the Creative Commons Attribution 4.0 License.
the Creative Commons Attribution 4.0 License.
What comes after the Sun? On the integration of soil biogeochemical pre-weathering into microplastic experiments
Frederick Büks
Martin Kaupenjohann
Recent studies have been engaged in estimating the adverse effects of microplastic (MP) on soil quality parameters. Mass concentrations of MP, as found in highly contaminated soils, have been shown to weaken the soil structure, and parts of the edaphon are adversely affected mainly by the <100 µm MP size fraction. However, the vast majority of these studies used pristine particles, which have surface characteristics different from those of environmental MP. Exposed to UV radiation, plastic undergoes photochemical weathering with embrittlement and the formation of surface charge, leading to an alteration of physiochemical behavior. When plastic particles then enter the soil environment, further aging factors appear with yet unknown efficacy. This little explored soil biogeochemical phase includes biofilm cover, decay with enzymes (as shown in laboratory experiments with both conventional and biodegradable plastics), contact with biotic and abiotic acids, oxidants, and uptake by the soil fauna that causes physical fragmentation. Such transformation of the surfaces is assumed to affect soil aggregation processes, soil faunal health, and the transport of plastic colloids and adsorbed solubles. This perspective article encourages us to consider the weathering history of MP in soil experiments and highlights the need for reproducing the surface characteristics of soil MP to conduct laboratory experiments with closer-to-nature results.
- Article
(129 KB) - Full-text XML
- BibTeX
- EndNote
Since the mass production of plastic articles for daily use started in the early 1950s (Thompson et al., 2009), a number of processes have caused the contamination of ecosystems such as inland and coastal waters, sediments, the open and deep seas, soils, and even the atmosphere with microplastic (MP; e.g., Cole et al., 2011; Woodall et al., 2014; Wu et al., 2018; Büks and Kaupenjohann, 2020; Trainic et al., 2020). The formation of soil MP pools occurs through littering and dispersion from landfills, the application of wastewater, contaminated surface water, sewage sludge, composts, digestates, mulching foils, seed and fertilizer coatings, road dust, and atmospheric deposition (Eerkes-Medrano et al., 2015; Huerta Lwanga et al., 2017a; Weithmann et al., 2018; Corradini et al., 2019; Dierkes et al., 2019; He et al., 2019; Edo et al., 2020; Huang et al., 2020; Bertling et al, 2021; Katsumi et al., 2021; Szewc et al., 2021).
Today, we are facing a global contamination of soil ecosystems with MP that averages 1.7 mg kg−1 of dry soil in agricultures (Büks and Kaupenjohann, 2020), exceeds this value by several orders of magnitude in heavily contaminated soils at road sides and industrial areas (Fuller and Gautam, 2016; Dierkes et al., 2019), and reaches even remote areas (Abbasi et al., 2021). Several laboratory studies showed the adverse effects of high MP concentrations on the soil fauna (Büks et al., 2020a) and soil structure (e.g., de Souza Machado et al., 2018, 2019; Liang et al., 2019; Lozano et al., 2021) and underlined the relevance of especially the small-sized fraction (MP < 100 µm) (Büks et al., 2020b).
Although these results are rightly alarming due to the function of the soil structure and the edaphon as soil fertility parameters (Bronick and Lal, 2005; Thiele-Bruhn et al., 2012), their informative value is limited by the fact that the vast majority of experiments used pristine plastic and a short runtime that does not allow for further weathering (e.g., de Souza Machado et al., 2018, 2019; Liang et al., 2019; Büks et al., 2020a; Lozano et al., 2021). For a better matching with environmental conditions, some studies have used photooxidatively weathered plastic. In soil, however, the bulk of MP is additionally exposed to biogeochemical alteration for years. Its surface characteristics and role within soil ecosystems thereby possibly change compared to solely aboveground weathering.
On a microscopic scale, the surfaces of pristine plastic items are normally smooth with nearly no surface charge (e.g., Fotopoulou and Karapanagioti, 2012, 2015). Exposed to sunlight, the depletion of UV absorbers and HALS (hindered amine light stabilizers) leads to enhanced photooxidation (e.g., Kokott, 1989; Pickett, 2018). From the point of view of the macroscopic observer, the plastic becomes less hydrophobic, stiff, and more prone to fragmentation by wind and water erosion.
