the Creative Commons Attribution 4.0 License.
the Creative Commons Attribution 4.0 License.
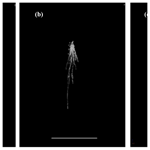
The effect of tillage depth and traffic management on soil properties and root development during two growth stages of winter wheat (Triticum aestivum L.)
Mary Harty
Saoirse R. Tracy
Kevin McDonnell
The management of agricultural soils during crop establishment can affect root development due to changes in the soil structure. This paper assesses the influence of tillage depth (250 mm, 100 mm, and zero tillage) and traffic management (conventional tyre pressure, low tyre pressure, and no traffic) on wheat root system architecture during winter wheat (Triticum aestivum L.) tillering and flowering growth stages (GS) at a long-term tillage trial site. The study revealed that zero-tillage systems increased crop yield through significantly greater root biomass (P<0.001), root length density, and deeper seminal rooting analysed using X-ray computed tomography (CT) (P<0.001) compared with trafficked treatments. In general, conventional-pressure traffic had a significant negative influence on the crop yield (P<0.01), root development (0.001), bulk density (P<0.05), and total soil porosity (P<0.05) of deep- and shallow-tillage conventional-pressure systems compared with no-traffic zero- and deep-tillage systems. Visual improvements in soil structure under zero-tillage conditions may have improved crop rooting in zero-tillage treatments through vertical pore fissures (biopores), enhancing water uptake during the crop flowering period. This study highlights the increasing implications of soil structural damage on root system architecture created by machinery traffic in crop production. Although the tillage method was less important, the constricted root systems were more pronounced in conventional-pressure shallow-tillage and deep-tillage systems, emphasizing the importance of using controlled-traffic farming methods to improve soil management and reduce the trafficked areas of agricultural fields.
- Article
(4716 KB) - Full-text XML
-
Supplement
(447 KB) - BibTeX
- EndNote
Soil resources are under significant pressure from anthropogenic activities, especially conventional tillage. The resulting soil degradation has significant implications for food security globally (Lal, 2010). Changing weather patterns from prolonged rain to drought periods are being experienced on a global scale, substantiating the challenges faced by food producers. In 2018, worldwide wheat production fell by 34.5×106 t due to prolonged droughts across Europe, Australia, and Canada. Soil compaction from field traffic is a well-recognized problem in many parts of the world (Chan et al., 2006; Arvidsson and Keller, 2007; Naderi-Boldaji et al., 2018), affecting 33×106 ha in Europe alone (Akker and Canarache, 2001). Soil compaction is a form of physical degradation caused by short crop rotations and heavy farm machinery working on low-organic-matter soils under wet conditions; this results in a loss of pore space due to an externally applied load, forcing soil aggregates together (Defossez and Richard, 2002). The resulting anaerobic high-density soils have a significantly reduced capacity to store the water and nutrients required by growing crops (Hamza and Anderson, 2005), and severely compacted soils prevent soil exploration from root growth (Tracy et al., 2012).
Soil compaction is due in part to the pressure to complete field operations, such as harvesting or drilling, often during short windows of good weather, and it is exacerbated by the increasing use of larger machinery with heavier axle loads designed to improve operational efficiencies. Common agricultural operations are conducted using wheeled farm machinery, which has tripled in weight and power since 1966 with wheel loads rising by a factor of 6 (Chamen, 2006). When soils are cultivated under moist or wet conditions, they cannot withstand the compressive forces applied post-cultivation by heavy farm machinery traffic during operations such as seeding (Raper, 2005), resulting in soil degradation (Batey, 2009). When soil is wet, tyre stress can propagate a greater distance down through the soil profile. The depth and severity of soil stress is related to the soil moisture, the traction device applied (track or tyre), the track size, the tyre inflation pressure, and the wheel load (Naderi-Boldaji et al., 2018).
Reforming the approach to soil management to mitigate challenges such as soil compaction and soil erosion offers significant financial and environmental benefits compared with conventional agriculture. Cultivation practices using minimal- or zero-tillage techniques are widespread across many climatic conditions from the semi-arid Canadian plains to the temperate climates of western Europe. In conventional tillage, the soil is either inverted > 200 mm using a mouldboard plough or deeply ripped using tines. The soil is then cultivated again to break down soil aggregates to a crumb structure or fine tilth that is suitable to receive seeds (Morris et al., 2010). Conservation tillage, also known as non-inversion tillage or reduced tillage, has been used for decades to improve soil structure and health (Skaalsveen et al., 2019). Under conservation tillage, soil is disturbed to a lesser extent (< 100 mm using tines or discs) or not disturbed at all, such as under zero-tillage which involves the direct placement of seed into undisturbed crop residues (Soane et al., 2012).
The successful adaption of reduced-tillage systems is not universally guaranteed, with factors such as soil texture and drainage, crop type, and weather influencing successful implementation (Soane et al., 2012). In northern Europe, crop yields under reduced cultivation systems rarely exceed those achieved by ploughing (Arvidsson, 2010). However, an exception to this is drier arid climates, such as Spain, in which no-tillage conditions have been found to improve crop yields due to moisture retention in below average rainfall years (Muñoz-Romero et al., 2010). Higher bulk density and penetration resistance are typically found throughout the formerly tilled or “plough pan” layer in non-tilled soils within the first 2 years of adoption, resulting in root mechanical impedance (Boguzas et al., 2006). However, over time, long-term zero tillage has been shown to cause improvements in soil pore architecture and continuity throughout the soil profile due to bioturbation, suggesting that roots could penetrate to lower soil horizons (Cooper et al., 2021).
To date, studies have focused on how tillage influences physical soil properties (e.g. bulk density, cone penetrometer, and soil aeration) with root and crop yield responses (Whalley et al., 2008; Pires et al., 2017; Czyż, 2004). Soil types and tillage systems have a considerable influence on the structural integrity of soil which controls rooting potential (Morris et al., 2017). Studies have shown that low-pressure tyres can reduce surface compaction compared with high tyre pressure (Soane et al., 1980; Boguzas and Hakansson, 2001). As traffic increases the soil strength and reduces a plant root's ability to penetrate soil layers, it is important to understand the relationship between tillage depth and root system architecture during the growing season in response to traffic. A dearth of information exists on how tillage depth and tyre pressure affect rooting properties and crop yield at longer-term field sites. Yield reduction by soil surface compaction can increase abiotic stress in plants in three ways: (1) it reduces soil aeration; (2) it increases the mechanical impedance of roots; and (3), as a consequence of point 2, it reduces root exploration of the soil, thereby mitigating the extraction of water and nutrients from the soil resource (Chamen, 2011).
Quantitative measurement of root system architecture in three dimensions (3D) has become tractable using X-ray computed tomography (CT) in pot experiments (Mairhofer et al., 2017). Few examples of root studies using high-resolution X-ray CT have been successfully conducted in field trials using undisturbed soil cores. Many studies have focused on measuring soil structural properties, such as porosity, soil pore size, and distribution, and the influence of the tillage method and traffic (Millington et al., 2017; Rab et al., 2014). However, studying root development and architecture in 3D field-structured soils with X-ray CT remains challenging due to a bottleneck in the rapid and standardized root extraction methods available, insufficient resolution, and the inability to segment similarities in greyscale values between root and organic materials (Zhou et al., 2021; Mooney et al., 2012; Pfeifer et al., 2015).
The purpose of this paper is to identify the effect and interaction of machinery traffic and tillage depth using commercial crop establishment methods. The root architecture, soil physical structure, and crop yield were studied during two key growth stages of winter wheat (Triticum aestivum L.). X-ray CT was deployed to establish if root architecture behaviours could be captured in situ in the soil structural environment created by the tillage method. Three cultivation practices and traffic management systems were studied: deep-tillage (250 mm), shallow-tillage (100 mm), and zero-tillage practices under no-traffic, low-tyre-pressure, and conventional-tyre-pressure management systems. The objectives of this study were (i) to assess the relationship between the above-mentioned traffic management systems and tillage depths as well as their effects on the root system architecture and soil physical properties and (ii) to utilize 3D image analysis along with 2D destructive methods to verify the rooting properties responsible for crop yield.
2.1 Site and soils
The study took place during the 2018–2019 growing season. The experimental site was 3.12 ha located at Harper Adams University (HAU), Edgmond, Newport, UK (52.779738∘ N, 2.426886∘ W). The HAU site is a loamy sand soil consisting of the Ollerton and Salwick series soils (eutric endogleyic Arenosol and chromic endostagnic Luvisol respectively) (Millington et al., 2017). Further details of the soil properties are described in Table 1. To highlight if any site variability existed across the site, soil properties were examined for fertility (pH and nutrient levels), bulk density, soil strength, and soil moisture. Particle size analysis (Gee and Or, 2002) was conducted to determine soil texture classifications. The trial site was established in 2011 for previous studies with plots and treatments carried out in the same location.
Each plot received the same tillage and traffic treatment as this study. During the trial period, the site was treated with a standard crop rotation of winter wheat (Triticum aestivum L.) harvested in 2012; winter wheat in 2013; winter barley (Hordeum vulgare L.) in 2014; winter barley in 2015 followed by a cover crop “TerraLife N-Fixx” (DSV United Kingdom Ltd, 2015); spring oats in 2016; spring wheat in 2017; and winter beans in 2018. In the year prior to this study, it was necessary to plant a break crop (2017–2018) as part of a standard crop rotation to improve soil conditions and reduce diseases such as “take-all” (Gaeumannomyces graminis var. tritici). A field bean (Vicia faba) break crop was planted, and yields were assessed to ensure that the trial site was uniform with no underlying issues. For this trial, winter wheat (Triticum aestivum L. `Graham') followed the bean crop and was drilled in early October 2018 when the soil was dry and friable and soil temperatures were > 6 ∘C. The seeding rate was 250 seeds m−2, and drilling took place on 5 October. This is in line with local normal farming practice.
Table 1Description of the topsoil (0–300 mm) properties for the Harper Adams University trial site, Shropshire, UK.
