of polyester MP fibers on soil structure and hydrological characteristics are most probably related to the different characteristics of the soil used in the experiments, as already hypothesized by Xu et al. (2020) and Zhang et al. (2019). Our three soil types differed for various characteristics (e.g., texture, clay mineralogy, TN, TOC, and CEC), which could have played a role in the observed responses to the contamination. Certainly, it is very difficult to identify how and on which factor/s polyester MP fibers influenced our results, also considering the several biotic and abiotic factors that interplay in the process of soil aggregation. For instance, our three soil types differ widely for clay mineralogy, i.e., montmorillonite in the Vertisols, illite in the Entisols, and kaolinite in the Alfisols. These three clay types largely differ in size, shape, specific surface area, and structure, and, as a consequence, their ability to form aggregates (Lal and Shukla, 2004). Therefore, it is possible to presume that polyester MP fibers could have differentially affected the ability of the abovementioned clay types to form aggregates during the incubation period. Those aspects deserve further investigation to clarify the mechanisms causing the different responses observed.
Polyester MP fiber contamination did not affect surface runoff and drainage in the Vertisol and in the Entisol, although a slight decrease in Ve was perceived for this last soil. However, it caused a slight decrease in surface runoff and an increase in percolation in the Alfisol, which has the coarsest texture among the three tested soils (Table 1). Moreover, the three studied soils differed widely in their susceptibility to erosion (Vertisol < Entisol < Alfisol). This result was partly expected, as many authors have highlighted that more silt and less organic matter in the soil enhance erodibility (Bonilla and Johnson, 2012; Chaney and Swift, 1984; Meyer and Harmon, 1984; Wischmeier and Smith, 1978). Adding polyester MP fibers had no effect on soil erosion in the least erodible soil (Vertisol), whereas it resulted in a pronounced decrease in soil erosion in the more erodible soils (Entisol and Alfisol). In other words, the tendency of microplastics to decrease soil erosion increased as the soil became intrinsically more erodible. The data therefore showed that contamination with polyester MP fibers modified the soil hydrological behavior (rainfall partition into surface runoff and percolation) only a little, and not for all soils, but it affected the soil erosion phenomena. Another way to summarize these results is that a decrease in soil erosion (Fig. 8) was linked with a slight decrease in the total runoff (Fig. 7). The MP influence on soil erosion was moderate or even negligible when the soil had only a slight inherent erodibility. Instead, it became appreciable in those cases in which the erosion phenomena were noticeable.
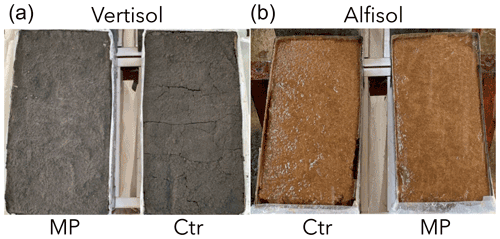
Figure 9Experimental units of experiment II (a Vertisol and b Alfisol) after the rainfall simulation.
At the end of the rainfall experiment, we observed differences between the Ctr and MP trays at the soil surface. In the trays of the Ctr treatment, micro-rills oriented parallel to the slope appeared during the rainfall–runoff event, especially in the contact zone between the soil and the box walls, or small cracks developed soon after rainfall, depending on the soil type (Fig. 9). Instead, neither micro-rills nor cracks were ever detected in the soil trays of the MP treatment. Therefore, it seems that the polyester MP fibers performed a soil particle binding action, possibly microbially mediated, that likely induced a decrease in soil erodibility. This decrease was not suggested by the sieving experiments, probably because mechanical impact of sieving cannot be considered equivalent to the impact of the raindrops (Fox and Le Bissonnais, 1998; Loch, 1994).
Moreover, after rainfall, the soil surface of the Ctr trays generally appeared smoother than the ones of the MP trays and, especially in the darker soil, the polyester MP fibers were noted to form a diffuse fluff on the soil surface by the end of the experiment (Fig. 9). Therefore, polyester MP fibers appeared to generally induce a greater resistance of the soil to flow tractive forces. An additional possible reason for the observed results was that these fibers were exposed to some degree as a consequence of erosion during the early stage of the experiment, but they remained entrapped by the subsoil. Therefore, the soil surface of the MP trays appeared to have an additional micro-roughness compared to that of the Ctr trays. Consequently, flow velocity and sediment transport capacity were likely greater in the Ctr trays than in the MP ones (Zhang et al., 2011). Perhaps the fluff formed by the exposed fibers also contributed to limit rainfall detachment, acting as a mulch.