Standardized approaches from materials science are newly used in soil science to reproduce natural photooxidative aging characteristics (i.e., the German Federal Ministry of Education and Research, BMBF, initiative “Plastic in the Environment”; https://www.bmbf-plastik.de/en, last access: 8 April 2022; e.g., Büks et al., 2021). They recommend xenon arc lamps with borosilicate filters that adjust the emitted spectrum closer to the natural UV spectrum, or fluorescent UV lamps (see DIN EN ISO 4892-2/3, “Plastics – Methods of exposure to laboratory light sources”). The performance of these approaches is enhanced by use of modern daylight filters, a steady temperature of 38 ∘C, relative air humidity of 25 % to 50 %, and regular washing of the sample surfaces by artificial rain (Pickett, 2018). Besides the use of UV, γ irradiation treatment is reported to imitate the carbonyl stretch in PE samples similar to a long-term UVB exposition (Johansen et al., 2019). Furthermore, Zhou et al. (2020) could demonstrate that discharged plasma oxidation (DPO) is likewise suitable for increasing the surface area, crystallinity, and carbonyl indices of plastic particles within hours.
However, it is unknown whether these protocols properly reproduce the additional influence of underground aging, which occurs under different physiochemical conditions. When plastic is exposed to the dark world of soil fauna, microorganisms, roots, and frequent leaching, the composition of weathering parameters changes significantly (Table 1). The plastic is now faced to new mechanical stresses such as (bio-)turbation, largely moist conditions, and is exposed to a variety of soil biogeochemical processes.
One of these potential aging factors is the diverse and active soil fauna that has been shown to ingest, digest, and excrete plastic particles (Büks et al., 2020). It is an ensemble of small, mobile bioreactors that incubate soil particles, including MP, within a habitat of high microbial diversity – their gastrointestinal tract – and distribute them throughout the soil by excretion. A well-known example for this multifaceted functionality is the earthworm. Some taxa like woodlice, termites, mealworms, and earthworms have been additionally found to comminute plastic by gnawing and, hence, actively produce MP (e.g., Lenz et al., 2012; Zhang et al., 2018; Büks et al., 2020a). There are also indications that the mealworm microbiome is able to degrade not only additives but also PE and PS polymers (e.g., Brandon et al., 2018).
While moisture evaporates quickly on sun-exposed, heated plastic surfaces, in soils it is the ubiquitous condition for microbial life, extracellular metabolic processes, and the release and transport of chemical agents that react with the plastic outside the fauna. Microbial colonization and biofilm formation on surfaces of MP particles have been shown in studies on various aquatic ecosystems (e.g., Zettler et al., 2013; McCormick et al., 2014; Oberbeckmann et al., 2015; Dussud et al., 2018; Jiang et al., 2018). Recent studies on soil ecosystems have also demonstrated that MP surfaces of different origin are covered with microbial communities. This could hypothetically cause a masking of plastic surface characteristics by the biofilm matrix. The composition of surface MP communities is very different from that of the soil matrix (Chai et al., 2020; Zhang et al., 2019). The altered soil microbial community is thereby not only determined by the physiochemical properties of the surrounding soil but also by the type of plastic and its additives (Chai et al., 2020; Ng et al., 2020; Wang et al., 2020; Wiedner and Polifka, 2020; Yan et al., 2020; Yi et al., 2020). This might lead to a physiochemical behavior of plastic particles that differs not because of the plastic type but because of its biofilm cover.