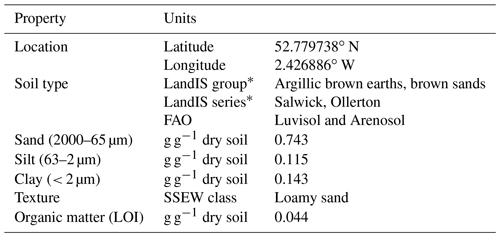
* LandIS Soils guide (Cranfield University, 2021). LOI denotes loss on ignition, FAO represents the Food and Agriculture Organization of the United Nations, and SSEW denotes the Soil survey of England and Wales.
2.2 Experiment design
The experiment was a randomized 3 × 3 factorial arrangement of nine treatments in four complete replicate blocks. Each plot was 4 m wide and 84 m long with the exception of block 4. Block 4 was 78.2 m long for operational reasons. Tramlines were at a 90∘ angle to plots with 24 m spacing for fertilizing and spraying operations throughout the growing season. A split-plot design was used: half the plot (30 m) was designated for sampling, and the other half was undisturbed for yield data collection. The half plot for sampling was subdivided for the two sampling stages, ensuring that sampling did not occur near the same location as the previous sample. Cultivation for spring beans in 2017 was performed at three depths: 250 mm for deep tillage, 100 mm for shallow tillage, and direct into stubble for zero tillage. In the winter wheat trial, soil cores were collected at tillering (GS 25, where GS represents growth stage) and the flowering stage (GS 61–69) (Zadoks et al., 1974) in July 2019.
Three commercial crop establishment systems were used that comprised three different tillage depths. The tillage treatments are denoted as follows: treatment 1 – deep tine cultivator at 250 mm (DT) for deep tillage, similar to Ren et al. (2019); treatment 2 – shallow disc cultivation at 100 mm (ST); and treatment 3 – zero tillage using a direct seed drill (ZT). In combination with the different tillage depths, three traffic regimes were used in this study: no traffic (NT), conventional tyre pressure (CP), and low tyre pressure (LP). Tillage depths were combined with traffic management practices for the nine treatments: DTNT, DTCP, DTLP, STNT, STCP, STLP, ZTNT, ZTCP, and ZTLP. Using GPS guidance and markers, trafficked areas of each plot were marked out to ensure that samples were taken from the correct location. A GPS (Trimble FMX display unit) was used to apply all tillage and drilling applications. All vehicle passes from cultivation and drilling occurred in the same traffic lanes for the duration of the trial. During drilling, the drill coulters directly behind the tractor wheel passes were marked to aid the identification of trafficked crop rows. During harvest, it was necessary to avoid driving on non-trafficked areas, with the plot combine restricted the trafficked zones. This ensured that a controlled-traffic farming (CTF) system was replicated.
2.2.1 Tillage equipment and tyres
Primary cultivations in HAU involved a rigid tine and conical disc cultivator (Vaderstad TopDown) at 250 mm depth to cut surface residues and loosen, mix, and consolidate the seedbed. The same implement was used for shallow-tillage treatments with tines adjusted upwards to reduce tillage depth (100 mm). A 290 hp Massey Ferguson 8480 with a track width of 2.1 m was used. Increased flexion AXIOBIB tyres were fitted on the rear axle (IF 650/85 R38 179D TL) and at the front (IF 600/70 R30 159D TL). A pneumatic disc seed drill (Vaderstad Spirit) was used to sow the crop with 167 mm row spacing. The same drill was used to sow the zero-tillage plots with the tines and discs lifted to minimize disturbance (Kaczorowska-Dolowy et al., 2019a).
For the tyre pressure treatment, the conventional tyre treatments were inflated to 1 bar for the front and rear tyres during cultivations. Low-tyre-pressure treatments and CTF plots operated on a 0.7 bar front and a 0.8 bar rear axle. A front weight block of 540 kg was applied to the tractor for tillage primary cultivation. All operations were performed in the same trafficked zones in order to maintain traffic-free zones for the CTF plots. During harvest, a Claas Dominator combine operated on a 4 m header, matching plot sizes (Smith, 2016). Crop husbandry was carried out in accordance with the Agriculture and Horticulture Development Board (AHDB) guidelines and soil fertility test analysis (AHDB, 2018).
2.2.2 Soil physical properties
To represent the bulk density of the tillage treatments, soil bulk density samples were also collected within the trafficked and non-trafficked area of the plot. Samples were replicated three times. Each core sample was 50 mm in width and 300 mm in length. An Eijkelkamp® soil corer was used to take bulk densities samples. Each bulk density sample was taken within 0.5 m of the location of the soil cores taken for X-ray CT. The objective was to represent the physical constraints (or lack of) on root growth in each plot examined. The method used in this study involved splitting the bulk density sample into three 100 mm sections (0–100, 100–200, and 200–300 mm), similar to Smith (2016). The corer was opened in the field and split using a knife and ruler.
The core sections were stored in resealable bags and labelled before transportation to the laboratory for analysis. Intact fresh soil cores were weighed prior to drying to record sample fresh weights. Samples were placed into an oven at 105 ∘C for 24 h and reweighed to determine the moisture percentage, as per Eq. (1), and dry bulk density, as per Eq. (2) (Campbell and Henshall, 2000).
2.2.3 Penetration resistance (PR)
Soil penetration resistance data were collected on each plot (in the trafficked zones and in the centre of the plot) down to 450 mm with a depth increment of 25 mm between each recorded penetrometer reading. A cone penetrometer (Datafield, Ukraine) was used, recording the soil strength (in kPa) as well as the location and the depth via a built-in GPS device. Only the PR samples were recorded at 450 mm to complete a reading on the data logger. It is also widely known that roots penetrate past “tillage pans” (Bengough et al., 2011). Five penetrations were made both under and between the trafficked zones on each plot at GS 25 sampling to represent each treatment. PR was measured when soil conditions were at field capacity to ensure the accuracy of each reading.
2.2.4 Soil porosity analysis
Before soil porosity analysis on ImageJ software (version 1.52) (Schneider et al., 2012) could commence, an image stack was created in VGStudio MAX® for each scan. The contrast was adjusted to improve the uniformity and visibility of the soil pores. The “Register object” tool corrected scan discrepancies for soil core angle. Straightening the scan allowed a cylindrical shape to be cropped and the tube edges and air space outside of the soil core to be removed. This enabled soil data to be captured throughout the soil core. A new volume was selected and extracted from the original. This created a separate cropped image volume to work from. The “Surface determination” tool in VGStudio MAX® was used to threshold pore spaces within the solid matrix. The tool defines the contour of objects, separating 3D data into regions, providing meaningful soil data. The image was then inverted to remove the extracted variables from the image, highlighting the pore spaces in the soil core. The processed image was exported as a .TIFF image stack for further analysis using ImageJ software.
Soil pore characteristics were measured using X-ray CT to establish information about the 3D soil environment for root growth without disrupting the structural integrity of the soil core. The original greyscale X-ray CT images were analysed using ImageJ software. The scale was set for each data set to define the spatial scale of the active image. The unit of length was set in millimetres, and the known distance was 0.045 mm (45 µm). Each scanned core was cropped to remove the area outside of the soil column. The action of soil coring during sampling had the effect of loosening the bottom 20 mm of the core; therefore, 415 slices at the bottom of each scan were discarded to remove the loosening effect due to the sampling process. The downward movement of the polyvinyl chloride (PVC) pipe also caused a smearing effect on the soil at the outside edge of the core, and this area was also removed by cropping.
The processed image was 1220 pixels × 1220 pixels in size. Applying the contrast enhancement filter helped normalize all slices. The filter reduces the differences in the pixel grey-level between slices, which is known as beam hardening (Wildenschild et al., 2002). The ImageJ Huang automatic threshold algorithms were used for each scan to create binarized images and separate the air-filled pores from the background region. The binarized scans were despeckled twice to remove unwanted noise within each scanned image, thereby improving the analysis and accuracy of the investigated pores. The lookup table (LUT) was inverted to change the white pores to black, ensuring that the analysis calculated the air-filled pores and not the soil matrix. The resulting binary images were analysed using the “Analyze particles” tool, which provided information on the average pore size, total area, and percentage porosity for each individual image.
2.2.5 Soil core sampling
The field soil core size was chosen to capture as much root material growing in the field as possible while minimizing the trade-off between image resolution and core size that exists with X-ray CT technology (Mooney et al., 2012; Zhou et al., 2021). The core dimensions were consistently 70 mm × 300 mm (diameter × depth) for each sample. Soil cores were extracted from the field sites at GS 25 in February and again at GS 61 in June. Sampling was carried out at GS 61 during wheat anthesis, when root growth is at its peak (Gregory et al., 1978). Due to the high moisture deficits at HAU (43 mm) during sampling at GS 61 in early July, the soil sample area was wetted with 2.5 L of water that was allowed to infiltrate. This lubricated the soil, reduced soil fracturing, and allowed tube insertion and soil core extraction to take place as smoothly as possible. PVC drainage pipes were cut to size (70 × 300 mm), and these tubes were used to collect soil cores, as per Millington et al. (2017).
A single wheat plant sample was located at random in each plot. The selected plant was cut at the base of the stem with scissors, and the above-ground biomass was discarded. The PVC tube was placed (plant centred) directly over the remaining plant stubble to maximize root system capture. Tubes were inserted into the soil using a mallet in the crop rows in the centre of the plots between the wheel tracks (un-trafficked by wheels) for the no-traffic samples. A second core was taken in the trafficked zones for the tyre pressure treatments. A small block of timber was used when hammering in the tube in order to protect the tubes and soil cores from damage. A total of 72 samples were extracted during each sampling occasion, and they were examined in this study. The PVC tubes were inserted into the soil to a depth of 300 mm. The soil core was extracted carefully using a spade, and the sample locations were backfilled with soil. Following sampling, cores were sealed (top and bottom) using tape, labelled, and carefully placed into boxes protected with bubble wrap. Cores were tightly packed and insulated to minimize the movement and drying of samples during transit to the laboratory for analysis. Samples were transferred to refrigerated storage (< 4 ∘C) to prevent and reduce compositional changes to the soil through biological degradation.