The active erosion processes vary with the measurement scale (Cammeraat, 2002). At the soil tray scale adopted in this investigation, erosion is expected to be due to rain splash and sheet flow (Bagarello and Ferro, 2004), and it should be a transport-limited process as a consequence of the reduced rain impacted flow and the limitation of flow velocity (Boix-Fayos et al., 2006; Chaplot and Le Bissonnais, 2000). Therefore, the collected data provide information on particle detachment and the early stage of their transport that can be expected to occur in upland agricultural soils during intense rainfall events. However, in agricultural fields, rill erosion can dominate total soil erosion due to the simultaneous occurrence of long slopes (dozens of meters or more) and the exposure of bare soil surfaces to rainfall in some periods of the year (Rejman and Brodowski, 2005).
Although the current MP contamination level in agroecosystems is some orders of magnitude below the concentration applied in our experiment, in some areas, it is steadily increasing (Büks and Kaupenjohann, 2020); therefore it is of key importance to investigate such contamination levels which may represent future scenarios, as is common practice in global change biology.
The results showed that the presence of polyester MP fibers limits the neoformation of soil aggregates (labile in incubation period applied in our experiments). Likely, polyester MP fibers hinder the natural aggregation processes since they interpose between the fine soil particles and hence reduce the possibility of establishing bonds between these. Such results seem in contrast with what we observed in our rainfall simulation experiment, where polyester MP fibers reduced soil loss and sediment concentration, especially in the most erodible soils, which would suggest that the polyester MP fibers had a soil particle binding effect that reduced erodibility of the porous medium. In particular, the lack of rills in the MP treated trays suggested that polyester MP fibers induced a greater resistance of the soil to flow tractive forces of runoff. Other factors that could have played a role on the observed results include the following: (i) the mechanical impact of sieving cannot be considered equivalent to raindrop impact, (ii) the presence of polyester MP fibers favored a higher micro-roughness at the soil surface as compared with the non-treated soil, and consequently the flow velocity and sediment transport capacity decreased with the addition of polyester MP fibers, and (iii) the contaminant, acting as a mulch, could have exerted a physical protective action by intercepting the raindrops, allowing the dissipation of their kinetic energy, and limiting the splash effect that leads to the disintegration of the aggregates.
In conclusion, our experiments showed that the contamination of soils with polyester MP fibers exerts an impact on the soil structure, susceptibility to erosion, and other hydrological characteristics as a function of soil type. This aspect is of great interest and underlines how a complete understanding of the potential impacts of polyester MP fibers on terrestrial ecosystems requires special attention to the processes that occur in the soil and to the knowledge of the mechanisms underlying the different responses. The effects we observed are short term (the incubation period applied in our experiments was ∼ 6 months), and we currently do not know whether such effects would be maintained in the long term. Moreover, in this study, we used fresh MP particles, and the contamination with aged MP particles may lead to different results (Waldman and Rillig, 2020). However, there is insufficient information about aged MP particles at this time.
The applied experimental methodologies in this investigation provide much information on the effect of polyester MP fiber addition on several physical soil properties and hydrological processes. These methodologies should be applied in the future to different MPs concentrations and types to better understand the effects of those contaminants on different soils and to help cover the potentially very large parameter space that, as suggested by Rillig and Lehmann (2020), represents a major challenge of MPs research in terrestrial ecosystems.
All data that support the findings of this study are included within the article and any Supplement.
The supplement related to this article is available online at: https://doi.org/10.5194/soil-8-421-2022-supplement.
All authors (RI, GA, VB, FGC, DG, MI, AL, MCR, and ASF) conceptualized the study. GA, DG, and ASF acquired the funds to conduct the experiment. RI, FGC, DG, MI, and ASF carried out the experiments. RI, DG, and ASF analyzed the data. RI, DG, GA, ASF, MI, and VB wrote the first draft. AL and MCR collaborated on the ideas and contributed critically to the drafts. All authors gave the final approval for publication.
The contact author has declared that neither they nor their co-authors have any competing interests.
Publisher's note: Copernicus Publications remains neutral with regard to jurisdictional claims in published maps and institutional affiliations.
The authors acknowledge funding from the University of Palermo (Palermo, Italy).
This research has been supported by the Open Access Publication Initiative of Freie Universität Berlin.
This paper was edited by Peter Fiener and reviewed by Frederick Büks and one anonymous referee.