2.2.6 X-ray computed tomography (CT) – root analysis
Soil cores were transferred to the University College Dublin (UCD) X-ray CT facility at the Rosemount Experimental Research Station at Belfield Campus, UCD, Ireland. The soil cores were scanned using a Phoenix® × m 240 kV scanner (GE Measurement & Control Solutions, Wunstorf, Germany). The × m was set at a voltage of 90 kV and a current of 400 µA in order to optimize the contrast between the background soil and root material. A voxel resolution of 45 µm was achieved by using the “multi-scan” option to scan in four segments. A total of 1800 projection images per section were taken at 200 m s−1 per image using the “`fast scan” option, with image averaging of 1 and 0 skip. No filters were used during scanning. The total scan time per core was 24 min, or 6 min per section. Once scanning was complete, the images were reconstructed using Phoenix datos|x2 rec reconstruction software, and the four scans were assembled into one 3D volume for the whole core. Core samples were scanned within a week of the sampling date, and the scanned core was 300 mm in length and 70 mm diameter. The software corrected movements during the scanning process and removed noise from scanned images.
2.2.7 X-ray CT root segmentation
Image analysis for X-ray CT images was performed using VGStudio MAX®, version 3.2, software (Volume Graphics GmbH, Heidelberg, Germany) to segment roots and soil porosity. Roots were segmented by setting seed points and using selected threshold values in the “Region grower” that enabled fast and accurate selection of greyscale voxels (3D pixels) pertaining to root materials. The root system was extracted from the greyscale CT image of soil using the VGStudio MAX® semi-automated local adaptive thresholding “Region growing” selection tool, similar to Tracy et al. (2013). Root volumes were calculated by segmenting the root region of interest (ROI). Once the roots were segmented from the image, the erosion and dilation tool was selected at one pixel using the Region growing tool. Root system architecture parameters such as root vertical depth, root volume, and root surface area were measured from the segmented root systems. Root vertical depth was calculated on the z axis in VGStudio MAX® from the length of a complete root from the base seed point.
2.2.8 Destructive 2D root analysis
After the soil cores were scanned, the soil and root material were separated by gently washing the roots with a water jet hose. Two sets of sieves with a respective mesh size of 2 and 1 mm collected root material. Roots were washed, and soil material was removed before the roots were placed into a sealed and labelled bag filled with water. The washed root samples were placed into a freezer until scanning and analysis with WinRHIZO® scanning software (version 2016a, Regent Instruments, Canada) commenced. The root samples were thawed before scanning with the above-mentioned software. Large root stumps were removed from the sample prior to placing it inside the tray in order to reduce root misrepresentation (Wang and Zhang, 2009). Roots were placed onto a clear transparent tray (30 cm × 20 cm) with water. A pair of plastic forceps were used to spread out seminal and lateral roots. Images were scanned at a resolution of 600 dpi (42 µm pixel size) with an Epson Perfection V800 scanning system. Root images were measured for root length, root surface area, average root diameter, and root volume for the total soil core. This output was used to verify the 3D root outputs from VGStudio MAX® (Flavel et al., 2017; Tracy et al., 2012). The WinRHIZO™ software enabled the rapid assessment of root parameters. It calculated the root volume by determining the average root diameter and root length by pixel counting the 2D root image and then assuming that the root shape was cylindrical. WinRHIZO™ uses a skeletonization method for characterizing root systems (Himmelbauer et al., 2004). The software utilizes greyscale values in .TIFF file format. The output of the images was distinguished by global thresholding analyses for root diameter, while root length was validated by skeleton images. After the WinRHIZO™ scans, the roots were removed from the scanning tray using forceps. The root samples were dried at 70 ∘C for 24 h, and the root biomass samples were weighed.
2.3 Soil moisture deficit model
A soil moisture deficit (SMD) was calculated based on the SMD hybrid model for Irish grassland (Schulte et al., 2005). The model is based on weather parameters and soil drainage classes. The inputs of the model include data on maximum and minimum temperatures, rainfall (mm), wind speed (m s−1), and sunshine hours, which were taken from the nearest weather station located in Newport, Shropshire, 6 km from the site (Met Office, 2019).
2.4 Statistics
Data from the scanned (destructive and non-destructive) images and root biomass were not normally distributed. Non-normal data do not meet the assumptions underpinning an ANOVA (analysis of variance); therefore, all data underwent log transformation (in Microsoft Excel) before being exported to Minitab 18® where an ANOVA was performed to homogenize the variances of the compared means. A two-way ANOVA was performed using the general linear model in the Minitab® software package. All means were analysed for normality before the test was run. When significant effects of rooting were detected, a regression analysis was utilized to observe the relationship between the variables. For linear regression analysis, residuals of data were made to ensure that the assumptions of the analysis were met (e.g. normal distribution and constant variance). Normality was tested using the Anderson–Darling test in Minitab 18®.
3.1 Growing conditions during crop season
In 2018, crops were established at low soil moisture levels, which may have reduced the soil compaction caused by tillage operations across all site locations. From January to August (2019), 418.6 mm of rainfall was recorded at HAU, with 68 mm in total for January and February. Soil moisture deficits reached 66.2 mm at HAU (Supplement Fig. S1) by early June 2019. High soil moisture deficits were recorded from early April to June, causing drought stress during rapid growth periods (Met Office, 2019).
3.1.1 Soil properties – bulk density and penetrometer resistance
The calculated probability (P value) using two-way linear model analysis (ANOVA) results are shown in Table 2. In the top 0–100 mm, bulk density was significantly higher in the DTCP (1.66 Mg m−3) and STCP (1.44 Mg m−3) treatments compared with ZTNT (0.994 Mg m−3) and DTNT (0.97 Mg m−3) (P<0.01). STNT (1.09 Mg m−3) was significantly higher than ZTNT and DTNT and only significantly lower than DTCP. In the middle horizon (100–200 mm), a significant interaction between the traffic treatments was found. Bulk density was significantly lower in DTNT (1.07 Mg m−3) compared with the DTCP (1.63 Mg m−3) and ZTCP (1.58 Mg m−3) treatments (P<0.05). In the bottom 200–300 mm layer measured, no significant tillage × traffic interaction was found (P>0.05). Table 2 shows a significant traffic effect on soil bulk density in the 0–100 mm layer and the 100–200 mm layer (P<0.01). No-traffic treatments revealed a lower bulk density in both layers compared with trafficked treatments.
Table 2Analysis of variance for bulk density × traffic, tillage, and tillage × traffic.
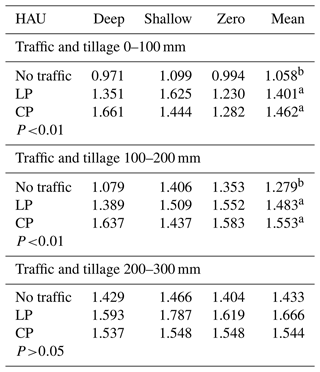
* A significant difference between means is represented by different letters.
Penetration resistance (PR) was recorded in February 2019 when the soil was at field capacity. Measurements were grouped into three groups, 0–150, 150–300, and 300–450 mm depth layers. A linear variance of analysis between tillage, traffic, and the tillage × traffic interaction was conducted, and the results are shown in Table 3. Figure 1 depicts the combined three layers grouped into one 0–450 mm graph. The one-way ANOVA analysis revealed highly significant differences for each layer. In the 0–150 mm layer, DTNT recorded the lowest kilopascal (kPa) readings and was significantly lower than ZTCP, STCP, STLP, ZTLP, and ZTNT (P<0.000). DTCP and DTLP showed significantly lower kilopascal readings than ZTLP, STLP, STCP, and ZTCP. ZTCP recorded the highest kilopascal reading and was significantly higher than ZTLP, ZTNT, STNT, DTLP, DTCP, and DTNT. In the second layer (150–300 mm), similar trends were found and were highly significant (P<0.000). STCP showed the highest kilopascal reading (3193.5 kPa) and was significantly higher than STNT, ZTNT, DTNT, DTLP, and DTCP. In contrast, DTNT recorded the lowest reading (1268.4 kPa) and was significantly lower than ZTNT, STNT, ZTLP, ZTCP, STCP, and STLP. STNT revealed a significantly lower kilopascal reading than STLP, ZTCP, and STCP. ZTNT penetrometer readings were significantly lower than all trafficked ZT and ST treatments. In the lower depth (300–450 mm), DTNT was significantly lower than STLP, STCP, ZTCP, ZTLP, and STNT (P<0.000). The results revealed a significant traffic interaction for 0–150 mm (P<0.001), 150–300 mm (P<0.000), and 300–450 mm (P<0.000). Again, the no-traffic PR was significantly lower than trafficked treatments. When tillage was measured, a significant effect was observed for each layer studied (0–150 mm = P<0.000, 150–300 mm = P<0.000, and 300–450 mm = P<0.000). In the top layer, deep tillage was significantly lower than shallow- and zero-tillage treatments. Further, shallow tillage was significantly lower than zero-tillage PR. In the second and third layer, deep tillage was lower than shallow- and zero-tillage treatments. A tillage × traffic interaction was observed in the first layer (0–150 mm) (P<0.001), but the second and third layer were not significant (P<0.067, P<0.313 respectively).
Table 3Analysis of variance table for penetration resistance × traffic, tillage, and tillage × traffic interactions between 0 and 150, 150 and 300, and 300 and 450 mm.
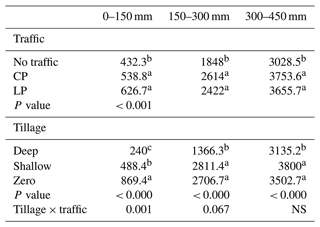
* A significant difference between means is represented by different letters.