Alimi, O. S., Farner Budarz, J., Hernandez, L. M., and Tufenkji, N.: Microplastics and Nanoplastics in Aquatic Environments: Aggregation, Deposition, and Enhanced Contaminant Transport, Environ. Sci. Technol., 52, 1704–1724, https://doi.org/10.1021/acs.est.7b05559, 2018.
Bagarello, V. and Ferro, V.: Plot-scale measurement of soil erosion at the experimental area of Sparacia (southern Italy), Hydrol. Process., 18, 141–157, https://doi.org/10.1002/hyp.1318, 2004.
Bagarello, V., Ferro, V., and Flanagan, D.: Predicting plot soil loss by empirical and process-oriented approaches. A review, J. Agr. Eng., 49, 1–18, 2018.
Bergami, E., Rota, E., Caruso, T., Birarda, G., Vaccari, L., and Corsi, I.: Plastics everywhere: first evidence of polystyrene fragments inside the common Antarctic collembolan Cryptopygus antarcticus, Biol. Lett., 16, 20200093, https://doi.org/10.1098/rsbl.2020.0093, 2020.
Boix-Fayos, C., Martínez-Mena, M., Arnau-Rosalén, E., Calvo-Cases, A., Castillo, V., and Albaladejo, J.: Measuring soil erosion by field plots: Understanding the sources of variation, Earth-Sci. Rev., 3–4, 267–285, https://doi.org/10.1016/j.earscirev.2006.05.005, 2006.
Bonilla, C. A. and Johnson, O. I.: Soil erodibility mapping and its correlation with soil properties in Central Chile, Geoderma, 189–190, 116–123, https://doi.org/10.1016/j.geoderma.2012.05.005, 2012.
Boots, B., Russell, C. W., and Green, D. S.: Effects of Microplastics in Soil Ecosystems: Above and Below Ground, Environ. Sci. Technol., 53, 11496–11506, https://doi.org/10.1021/acs.est.9b03304, 2019.
Bradford, J. M., Ferris, J. E., and Remley, P. A.: Interrill Soil Erosion Processes: II. Relationship of Splash Detachment to Soil Properties, Soil Sci. Soc. Am. J., 51, 1571–1575, https://doi.org/10.2136/sssaj1987.03615995005100060030x, 1987.
Büks, F. and Kaupenjohann, M.: Global concentrations of microplastics in soils – a review, SOIL, 6, 649–662, https://doi.org/10.5194/soil-6-649-2020, 2020.
Cammeraat, L. H.: A review of two strongly contrasting geomorphological systems within the context of scale, Earth Surf. Proc. Land., 27, 1201–1222, https://doi.org/10.1002/esp.421, 2002.
Chaney, K. and Swift, R. S.: The influence of organic matter on aggregate stability in some British soils, J. Soil Sci., 35, 223–230, 1984.
Chaplot, V. and Le Bissonnais, Y.: Field measurements of interrill erosion under different slopes and plot sizes, Earth Surf. Proc. Land., 25, 145–153, https://doi.org/10.1002/(SICI)1096-9837(200002)25:2<145::AID-ESP51>3.0.CO;2-3, 2000.
Christiansen, J. E.: Irrigation by sprinkling, Berkeley: University of California, 1942.
Crossman, J., Hurley, R. R., Futter, M., and Nizzetto, L.: Transfer and transport of microplastics from biosolids to agricultural soils and the wider environment, Sci. Total Environ., 724, 138334, https://doi.org/10.1016/j.scitotenv.2020.138334, 2020.
Dane, J. H., Hopmans, J. W., and Topp, G. C.: Hanging water column, in: Methods Soil Anal. Part 4, 680–683, 2002a.
Dane, J. H., Hopmans, J. W., and Topp, G. C.: Pressure plate extractor, in: Methods Soil Anal. Part 4, 688–690, 2002b.
Deviren Saygın, S., Cornelis, W. M., Erpul, G., and Gabriels, D.: Comparison of different aggregate stability approaches for loamy sand soils, Appl. Soil Ecol., 54, 1–6, https://doi.org/10.1016/j.apsoil.2011.11.012, 2012.
Dris, R., Gasperi, J., and Tassin, B.: Sources and Fate of Microplastics in Urban Areas: A Focus on Paris Megacity, in: Freshwater Microplastics: Emerging Environmental Contaminants?, edited by: Wagner, M. and Lambert, S., Springer International Publishing, Cham, 69–83, https://doi.org/10.1007/978-3-319-61615-5_4, 2018.