3.1.2 Soil porosity
The results of the ANOVA analysis of the CT-measured porosity (0–220 mm) are presented in Table 4. Soil porosity results were split into two soil layers, 0–100 and 100–200 mm respectively. In the top 0–100 mm layer, DTNT showed a significantly higher total pore space (P<0.01) compared with all other treatments except ZTNT. Tillage had a significant effect on soil porosity in the no-traffic samples in the 0–100 mm layer (P<0.05). Deep tillage with no traffic had higher soil porosity (22.72 %) than shallow tillage treatments with no traffic (10.58 %). There was no significant difference between soil porosity under zero-tillage and shallow-tillage conditions in the no-traffic samples. Traffic had a significant effect on overall porosity. In deep-tillage treatments, overall porosity 22.72 % (no traffic) was reduced to 8.08 % (under low-tyre-pressure conditions) and to 6.50 % (under conventional-tyre-pressure conditions). Traffic had little effect on shallow- and zero-tillage porosity in the top 0–100 mm when compared to the no-traffic samples, with small reductions in porosity. In the second examined layer, the 100–200 mm zone, tillage and traffic were not significantly different (P<0.487). The percentage porosity, shown in Table 4, indicates a sharp decline in the lower depth, with only 9.02 % in DTNT. DTCP treatments recorded the lowest porosity (3.96 %).
3.1.3 Destructive 2D root analysis
The interaction between the tillage system and traffic protocols using destructive root measuring methods (WinRHIZO™) are shown in Fig. S2 (for GS 25) and Fig. S3 (for GS 61) in the Supplement. The analysis of variance results for WinRHIZO™ are presented in Table 5. At GS 25, no significant differences were found between tillage, traffic, and traffic × tillage interactions. However, the WinRHIZO™ analysis revealed a tendency towards increased root growth in no-traffic treatments. At the later growth stage (GS 61), Table 5 depicts results showing highly significant interactions between traffic and tillage systems for root length density (RLD) (P< 0.001) and root length (P< 0.001), root surface area (P< 0.002), and a traffic effect on root volume (P< 0.05). Analysis of variance results showed a significant effect of tillage system (P< 0.01) for deep-tillage systems compared with shallow- and zero-tillage systems. When traffic was considered, an even greater significance was recorded (P< 0.000) for root length, with no-traffic treatments being significantly greater than conventional-pressure (CP) and low-pressure (LP) treatments. A significant tillage (P< 0.05) and traffic (P< 0.000) were found for root surface area (mm2). Deep tillage showed significance over shallow tillage but not over zero tillage. The root surface area was significantly lower in CP than in no traffic but not in LP. The root volume (mm3) showed a significant traffic effect, but tillage was not significant. Indeed, no traffic was significantly greater than CP but was not greater than LP. For RLD (mm3), a significant tillage (P< 0.01) and traffic effect (P< 0.000) was found. Deep tillage established greater RLD compared with zero and shallow tillage, whereas no traffic was significantly greater than CP and LP.
When traffic × tillage interactions were compared, no significant difference was found (Table 5). However, individually, the treatments were significantly greater than the others. For example, DTNT showed significantly higher RLD, root surface area, and root length compared with ZTCP, STCP, and STLP. The root volume was significantly higher in DTNT compared with ZTCP and STCP. DTNT produced nearly double the root length compared with ZTCP. In contrast to DTCP, the root surface area decreased by 36 % compared with un-trafficked areas (no-traffic samples). In shallow- and zero-tillage systems, the root surface area was reduced by 32 % and 63.6 % respectively in conventional pressure samples compared with un-trafficked samples. There was no significant difference in root diameter and between all tillage and traffic regimes. The results demonstrate that there was no significant difference in RLD at the tillering stage nor could trends be found as roots were undeveloped. However, at anthesis, the RLD was significantly higher under non-trafficked tillage treatments when compared with DTCP, STCP, and ZTCP (Fig. S3b).
3.1.4 X-ray CT root analysis results
Significant differences were found between traffic treatments at GS 61 for RLD and vertical root depth using non-destructive analysis with VGStudio MAX 3.2® (Table 4). The X-ray CT scans revealed significantly longer vertical rooting (measured via the z axis in VGStudio MAX®) in ZTNT (112.7 mm) compared with the DTCP (60.44 mm), DTLP (66.96 mm), and STLP (65.39 mm) treatments (P< 0.001). ZTNT showed significantly greater RLD (0.000098 mm m−3) compared with the DTCP (0.000052 mm m−3), DTLP (0.000058 mm m−3), STLP (0.000058 mm m−3), and ZTCP (0.000060 mm m−3) treatments (P< 0.001). Root volume and surface area showed no significant difference using X-ray CT. However, similar trends were found to the conventional WinRHIZO™ method. Traffic had more of an influence on rooting than the tillage method, which did not have any significant effect on root parameters. As RLD is an important root trait commonly measured to estimate water uptake (White et al., 2015), linear regression was used to verify the relationship between root depth and RLD. A significant relationship (P< 0.001) was found with a coefficient of determination of R2=0.54 (Fig. S4 in the Supplement).
Table 6Root system architecture analysis using a non-destructive method.
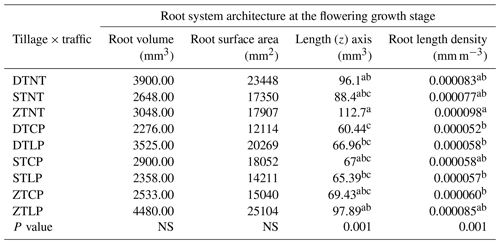
* Significant differences between means are represented by different letters.
Figure 2 shows root biomass results for GS 25 and GS 61. No significant differences between treatments were found at GS 25 (P< 0.848). However, root biomass was significantly different for tillage × traffic with a high confidence level at GS 61 (P< 0.001). DTNT (0.829 g) showed significantly (P< 0.001) greater root biomass than the STCP (0.437 g) and ZTCP (0.4530 g) treatments. DTNT did not significantly differ from ZTLP (0.7992 g), ZTNT (0.7939 g), DTLP (0.6837 g), STNT (0.4991 g), or STLP (0.4923 g). The results show that DTNT, ZTLP, and ZTNT resulted in nearly 50 % greater root biomass compared with the STCP and ZTCP treatments. Tillage treatments (centre line where there was no traffic effect) did not differ significantly with respect to root biomass.
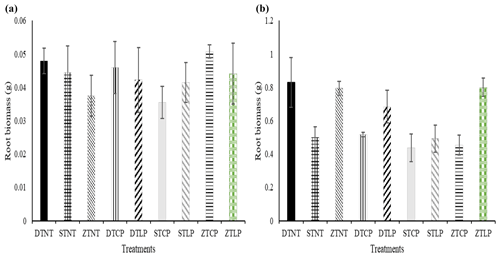
Figure 2Root biomass at tillering (GS 25) and flowering (GS 61) for the traffic and tillage treatments. Treatments are represented by initials. For tillage, the abbreviations for the treatments are D – deep, S – shallow, and Z – zero; the abbreviated treatments for traffic are NT – no traffic, LP – low-pressure tyre, and CP – conventional-pressure tyre.
3.2 Crop yield
Crop yield was highly significant between the traffic treatments and tillage (P< 0.01), as shown in Fig. 3. ZTLP had the highest yield (11 385 kg ha−1) and was significantly greater than DTLP (10 757 kg ha−1), STCP (10 700 kg ha−1), STNT (10 678 kg ha−1), STLP (10 638 kg ha−1), and DTCP (10 613 kg ha−1). All three zero-tillage treatments trended higher than deep-tillage and shallow-tillage treatments. ZTLP showed a 500 kg ha−1 yield advantage over DTNT (NS) and between 628 and 772 kg ha−1 over trafficked treatments and STNT (with high significance). In general, this study did not show a trend in yield between conventional-tyre-pressure and low-tyre-pressure treatments. For deep tillage, conventional tyre pressure reduced the crop yield by 144 kg ha−1 (1.34 %) compared with low tyre pressure. When compared with the no-traffic sample, conventional tyre pressure consistently reduced yield by 272 kg ha−1 (2.5 %) in deep tillage. Although not significant, traffic trended towards improving yield by 30 kg ha−1 (0.03 %) using conventional tyre pressure and 340 kg ha−1 (3.07 %) using low tyre pressure. No trends were found in shallow-tillage treatments. A linear regression of root depth using X-ray CT showed a significant relationship with crop yield (P< 0.001) and a positive correlation (r = 0.54). However, the coefficient of determination was low, R2 = 0.3094 (Fig. S5). Moreover, regression analysis also showed a significant relationship between root biomass and crop yield (P< 0.01). However, the correlation between the two variables was weaker (r = 0.43; coefficient of variance R2 = 0.1859). This indicates that root depth is a stronger predictor of crop yield.
4.1 Soil physical responses to tillage
Previous studies have shown that zero-tillage systems increase bulk density and penetration resistance and reduce porosity in the early years of adoption from conventional-tillage systems (Christian and Ball, 1994; Six et al., 2004; Mangalassery et al., 2014b; Smith, 2016). Indeed, Soane et al. (2012) reported that significant regeneration of soil structure requires a 3-year period from tillage depending on the previous historic land management practice. Moreover, values decrease in the long term with multiple benefits including improved saturated conductivity, soil organic matter, and air permeability in lower soil horizons. Using uniaxial compression tests, Arvidsson (1998) showed that soils with < 30 g kg−1 organic matter were likely to suffer 11 % higher crop yield loss due to compaction. It is plausible that the actions of soil fauna such as earthworms and old root channels could have reduced bulk density over time (Fig. 4), as identified by Angers and Caron (1998). Roots promote soil structural formation by increasing soil aggregation. Root mucilage production, root hair formation, and localized wetting and drying cycles encourage a reduction in soil bulk density (Bengough, 2012).
4.1.1 Soil porosity in response to tillage
Sandy soils, due to their adhesive and coarse-grain nature, have reduced porosity, including lower levels of micropores, compared with loamy soils (Arvidsson, 1998). The aggregation potential in this sandy loam soil is low. In the presence of plants, porosity and pore connectivity have been shown to decrease further compared with clay cohesive soils, which tend to increase in porosity through flocculation and aggregation (Bacq-Labreuil et al., 2018). Here, we found soil porosity to be low in general across all treatments. When comparing cultivation systems, we found that shallow tillage in the 0–100 mm layer had significantly lower porosity (10.58 %) compared with deep tillage (22.72 %). Although zero tillage also recorded low porosity values (10.72 %), it was not significantly different to the other two systems.