Fox, D. M. and Le Bissonnais, Y.: Process-Based Analysis of Aggregate Stability Effects on Sealing, Infiltration, and Interrill Erosion, Soil Sci. Soc. Am. J., 62, 717–724, https://doi.org/10.2136/sssaj1998.03615995006200030025x, 1998.
Gee, G. W. and Bauder, J. W.: Particle-size Analysis, in: Methods of Soil Analysis, John Wiley & Sons, Ltd, 383–411, https://doi.org/10.2136/sssabookser5.1.2ed.c15, 1986.
Hartmann, N. B., Hüffer, T., Thompson, R. C., Hassellöv, M., Verschoor, A., Daugaard, A. E., Rist, S., Karlsson, T., Brennholt, N., Cole, M., Herrling, M. P., Hess, M. C., Ivleva, N. P., Lusher, A. L., and Wagner, M.: Are We Speaking the Same Language? Recommendations for a Definition and Categorization Framework for Plastic Debris, Environ. Sci. Technol., 53, 1039–1047, https://doi.org/10.1021/acs.est.8b05297, 2019.
Ho, J., Tumkaya, T., Aryal, S., Choi, H., and Claridge-Chang, A.: Moving beyond P values: data analysis with estimation graphics, Nat. Methods, 16, 565–566, https://doi.org/10.1038/s41592-019-0470-3, 2019.
Horton, A. A., Walton, A., Spurgeon, D. J., Lahive, E., and Svendsen, C.: Microplastics in freshwater and terrestrial environments: Evaluating the current understanding to identify the knowledge gaps and future research priorities, Sci. Total Environ., 586, 127–141, https://doi.org/10.1016/j.scitotenv.2017.01.190, 2017.
Ingraffia, R., Amato, G., Iovino, M., Rillig, M., Giambalvo, D., and Frenda, A. S.: Polyester microplastic fibers in soil increase nitrogen loss via leaching and decrease plant biomass production and N uptake, Environ. Res. Lett., 17, 054012, https://doi.org/10.1088/1748-9326/ac652d, 2022.
Iovino, M., Castellini, M., Bagarello, V., and Giordano, G.: Using Static and Dynamic Indicators to Evaluate Soil Physical Quality in a Sicilian Area, Land Degrad. Dev., 27, 200–210, https://doi.org/10.1002/ldr.2263, 2016.
Iserloh, T., Fister, W., Seeger, M., Willger, H., and Ries, J. B.: A small portable rainfall simulator for reproducible experiments on soil erosion, Soil Tillage Res., 124, 131–137, https://doi.org/10.1016/j.still.2012.05.016, 2012.
Iserloh, T., Ries, J. B., Arnáez, J., Boix-Fayos, C., Butzen, V., Cerdà, A., Echeverría, M. T., Fernández-Gálvez, J., Fister, W., and Geißler, C.: European small portable rainfall simulators: A comparison of rainfall characteristics, Catena, 110, 100–112, 2013.
Lal, R. and Shukla, M. K.: Principles of Soil Physics, CRC Press, Boca Raton, 736 pp., https://doi.org/10.4324/9780203021231, 2004.
Lehmann, A., Fitschen, K., and Rillig, M. C.: Abiotic and Biotic Factors Influencing the Effect of Microplastic on Soil Aggregation, Soil Syst., 3, 21, https://doi.org/10.3390/soilsystems3010021, 2019.
Lehmann, A., Leifheit, E. F., Feng, L., Bergmann, J., Wulf, A., and Rillig, M. C.: Microplastic fiber and drought effects on plants and soil are only slightly modified by arbuscular mycorrhizal fungi, Soil Ecol. Lett., https://doi.org/10.1007/s42832-020-0060-4, 2020.
Lehmann, A., Leifheit, E. F., Gerdawischke, M., and Rillig, M. C.: Microplastics have shape- and polymer-dependent effects on soil aggregation and organic matter loss – an experimental and meta-analytical approach, Microplastics Nanoplastics, 1, 7, https://doi.org/10.1186/s43591-021-00007-x, 2021.
Liang, Y., Lehmann, A., Yang, G., Leifheit, E. F., and Rillig, M. C.: Effects of Microplastic Fibers on Soil Aggregation and Enzyme Activities Are Organic Matter Dependent, Front. Environ. Sci., 9, 97, https://doi.org/10.3389/fenvs.2021.650155, 2021.