A key characteristic of non-tilled soils is a change in the soil pore architecture, with vertically orientated fissures connected down through the soil profile created by biopores (Fig. 4). Similar findings have resulted in reduced CO2 fluxes and increased saturated hydraulic conductivity by surface-connected porosity (Cooper et al., 2021). Cooper et al. (2021) found similar soil porosity levels between conventional- and zero-tillage systems, with zero-tillage total porosity ranging from < 5 %, 10 %, and 12 % on average over 1–5, 6–10, and 11–15 years respectively. The significant increase in deeply tilled soil porosity substantially increases soil respiration, resulting in up to 13.8 times higher CO2 emissions through increased oxidation and carbon breakdown (Reicosky et al., 1999). The lower porosities in non-tilled and shallowly tilled soils reduce the space for gas exchange, thereby decreasing soil respiration and supporting carbon sequestration; this, in turn, increases the recalcitrant levels of carbon in the soil. Mangalassery et al. (2014) found similar porosity results using X-ray CT methods to measure the effect of tillage method on greenhouse gas emissions, reporting significantly higher porosity in tilled soil (13.6 %) compared with non-tilled soil (9.6 %) in the top 0–100 mm layer. However, in deeper soil horizons, no difference could be found between the tillage systems. The findings in this experiment agree with that study: both tillage methods did not differ significantly in the 100–200 mm layer, with lower soil porosities recorded.
4.1.2 Root system architecture responses to tillage
The “hidden half” (i.e. roots) of plants is difficult to interpret in field studies (Lynch and Brown, 2001). A large root system is characterized by abundant biomass, a large root length, and a high root length density (Ehdaie et al., 2010; Hamblin and Tennant, 1987). Root biomass was an important indicator of root size, showing a treatment effect at anthesis compared with the tillering stage. In general, root biomass had a positive relationship with grain yield. Zero-tillage treatments, both un-trafficked and trafficked, at low pressure had greater root biomass over all shallow-tillage treatments and deep-tillage trafficked treatments at conventional pressure. Although deep-tillage treatments had the highest root biomass by GS 61, they did not achieve the highest yield. No significant difference in root biomass was found between tillage treatments, confirming that roots are more sensitive to traffic than to tillage method. RLD in shallow- and zero-tillage treatments trafficked under conventional pressure showed sensitivity to compaction, reducing the area of soil explored. RLD is an important parameter for characterizing root growth (Doussan et al., 2006) and has been used as a key root parameter for modelling water uptake in previous studies (Tinker and Nye, 2000; Javaux et al., 2013). Muños-Romero et al. (2010) and Chakraborty et al. (2008) have indicated that RLD is a positive predictor of crop yield. Although RLD had a positive correlation with crop yield in this study, root depth (using X-ray) displayed a much stronger relationship with crop yield (Fig. S5).
4.1.3 Soil physical responses to traffic
In line with this paper's hypothesis, traffic effects were more influential with respect to crop and root performance than the tillage system. The presence of trafficked areas in both zero- and deep-cultivation treatments increased soil bulk density significantly in deep-tillage treatments (Table 2). Our data show similar findings, with zero and deep tillage significantly reducing bulk density values in un-trafficked zones. However, in trafficked treatments, high tyre pressure combined with deep-tillage treatments resulted in higher bulk density values due to the loss of inherent strength in tilled soil, resulting in compression of the soil particles (Raper, 2005; Soane et al., 1986). Chan et al. (2006) observed that traffic after deep tillage increased bulk density values from 1.27 to 1.54 Mg m−3, emphasizing the effect of traffic on the reduced bearing capacity of the deeply tilled soil. The optimum soil density has been reported to differ between soil types in previous studies. Indeed, Czyż (2004) established a soil-type interaction between crop yield, bulk density, and root mass, concluding with sandy loam soils (similar to this study) having an optimum bulk density value of 1.54–1.66 Mg m−3. However, in this study, root biomass was significantly reduced, with treatments displaying similar soil density values to that reported optimum. Although conventional-pressure tyres significantly affected zero tillage in the 100–200 mm layer, traffic affected the 0–200 mm layer under deep-tillage conditions. In shallow-tillage treatments, the top 0–100 mm layer was considerably impacted by high tyre pressure.
4.1.4 Soil porosity in response to traffic
Compared with non-trafficked treatments, trafficked soil generally displayed a sharp decline in soil porosity in the top 0–100 mm layer. Tyre inflation pressure is one of the key contributors to soil stress in the 100–1000 mm layer (Botta et al., 2008). The effect of re-compaction from traffic after cultivation was often worse in deep-tillage treatments, with a lower percentage porosity than in zero- and shallow-tillage systems (see Table 4 for the DTLP and DTCP treatments). In deeply cultivated soils, water infiltration rates can be reduced by up to 82 % after a single vehicle pass (Chyba, 2012), which has agronomic implications such as reduced water and nutrient use efficiency by up to 22 %, thereby potentially resulting in crop yield penalties of up to 38 % (Ishaq et al., 2001). Yield effects due to traffic were modest in our study due to low-soil-moisture conditions during sowing in autumn 2018 (Met Office, 2019). Dry soil has increased soil strength, reducing the effects of soil compaction, as the soil load support capacity would have increased, thereby increasing the permissible ground pressure (Hamza and Anderson, 2005).
4.1.5 Penetrometer responses to traffic
Penetrometer resistance (PR) is a useful parameter for the evaluation of soil physical resistance to root growth (Otto et al., 2011). In general, traffic had a considerable influence on soil PR in this study, as depicted in Fig. 6. The greatest contrast in soil penetration resistance was between trafficked and un-trafficked soil, with zero tillage showing the highest resistance under conventional-tyre-pressure conditions. Recent studies have shown that roots can exploit pores and bypass layers of strong soil (Atkinson et al., 2020). Axial pressure from repeated traffic in ZTCP resulted in the highest PR values; however, the root depth was less affected in contrast to STCP and DTCP. This might explain why roots could exploit existing pore networks in undisturbed soils compared with tillage treatments. In the middle layer examined, shallow-tillage conventional-pressure treatments suffered from a tillage pan effect, as shown in Fig. 5. In fact, all trafficked zero- and shallow-tillage systems resulted in PR values beyond 2000 kPa, which is a threshold level at which several studies have shown that there is a reduction in root growth (da Silva et al., 1994; Lapen et al., 2004; Tormena et al., 1999). A compact zone at shallow depths is detrimental to plant growth and crop yield in rainfed temperate climates when short-term droughts occur (Campbell et al., 1976).
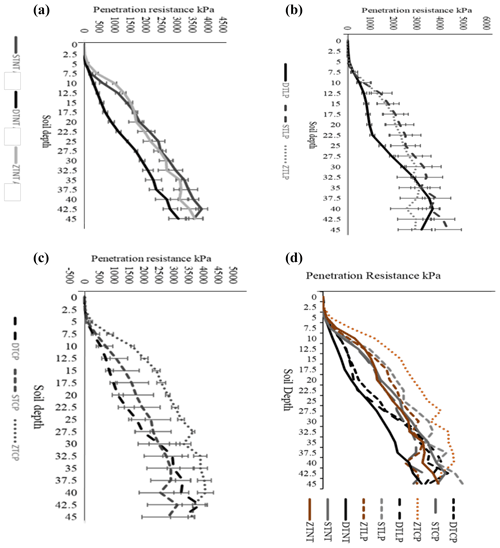
Figure 5Penetration resistance (kPa) for tillage and traffic treatments at soil depths of 0–450 mm. The x axis depicts soil depth, whereas the y axis depicts soil penetration resistance (kPa). Treatments are represented by initials. For tillage, the abbreviations for the treatments are D – deep, S – shallow, and Z – zero, while the abbreviated treatments for traffic are NT – no traffic, LP – low-pressure tyre, and CP – conventional-pressure tyre. Panel (a) shows no traffic, panel (b) shows low tyre pressure, panel (c) shows conventional tyre pressure, and panel (d) shows the traffic × tillage treatments combined.
4.1.6 Root system architecture responses to traffic
Traffic significantly affected root volume, root surface area, and root length. Moreover, when comparing the highest root biomass (under deep tillage with no traffic) and bulk density results in the 100–200 mm layer, we found a reduction in root biomass when trafficked under conventional pressure by 28 % in deep-tillage treatments (bulk density = 1.66 g cm−3), 37 % in shallow-tillage treatments (1.437 g cm−3), and 39 % in zero-tillage treatments (1.583 g cm−3). The compaction effects of traffic on the soil structure exacerbated the impact on rooting in general. Typically, studies report shallower rooting, increases in root diameter, and decreased axial and lateral rooting (Grzesiak et al., 2014). Shallow tillage had the lowest root biomass in both the trafficked and un-trafficked treatments. Shallow-tillage treatments suffered from visible horizontal fissures or “tillage pan” (Fig. 5), causing significantly reduced rooting compared with deep-tillage treatments. Moreover, with a combination of < 10 % porosity and PR reaching > 2000 kPa in the 100–200 mm layer, it is likely that roots may have also suffered from anaerobic conditions due to poor infiltration rates through the tillage pan during heavy rainfall events. Conversely, root impedance may have occurred during drought periods through May and June (Batey, 2009). Alameda et al. (2012) proposed that axial growth suffers more than radial root growth. These effects of increased PR and soil bulk density were observed in the current study; however, the increase in root diameter reported by several authors was not detected here (Chen et al., 2014; Lipiec et al., 2012; Tracy et al., 2012; Alameda et al., 2012).
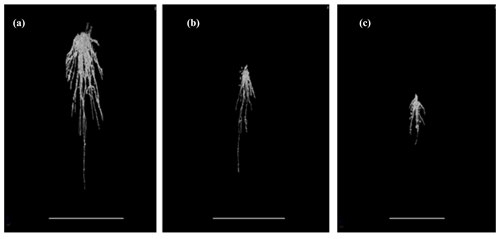
Figure 6The root system architecture of winter wheat during anthesis for (a) deep-tillage no-traffic systems, (b) zero-tillage low-tyre-pressure systems, and (c) deep-tillage conventional-tyre-pressure systems. Panels (a) and (b) show a significantly longer root length on the primary axis compared with the deep-tillage trafficked treatments shown in panel (c). The scale bar represents 70 mm.
4.1.7 Tillage and traffic effects on rooting and crop yield
In the present study, it was found that long-term zero-tillage plots under low-tyre-pressure conditions increased yields by up to 0.772 Mt ha−1 compared with the deep-tillage conventional-tyre-pressure treatments. All zero-tillage treatments yielded over 11 Mt ha−1 compared with deep- and shallow-tillage treatments (mean of 10.71 Mt ha−1). Evidence using data collected from the X-ray CT scans showed deeper vertical rooting in zero-tillage plots compared with shallow- and deep-tillage treatments (Fig. 6). Coupled with deeper rooting, zero-tillage no-traffic treatments had a significantly lower bulk density than deep-tillage conventional-pressure plots. Muñoz-Romero et al. (2010) reported a yield increase of 0.5 Mt ha−1 in zero-tillage systems compared with conventional tillage which was associated with greater water use and increased water use efficiency, similar to Chakraborty et al. (2008). Improvements in moisture retention and soil pore structures as well as reduced soil compaction under zero-tillage conditions may also have contributed to a yield increase over conventionally tilled treatments.
It is possible that the lower levels of porosity found in zero-tillage treatments aided with water retention during drought periods in the highly sandy soil in this trial. Coupled with the development of vertically oriented soil structural characteristics attributed to earthworm activity and old root channels (Fig. 4), the zero-tillage treatments may also have had increased root access to water at lower soil horizons. Indeed, biopores benefit root growth by altering the surrounding chemical, physical, and biological properties of soil (Stroud et al., 2017; Banfield et al., 2017), thereby providing macropore pathways with lower mechanical resistance which deeper roots preferentially grow towards (Zhou et al., 2021). In contrast, deep cultivation created a porous structure that has been shown to increase the respiration of aerobic microorganisms, improving the flow of air and water and, thus, increasing CO2 emissions (Mangalassery et al., 2014a). Crop yield was less influenced by traffic in zero-tillage treatments than in the other tillage treatments. The lower sensitivity to compaction in zero-tillage treatments is attributed to an elastic behaviour or increase in the bearing capacity, with soil acquiring similar structural properties to grassland soil (Ehlers and Claupein, 1994).
4.2 Using 2D and 3D imaging to study root–soil relationships
Due to the complexity of measuring root systems, two methods were conducted to provide a comprehensive analysis. Important topological (root networks) and geometrical (physical positions) characteristics of wheat rooting were found in this study using X-ray CT. A strong significant relationship between RLD (WinRHIZO™) and root depth (X-ray CT) was uncovered (Fig. S4), thereby validating the suitability of image analysis methods in field studies. Further, root depth showed the strongest correlation with crop yield compared with root biomass and RLD (Fig. S5). Moreover, the large environmental variance (low r number) in root relationships may have been caused by the spatial effects reported in previous studies (Guo et al., 2020; Zhou et al., 2021). Visualizing important behaviours of wheat rooting in field-scale trials highlights the significance of root depth with respect to sustaining high yields under drought conditions. Figure 6 depicts a significantly longer root length in zero-tillage treatments compared with trafficked deep and shallow tillage; in trafficked treatments, roots were generally confined to the top 0–50 mm of soil. In general, the root length rarely surpassed 100 mm in depth. This was partly due to the insufficient resolution available with the X-ray CT scanner with respect to capturing the finer root materials (Pfeifer et al., 2015).
In general, both root analysis methods showed agreement in the results. Zero-tillage treatments had significantly deeper rooting over shallow-tillage and deep-tillage trafficked treatments. Using the WinRHIZO™ method, un-trafficked deep-tillage treatments showed superior root length. Similar disagreements in findings between methods could be explained by the difference in methodology between the two imaging approaches: X-ray CT is 3D and scans roots in soil, whereas WinRHIZO™ is 2D and scans washed roots (Tracy et al., 2012). Root volume and surface area were also examined using X-ray CT. In contrast to the WinRHIZO™ analysis, no significant differences could be detected between treatments. The root volumes obtained by WinRHIZO™ were much greater than the volumes observed from the X-ray CT scan. This difference can be attributed to much clearer contrasts between air and root material when using the destructive method compared with the resolution limitations and density differences between soil, root, and organic materials (Mooney et al., 2012) in the X-ray CT scan images.
The results from this research highlight the importance of traffic management for improving crop productivity. Physical and visual implications of soil compaction on the soil profile were demonstrated in this study, signifying the implications of tyre pressure on root growth. Traffic significantly reduced root development in all tillage treatments, with tyre pressure having no significant effect on mitigating compaction effects on soil and roots. Moreover, deep- and shallow-tillage systems were more influenced by compaction than zero-tillage treatments with roots confined to the top 0–60 mm, reducing primary vertical rooting and inhibiting root access to deeper soil moisture reserves. The highly significant impact on crop yield was highlighted by the strong relationship between root depth and crop yield. The visible effects of traffic on the soil profile, depicted through X-ray CT, provide evidence of the damage modern farm machinery can cause with respect to root resource capture, leading to potential increased drought stress and yield loss in crop production. This long-term trial site has shown that zero tillage does not affect root growth; in fact, zero-tillage treatments reduced bulk density and improved grain yield and rooting depth significantly through deeply connected vertical soil pore fissures created by earthworms and old root channels, allowing roots to access deeper soil moisture reserves. These findings suggest that scientists and farmers should focus on designing improved zero-tillage cropping systems and managing field traffic protocols with controlled-traffic farming. Further investigation into tracks and dual-radial tyres is also required in order to quantify practical compaction mitigation measures. Moreover, this research shows that the combination of X-ray CT scanning and traditional destructive methods provides a robust method for assessing field rooting for future crop breeding initiatives and soil management practice. This research concludes that traffic has more profound effects for root growth and crop yield than the tillage method.
The datasets are available upon request by emailing the corresponding author directly.
The supplement related to this article is available online at: https://doi.org/10.5194/soil-8-391-2022-supplement.
KM and SRT conceived the experiment. DH and MH carried out sampling and soil analysis. DH processed and analysed all samples. DH analysed and interpreted the data and wrote the paper. All authors contributed to the data interpretation and provided comments on the paper.
The contact author has declared that neither they nor their co-authors have any competing interests.
Publisher's note: Copernicus Publications remains neutral with regard to jurisdictional claims in published maps and institutional affiliations.
This research took samples from a long-term tillage and traffic experiment site at Harper Adams University. Long-term crop rotation treatments at the Large Marsh site on the University grounds are managed and maintained by the agricultural staff at the university.
This research has been supported by the Science Foundation Ireland (grant no. 16/SPP/3296).
This paper was edited by Engracia Madejón Rodríguez and reviewed by two anonymous referees.
AHDB: Wheat growth guide, AHDB Cereals & Oilseeds, Stoneleigh Park, Kenilworth, Warwickshire, CV8 2TL, Agriculture and Horticulture development board, https://ahdb.org.uk/knowledge-library/wheat-growth-guide (last access: 24 May 2022), 2018.
Akker, J. J. H. V. D. and Canarache, A.: Two European concerted actions on subsoil compaction, Wageningen Environ. Res., 42, 15–22, 2001.
Alameda, D., Anten, N. P. R., and Villar, R.: Soil compaction effects on growth and root traits of tobacco depend on light, water regime and mechanical stress, Soil Till. Res., 120, 121–129, 2012.
Angers, D. A. and Caron, J.: Plant-induced Changes in Soil Structure: Processes and Feedbacks, Biogeochemistry, 42, 55–72, 1998.
Arvidsson, J.: Influence of soil texture and organic matter content on bulk density, air content, compression index and crop yield in field and laboratory compression experiments, Soil Till. Res., 49, 159–170, https://doi.org/10.1016/S0167-1987(98)00164-0, 1998.
Arvidsson, J.: Direct drilling possible with a good preceding crop, Swedish Rural Econ. Agr. Soc., 4, 9–10, 2010.
Arvidsson, J. and Keller, T.: Soil stress as affected by wheel load and tyre inflation pressure, Soil Till. Res., 96, 284–291, https://doi.org/10.1016/j.still.2007.06.012, 2007.
Atkinson, J. A., Hawkesford, M. J., Whalley, W. R., Zhou, H., and Mooney, S. J.: Soil strength influences wheat root interactions with soil macropores, Plant Cell Environ., 43, 235–245, https://doi.org/10.1111/pce.13659, 2020.
Bacq-Labreuil, A., Crawford, J. W., Mooney, S., Neal, A. L., Akkari, E., Mcauliffe, C., Zhang, X. X., Redmile-Gordon, M. A., and Ritz, K.: Effects of cropping systems upon the three-dimensional architecture of soil systems are modulated by texture, Geoderma, 332, 73–83, 2018.
Banfield, C. C., Dippold, M. A., Pausch, J., Hoang, D. T., and Kuzyakov, Y.: Biopore history determines the microbial community composition in subsoil hotspots, Biol. Fert. Soils, 53, 573–588, 2017.
Batey, T.: Soil compaction and soil management – a review, Soil Use Manag., 25, 335–345, https://doi.org/10.1111/j.1475-2743.2009.00236.x, 2009.
Bengough, A. G.: Water Dynamics of the Root Zone: Rhizosphere Biophysics and Its Control on Soil Hydrology, Vadose Zone J., 11, 2, https://doi.org/10.2136/vzj2011.0111, 2012.
Bengough, A. G., McKenzie, B. M., Hallett, P. D., and Valentine, T. A.: Root elongation, water stress, and mechanical impedance: a review of limiting stresses and beneficial root tip traits, J. Exp. Bot., 62, 59–68, https://doi.org/10.1093/jxb/erq350, 2011.