Loch, R. J.: A method for measuring aggregate water stability of dryland soils with relevance to surface seal development, Soil Res., 32, 687–700, https://doi.org/10.1071/sr9940687, 1994.
Lowery, B., Swan, J., Schumacher, T., and Jones, A.: Physical properties of selected soils by erosion class, J. Soil Water Conserv., 50, 306–311, 1995.
Lozano, Y. M., Lehnert, T., Linck, L. T., Lehmann, A., and Rillig, M. C.: Microplastic Shape, Polymer Type, and Concentration Affect Soil Properties and Plant Biomass, Front. Plant Sci., 12, https://doi.org/10.3389/fpls.2021.616645, 2021.
Maaß, S., Daphi, D., Lehmann, A., and Rillig, M. C.: Transport of microplastics by two collembolan species, Environ. Pollut., 225, 456–459, https://doi.org/10.1016/j.envpol.2017.03.009, 2017.
Machado, A. A., Lau, C. W., Till, J., Kloas, W., Lehmann, A., Becker, R., and Rillig, M. C.: Impacts of Microplastics on the Soil Biophysical Environment, Environ. Sci. Technol., 52, 9656–9665, https://doi.org/10.1021/acs.est.8b02212, 2018.
Machado, A. A., Lau, C. W., Kloas, W., Bergmann, J., Bachelier, J. B., Faltin, E., Becker, R., Görlich, A. S., and Rillig, M. C.: Microplastics Can Change Soil Properties and Affect Plant Performance, Environ. Sci. Technol., 53, 6044–6052, https://doi.org/10.1021/acs.est.9b01339, 2019.
Mamedov, A. I. and Levy, G. J.: Soil erosion–runoff relations on cultivated land: Insights from laboratory studies, Eur. J. Soil Sci., 70, 686–696, https://doi.org/10.1111/ejss.12759, 2019.
Meyer, L. D. and Harmon, W. C.: Susceptibility of Agricultural Soils to Interrill Erosion, Soil Sci. Soc. Am. J., 48, 1152–1157, https://doi.org/10.2136/sssaj1984.03615995004800050040x, 1984.
Napper, I. E., Davies, B. F. R., Clifford, H., Elvin, S., Koldewey, H. J., Mayewski, P. A., Miner, K. R., Potocki, M., Elmore, A. C., Gajurel, A. P., and Thompson, R. C.: Reaching New Heights in Plastic Pollution—Preliminary Findings of Microplastics on Mount Everest, One Earth, 3, 621–630, https://doi.org/10.1016/j.oneear.2020.10.020, 2020.
Nelson, D. W. and Sommers, L. E.: Total Carbon, Organic Carbon, and Organic Matter, in: Methods of Soil Analysis, John Wiley & Sons, Ltd, 961–1010, https://doi.org/10.2136/sssabookser5.3.c34, 1996.
O'Kelly, B. C., El-Zein, A., Liu, X., Patel, A., Fei, X., Sharma, S., Mohammad, A., Goli, V. S. N. S., Wang, J. J., Li, D., Shi, Y., Xiao, L., Kuntikana, G., Shashank, B. S., Sarris, T. S., Hanumantha Rao, B., Mohamed, A. M. O., Paleologos, E. K., Nezhad, M. M., and Singh, D. N.: Microplastics in soils: an environmental geotechnics perspective, Environmental Geotechnics, 8, 586–618, https://doi.org/10.1680/jenge.20.00179, 2021.
Pinheiro, J., Bates, D., DebRoy, S., and Sarkar, D.: R Core Team, _nlme: Linear and Nonlinear Mixed Effects Models, R package version 3.1-152, https://CRAN.R-project.org/package=nlme, 2021.
Qi, R., Jones, D. L., Li, Z., Liu, Q., and Yan, C.: Behavior of microplastics and plastic film residues in the soil environment: A critical review, Sci. Total Environ., 703, 134722, https://doi.org/10.1016/j.scitotenv.2019.134722, 2020.
R Core Team 2020: R: A language and environment for statistical computing, R Foundation for Statistical Computing, Vienna, Austria, https://www.R-project.org/, 2020.
Rehm, R., Zeyer, T., Schmidt, A., and Fiener, P.: Soil erosion as transport pathway of microplastic from agriculture soils to aquatic ecosystems, Sci. Total Environ., 795, 148774, 2021.
Rejman, J. and Brodowski, R.: Rill characteristics and sediment transport as a function of slope length during a storm event on loess soil, Earth Surf. Proc. Land., 30, 231–239, https://doi.org/10.1002/esp.1177, 2005.