Boguzas, V. and Hakansson, I.: Barley yield losses simulation under Lithuanian conditions using the Swedish soil compaction model, Soil management department, Lithuanian University of Agriculture, CABI, CAB Direct, 24–28, https://www.cabdirect.org/cabdirect/abstract/20013143017 (last access: 24 May 2022), 2001.
Boguzas, V., Kairyte, A., Jodaugiene, D., and Lukosiunas, K.: Effect of reduced and no-tillage, straw and green manure management on soil physical properties and earthworms, Agronomy, 35, 1566–1577, 2006.
Botta, G. F., Rivero, D., Tourn, M., Bellora Melcon, F., Pozzolo, O., Nardon, G., Balbuena, R., Tolon Becerra, A., Rosatto, H., and Stadler, S.: Soil compaction produced by tractor with radial and cross-ply tyres in two tillage regimes, Soil Till. Res., 101, 44–51, https://doi.org/10.1016/j.still.2008.06.001, 2008.
Campbell, D. J. and Henshall, J. K.: Bulk density, Soil & environment Analysis: Physical Methods, 2nd Edn., edited by: Smith, K. A., 315–348, Revised & Expanded, https://books.google.ie/books?hl=en&lr=&id=lGMFYp2CA2EC&oi=fnd&pg=PA315&dq=Campbell,+D.+J.+and+Henshall,+J.+K.:+Bulk+density,+&ots=DfzKp6YHGM&sig=R6eBCiV448fig2POxUNb6bVajZk&redir_esc=y#v=onepage&q=Campbell%2C%20D.%20J.%20and%20Henshall%2C%20J.%20K.%3A%20Bulk%20density%2C&f=false (last access: 26 May 2022), 2000.
Campbell, R. B., Reicosky, D. C., and Doty, C. W.: Corn Plant Water Stress as Influenced by Chiseling, Irrigation, and Water Table Depth, Agron. J., 68, 499–503, https://doi.org/10.2134/agronj1976.00021962006800030015x, 1976.
Chakraborty, D., Nagarajan, S., Aggarwal, P., Gupta, V. K., Tomar, R. K., Garg, R. N., Sahoo, R. N., Sarkar, A., Chopra, U. K., Sundara Sarma, K. S., and Kalra, N.: Effect of mulching on soil and plant water status, and the growth and yield of wheat (Triticum aestivum L.) in a semi-arid environment, Agr. Water Manage., 95, 1323–1334, 2008.
Chamen, T.: Controlled traffic farming on a field scale in the UK, Soil management for sustainability, Adv. Geoecol., 38, 251–260, 2006.
Chamen, W. C. T.: The effects of low and controlled traffic systems on soil physical properties, yields and the profitability of cereal crops on different soil types, PhD, Cranfield University, http://dspace.lib.cranfield.ac.uk/handle/1826/7009 (last access: 24 May 2022), 2011.
Chan, K. Y., Oates, A., Swan, A. D., Hayes, R. C., Dear, B. S., and Peoples, M. B.: Agronomic consequences of tractor wheel compaction on a clay soil, Soil Till. Res., 89, 13–21, 2006.
Chopra, U. K., Sundara Sarma, K. S., and Kalra, N.: Effect of mulching on soil and plant water status, and the growth and yield of wheat (Triticum aestivum L.) in a semi-arid environment, Agr. Water Manage., 95, 1323–1334, https://doi.org/10.1016/j.agwat.2008.06.001, 2008.
Christian, D. G. and Ball, B. C.: Reduced cultivations and direct drilling for cereals in Great Britain, Conservation Tillage in Temperate Agroecosystems, Boca Raton, Florida, USA, Lewis Publishers, 117–140, ISBN 978-1-31515-0-529, 1994.
Chyba, J.: The influence of traffic intensity and soil texture on soil water infiltration rate, Msc, Harper adams University, Newport, UK, https://hau.repository.guildhe.ac.uk/id/eprint/17410 (last access: 24 May 2022), 2012.
Cooper, H. V., Sjogersten, S., Lark, R. M., and Mooney, S. J.: To till or not to till in a temperate ecosystem? Implications for climate change mitigation, Environ. Res. Lett., 16, 054022, https://doi.org/10.1088/1748-9326/abe74e, 2021.
da Silva, A. P., Kay, B. D., and Perfect, E.: Characterization of the Least Limiting Water Range of Soils, Soil Sci. Soc. Am. J., 58, 1775–1781, 1994.
Cranfield University: The Soils Guide, Cranfield University, UK, http://www.landis.org.uk, last access: 22 November 2021.
Czyż, E. A.: Effects of traffic on soil aeration, bulk density and growth of spring barley, Soil Till. Res., 79, 153–166, https://doi.org/10.1016/j.still.2004.07.004, 2004.
da Silva, A. P., Kay, B. D., and Perfect, E.: Characterization of the Least Limiting Water Range of Soils, Soil Sci. Soc. Am. J., 58, 1775–1781, 1994.
Defossez, P. and Richard, G.: Models of soil compaction due to traffic and their evaluation, Soil Till. Res., 67, 41–64, 2002.
Doussan, C., Pierret, A., Garrigues, E., and Pages, L.: Water Uptake by Plant Roots: II – Modelling of Water Transfer in the Soil Root-system with Explicit Account of Flow within the Root System – Comparison with Experiments, Plant Soil, 283, 99–117, https://doi.org/10.1007/s11104-004-7904-z, 2006.
Ehdaie, B., Merhaut, D. J., Ahmadian, S., Hoops, A. C., and Khuong, T.: Root System Size Influences Water-Nutrient Uptake and Nitrate Leaching Potential in Wheat, J. Agron. Crop Sci., 196, 455–466, https://doi.org/10.1111/j.1439-037X.2010.00433.x, 2010.
Ehlers, W. and Claupein, W.: Approaches Towards Conservation Tillage in Germany, Conservation Tilage in Temperate Agroecosystems, 1st Edn., CRC Press, p. 25, ISBN 978-1-31515-0-529, 1994.
Flavel, R. J., Guppy, C. N., Rabbi, S. M. R., and Young, I. M.: An image processing and analysis tool for identifying and analysing complex plant root systems in 3D soil using non-destructive analysis: Root1, PloS ONE, 12, e0176433, https://doi.org/10.1371/journal.pone.0176433, 2017.
Gee, G. W. and Or, D.: Particle-Size Analysis, Methods of Soil Analysis: Part 4 Physical Methods, 5.4, edited by: Dane, J. H. and Topp, G. C., Soil Society of America, 255–293, https://doi.org/10.2136/sssabookser5.4.c12, 2002.
Gregory, P. J., McGowan, M., Biscoe, P. V., and Hunter, B.: Water relations of winter wheat: 1. Growth of the root system, J. Agr. Sci., 91, 91–102, https://doi.org/10.1017/S0021859600056653, 1978.
Grzesiak, M. T., Ostrowska, A., Hura, K., Rut, G., Janowiak, F., Rzepka, A., Hura, T., and Grzesiak, S.: Interspecific differences in root architecture among maize and triticale genotypes grown under drought, waterlogging and soil compaction, Acta Physiol. Plant, 36, 3249–3261, https://doi.org/10.1007/s11738-014-1691-9, 2014.
Guo, X., Svane, S. F., Füchtbauer, W. S., Andersen, J. R., Jensen, J., and Thorup-Kristensen, K.: Genomic prediction of yield and root development in wheat under changing water availability, Plant Method., 16, 90, https://doi.org/10.1186/s13007-020-00634-0, 2020.
Hamblin, A. P. and Tennant, D.: Root length density and water uptake in cereals and grain legumes: how well are they correlated, Austr. J. Agr. Res., 38, 513–527, https://doi.org/10.1071/AR9870513, 1987.
Hamza, M. A. and Anderson, W. K.: Soil compaction in cropping systems: A review of the nature, causes and possible solutions, Soil Till. Res., 82, 121–145, https://doi.org/10.1016/j.still.2004.08.009, 2005.
Himmelbauer, M. L., Loiskandl, A. W., and Kastanek, A. F.: Estimating length, average diameter and surface area of roots using two different Image analyses systems, Plant Soil, 260, 111–120, https://doi.org/10.1023/B:PLSO.0000030171.28821.55, 2004.
Ishaq, M., Hassan, A., Saeed, M., Ibrahim, M., and Lal, R.: Subsoil compaction effects on crops in Punjab, Pakistan: I. Soil physical properties and crop yield, Soil Till. Res., 59, 57–65, https://doi.org/10.1016/S0167-1987(00)00189-6, 2001.
Javaux, M., Couvreur, V., Vanderborght, J., and Vereecken, H.: Root Water Uptake: From Three-Dimensional Biophysical Processes to Macroscopic Modeling Approaches, Vadose Zone J., 12, 4, https://doi.org/10.2136/vzj2013.02.0042, 2013.
Kaczorowska-Dolowy, M., Godwin, R. J., Dickin, E., White, D. R., and Misiewicz, P. A.: Controlled traffic farming delivers better crop yield of winter bean as a result of improved root development, Agronomy Res., 17, 725–740, https://doi.org/10.15159/ar.19.136, 2019.
Lal, R.: Beyond Copenhagen: mitigating climate change and achieving food security through soil carbon sequestration, Food Secur., 2, 169–177, 2010.
Lapen, D. R., Topp, G. C., Gregorich, E. G., and Curnoe, W. E.: Least limiting water range indicators of soil quality and corn production, eastern Ontario, Canada, Soil Till. Res., 78, 151–170, https://doi.org/10.1016/j.still.2004.02.004, 2004.
Lipiec, J., Horn, R., Pietrusiewicz, J., and Siczek, A.: Effects of soil compaction on root elongation and anatomy of different cereal plant species, Soil Till. Res., 121, 74–81, https://doi.org/10.1016/j.still.2012.01.013, 2012.
Lynch, J. P. and Brown, K. M.: Topsoil foraging – an architectural adaptation of plants to low phosphorus availability, Plant Soil, 237, 225–237, 2001.
Mairhofer, S., Pridmore, T., Johnson, J., Wells, D. M., Bennett, M. J., Mooney, S. J., and Sturrock, C. J.: X-ray computed Tomography of Crop Plant Root Systems Grown in Soil, Current Protocols in Plant Biology, 2, 270–286, https://doi.org/10.1002/cppb.20049, 2017.
Mangalassery, S., Sjogersten, S., Sparkes, D. L., Sturrock, C. J., Craigon, J., and Mooney, S. J.: To what extent can zero tillage lead to a reduction in greenhouse gas emissions from temperate soils?, Sci. Rep., 4, 4586, https://doi.org/10.1038/srep04586, 2014.
Met Office: National meteorological Library & Archive, Met Office, UK, 2019.
Millington, W. A., Misiewicz, P., White, D., Dickin, E., Mooney, S. J., and Godwin, R. J.: An investigation into the effect of traffic and tillage on soil properties using X-ray computed tomography, 2017 ASABE Annual International Meeting, p. 1, https://doi.org/10.13031/aim.201700380, 2017.
Mooney, S. J., Pridmore, T. P., Helliwell, J., and Bennett, M. J.: Developing X-ray Computed Tomography to non-invasively image 3-D root systems architecture in soil, Plant Soil, 352, 1–22, 2012.
Morris, E. C., Griffiths, M., Golebiowska, A., Mairhofer, S., Burr-Hersey, J., Goh, T., von Wangenheim, D., Atkinson, B., Sturrock, C. J., Lynch, J. P., Vissenberg, K., Ritz, K., Wells, D. M., Mooney, S. J., and Bennett, M. J.: Shaping 3D Root System Architecture, Curr. Biol., 27, R919–R930, 2017.
Morris, N. L., Miller, P. C. H., Orson, J. H., and Froud-Williams, R. J.: The adoption of non-inversion tillage systems in the United Kingdom and the agronomic impact on soil, crops and the environment – A review, Soil Till. Res., 108, 1–15, 2010.
Muñoz-Romero, V., Benitez-Vega, J., Lopez-Bellido, R. J., Fontan, J. M., and Lopez-Bellido, L.: Effect of tillage system on the root growth of spring wheat, Plant Soil, 326, 97–107, 2010.
Naderi-Boldaji, M., Kazemzadeh, A., Hemmat, A., Rostami, S., and Keller, T.: Changes in soil stress during repeated wheeling: A comparison of measured and simulated values, Soil Res., 56, 204–214, https://doi.org/10.1071/SR17093, 2017.
Otto, R., Silva, A. P., Franco, H. C. J., Oliveira, E. C. A., and Trivelin, P. C. O.: High soil penetration resistance reduces sugarcane root system development, Soil Till. Res., 117, 201–210, https://doi.org/10.1016/j.still.2011.10.005, 2011.
Pfeifer, J., Kirchgessner, N., Colombi, T., and Walter, A.: Rapid phenotyping of crop root systems in undisturbed field soils using X-ray computed tomography, Plant Method., 11, 41, https://doi.org/10.1186/s13007-015-0084-4, 2015.
Pires, L. F., Borges, J. A. R., Rosa, J. A., Cooper, M., Heck, R. J., Passoni, S., and Roque, W. L.: Soil structure changes induced by tillage systems, Soil Till. Res., 165, 66–79, https://doi.org/10.1016/j.still.2016.07.010, 2017.
Rab, M. A., Haling, R., Aarons, S., Hannah, M., Young, I., and Gibson, D.: Evaluation of X-ray computed tomography for quantifying macroporosity of loamy pasture soils, Geoderma, 213, 460–470, https://doi.org/10.1016/J.GEODERMA.2013.08.037, 2014.
Raper, R. L.: Agricultural traffic impacts on soil, J. Terramechanics, 42, 259–280, https://doi.org/10.1016/j.jterra.2004.10.010, 2005.
Reicosky, D. C., Reeves, D. W., Prior, S. A., Runion, G. B., Rogers, H., and Raper, R. L.: Effects of residue management and controlled traffic on carbon dioxide and water loss, Soil Till. Res., 52, 153–165, https://doi.org/10.1016/S0167-1987(99)00065-3, 1999.
Ren, L., Vanden Nest, T., Ruysschaert, G., D'Hose, T., and Cornelis, W. M.: Short-term effects of cover crops and tillage methods on soil physical properties and maize growth in a sandy loam soil, Soil Till. Res., 192, 76–86, https://doi.org/10.1016/j.still.2019.04.026, 2019.
Schneider, C. A., Rasband, W. S., and Eliceiri, K. W.: NIH Image to ImageJ: 25 years of image analysis, Nat. Methods, 9, 671–675, https://doi.org/10.1038/nmeth.2089, 2012.
Schulte, R. P. O., Diamond, J., Finkele, K., Holden, N. M., and Brereton, A. J.: Predicting the Soil Moisture Conditions of Irish Grasslands, Irish J. Agr. Food Res., 44, 95–110, https://www.jstor.org/stable/25562535 (last access: 24 May 2022), 2005.
Six, J., Ogle, S. M., Breidt, F. J., Conant, R. T., Mosier, A. R., and Paustian, K.: The potential to mitigate global warming with no-tillage management is only realized when practised in the long term, Glob. Change Biol., 10, 155–160, https://doi.org/10.1111/j.1529-8817.2003.00730.x, 2004.
Skaalsveen, K., Ingram, J., and Clarke, L. E. : The effect of no-till farming on the soil functions of water purification and retention in north-western Europe: a literature review, Soil Till. Res., 189, 98–109, https://doi.org/10.1016/j.still.2019.01.004, 2019.
Smith, E.: The effect of agricultural traffic and tillage on soil physical properties and crop yields: A thesis submitted in partial fulfilment of the requirements for the degree of Doctor of Philosophy, Newport: Harper Adams University, PhD, Harper Adams University, Newport, https://hau.repository.guildhe.ac.uk/id/eprint/17337 (last access: 24 May 2022), 2016.
Soane, B. D., Blackwell, B. D., Dickson, J. W., and Painter, D. J.: Compaction by agricultural vehicles: A review II. Compaction under tyres and other running gear, Soil Till. Res., 1, 373–400, https://doi.org/10.1016/0167-1987(80)90039-2, 1980.
Soane, G. C., Godwin, R. J., and Spoor, G.: Influence of deep loosening techniques and subsequent wheel traffic on soil structure, Soil Till. Res., 8, 231–237, https://doi.org/10.1016/0167-1987(86)90336-3, 1986.
Soane, B. D., Ball, B. C., Arvidsson, J., Basch, G., Moreno, F., and Roger-Estrade, J.: No-till in northern, western and south-western Europe: A review of problems and opportunities for crop production and the environment, Soil Till. Res., 118, 66–87, https://doi.org/10.1016/j.still.2011.10.015, 2012.
Stroud, J. L., Irons, D. E., Watts, C. W., Storkey, J., Morris, N. L., Stobart, R. M., Fielding, H. A., and Whitmore, A. P.: Cover cropping with oilseed radish (Raphanus sativus) alone does not enhance deep burrowing earthworm (Lumbricus terrestris) midden counts, Soil Till. Res., 165, 11–15, https://doi.org/10.1016/j.still.2016.07.013, 2017.
Tinker, P. B. and Nye, P. H.: Solute movement in the Rhizosphere, Oxford, Oxford University press, 2000.
Tormena, C. A., da Silva, A. P., and Libardi, P. L.: Soil physical quality of a Brazilian Oxisol under two tillage systems using the least limiting water range approach, Soil Till. Res., 52, 223–232, https://doi.org/10.1016/S0167-1987(99)00086-0, 1999.
Tracy, S. R., Black, C. R., Roberts, J. A., McNeill, A., Davidson, R., Tester, M., Samec, M., Korosak, D., Sturrock, C., and Mooney, S. J.: Quantifying the effect of soil compaction on three varieties of wheat (Triticum aestivum L.) using X-ray Micro Computed Tomography (CT), Plant Soil, 353, 195–208, 2012.
Tracy, S. R., Black, C. R., Roberts, A., and Mooney, S. J.: Exploring the interacting effect of soil texture and bulk density on root system development in tomato (Solanum lycopersicum L.), Environ. Exp. Bot., 91, 38–47, https://doi.org/10.1016/j.envexpbot.2013.03.003, 2013.
Wang, M.-B. and Zhang, Q.: Issues in using the WinRHIZO system to determine physical characteristics of plant fine roots, Acta Ecol. Sin., 29, 136–138, https://doi.org/10.1016/j.chnaes.2009.05.007, 2009.
Whalley, W. R., Watts, C. W., Gregory, A. S., Mooney, S. J., Clark, L. J., and Whitmore, A. P.: The effect of soil strength on the yield of wheat, Plant Soil, 306, 237, https://doi.org/10.1007/s11104-008-9577-5, 2008.
White, C. A., Sylvester-Bradley, R., and Berry, P. M.: Root length densities of UK wheat and oilseed rape crops with implications for water capture and yield, J. Exp. Bot., 66, 2293–2303, https://doi.org/10.1093/jxb/erv077, 2015.
Wildenschild, D., Vaz, C. M. P., Rivers, M. L., Rikard, D., and Christensen, B. S. B.: Using X-ray computed tomography in hydrology: systems, resolutions, and limitations, J. Hydrol., 267, 285–297, https://doi.org/10.1016/S0022-1694(02)00157-9, 2002.
Zadoks, J. C., Chang, T. T., and Konzak, C. F.: A decimal code for the growth stages of cereals, Weed Res., 14, 415–421, https://doi.org/10.1111/j.1365-3180.1974.tb01084.x, 1974.
Zhou, H., Whalley, W. R., Hawkesford, M. J., Ashton, R. W., Atkinson, B., Atkinson, J. A., Sturrock, C. J., Bennett, M. J., and Mooney, S. J.: The interaction between wheat roots and soil pores in structured field soil, J. Exp. Bot., 72, 747–756, https://doi.org/10.1093/jxb/eraa475, 2021.