Reynolds, W. D., Drury, C. F., Tan, C. S., Fox, C. A., and Yang, X. M.: Use of indicators and pore volume-function characteristics to quantify soil physical quality, Geoderma, 152, 252–263, 2009.
Ries, J. B., Seeger, M., Iserloh, T., Wistorf, S., and Fister, W.: Calibration of simulated rainfall characteristics for the study of soil erosion on agricultural land, Soil Tillage Res., 106, 109–116, 2009.
Rillig, M. C. and Lehmann, A.: Microplastic in terrestrial ecosystems, Science, 368, 1430–1431, https://doi.org/10.1126/science.abb5979, 2020.
Rillig, M. C., Ingraffia, R., and de Souza Machado, A. A.: Microplastic Incorporation into Soil in Agroecosystems, Front. Plant Sci., 8, p. 1805, https://doi.org/10.3389/fpls.2017.01805, 2017a.
Rillig, M. C., Ziersch, L., and Hempel, S.: Microplastic transport in soil by earthworms, Sci. Rep., 7, 1362, https://doi.org/10.1038/s41598-017-01594-7, 2017b.
Topp, G. C., Reynolds, W. D., Cook, F. J., Kirby, J. M., and Carter, M. R.: Physical attributes of soil quality, in: Developments in soil science, vol. 25, Elsevier, 21–58, 1997.
van Genuchten, M. Th.: A Closed-form Equation for Predicting the Hydraulic Conductivity of Unsaturated Soils, Soil Sci. Soc. Am. J., 44, 892–898, https://doi.org/10.2136/sssaj1980.03615995004400050002x, 1980.
Waldman, W. R. and Rillig, M. C.: Microplastic Research Should Embrace the Complexity of Secondary Particles, Environ. Sci. Technol., 54, 7751–7753, https://doi.org/10.1021/acs.est.0c02194, 2020.
Wasserstein, R. L. and Lazar, N. A.: ASA Statement on Statistical Significance and p-Values, in: The Theory of Statistics in Psychology: Applications, Use, and Misunderstandings, edited by: Gruber, C. W., Springer International Publishing, Cham, 1–10, https://doi.org/10.1007/978-3-030-48043-1_1, 2020.
Weithmann, N., Möller, J. N., Löder, M. G. J., Piehl, S., Laforsch, C., and Freitag, R.: Organic fertilizer as a vehicle for the entry of microplastic into the environment, Sci. Adv., 4, eaap8060, https://doi.org/10.1126/sciadv.aap8060, 2018.
Wischmeier, W. H. and Smith, D. D.: Predicting rainfall erosion losses: a guide to conservation planning, Department of Agriculture, Science and Education Administration, 1978.
Xu, B., Liu, F., Cryder, Z., Huang, D., Lu, Z., He, Y., Wang, H., Lu, Z., Brookes, P. C., Tang, C., Gan, J., and Xu, J.: Microplastics in the soil environment: Occurrence, risks, interactions and fate – A review, Crit. Rev. Env. Sci. Tec., 50, 2175–2222, https://doi.org/10.1080/10643389.2019.1694822, 2020.
Zhang, B., Yang, X., Chen, L., Chao, J., Teng, J., and Wang, Q.: Microplastics in soils: a review of possible sources, analytical methods and ecological impacts, J. Chem. Technol. Biot., 95, 2052–2068, https://doi.org/10.1002/jctb.6334, 2020.
Zhang, G. S. and Liu, Y. F.: The distribution of microplastics in soil aggregate fractions in southwestern China, Sci. Total Environ., 642, 12–20, https://doi.org/10.1016/j.scitotenv.2018.06.004, 2018.
Zhang, G. S., Zhang, F. X., and Li, X. T.: Effects of polyester microfibers on soil physical properties: Perception from a field and a pot experiment, Sci. Total Environ., 670, 1–7, https://doi.org/10.1016/j.scitotenv.2019.03.149, 2019.
Zhang, G.-H., Wang, L.-L., Tang, K.-M., Luo, R.-T., and Zhang, X. C.: Effects of sediment size on transport capacity of overland flow on steep slopes, Hydrol. Sci. J., 56, 1289–1299, https://doi.org/10.1080/02626667.2011.609172, 2011.
Zubris, K. A. V. and Richards, B. K.: Synthetic fibers as an indicator of land application of sludge, Environ. Pollut., 138, 201–211, https://doi.org/10.1016/j.envpol.2005.04.013, 2005.