Although plastic is unlikely to serve as a major substrate for microbes due to its large molecular size, high chemical stability, and low bioavailability (Oberbeckmann and Labrenz, 2020), there is indication that biofilm cover causes the alteration of its chemical properties. Not only acting as a viscous matrix that protects bacteria against mechanical stress, predators, desiccation, and irradiation, biofilm is also an extracellular reaction space that facilitates the concentration and metabolization of nutrients and the recycling of dead cell material (Flemming and Wingender, 2010). For this purpose, manifold extracellular enzymes are produced by the biofilm community to decompose food sources or modify the biofilm matrix in face of, e.g., oxygen or nutrient gradients (Flemming and Wingender, 2010). Among these are esterases, proteases, and amidases that target substrates like polysaccharides, proteins, extracellular DNA, lipids, and urea but also allow the (co)metabolization of artificial polymers such as diverse polyesters, ester-based PU, and PET in laboratory experiments (Shimao, 2001; Wei and Zimmermann, 2017; Danso et al., 2019).
Given a poor biodegradability of polymers with C–C backbones and no hydrolyzable functional groups such as pristine PE, PP, PS, and PVC, laboratory experiments showed an unexpected degradation of PE by a bacterial alkane hydroxylase (Yoon et al., 2012) and, beyond this, the specific targeting of PET with a bacterial PETase (Yoshida et al., 2016). In contrast, neither degrading enzymes nor observed biodegradation have been reported in the case of PP and PVC (Danso et al., 2019). Unspecific lignin-degrading enzymes such as laccases, manganese peroxidases, hydroquinone peroxidases, and lignin peroxidases produced by actinomycetes and other bacteria, as well as fungi, have been further shown to depolymerize even plastics such as PE, PS, and PA that are considered recalcitrant (Bhardwaj et al., 2013; Wei and Zimmermann, 2017). Beside the direct proof of enzymatic degradation pathways, there are numerous references on the metabolization of (bio)plastic samples by bacterial and fungal strains (e.g., Bhardwaj et al., 2013; Kale et al., 2015; Raziyafathima et al., 2016; Roohi et al., 2017). However, since many studies have applied commercial polymers that have concealed compositions (Danso et al., 2019), there is often poor insight to what degree the measured mass loss is caused by microbial/enzymatic decomposition of the polymer or additives. These findings imply that biodegradation of plastic surfaces in soil is conceivable.
Table 1Development of surface characteristics during the three phases of aging (pristine, photooxidative, and soil biogeochemical phase). Data of soil biogeochemical weathering are only known from aquatic systems. The question mark (?) indicates assumptions based on soil biogeochemical processes found in soils. Some references are from 1 Fotopoulou and Karapanagioti (2012), 2 Fotopoulou and Karapanagioti (2015), 3 ter Halle et al. (2017), 4 Dong et al. (2020), 5 Pickett (2018), 6 Andrady et al. (1993).
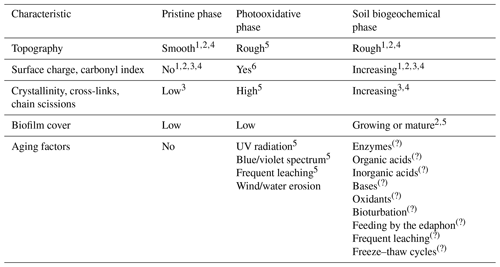
Table 2Approaches of surface (pre-)weathering in recent experiments with soil microplastic. The abbreviations used in this table are as follows: UV – ultraviolet; TBBPA – tetrabromobisphenol A; FE – feeding experiment; BD – biodegradable plastic; OP – oxo-degradable plastic, PA – polyamide; PE – polyethylene; PO – polyolefin; PP – polypropylene; PVC – polyvinyl chloride; TCE – thermoplastic copolyester elastomer. NA denotes that information was not available.
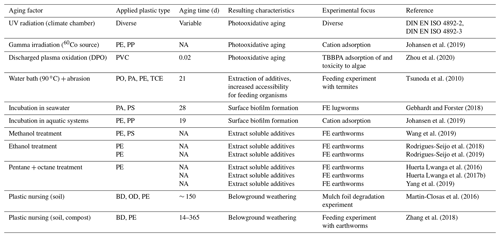
Beside the soil biome, soil-born acids, bases, and oxidants are expected to directly influence the belowground alteration of plastic surfaces. While – to the best of our knowledge – there has been no systematic examination of the effects of such agents within natural ranges of concentration and time of exposure, the treatment of plastic with highly concentrated reagents caused damaging effects from color leaching and expansion to total dissolution (Enders et al., 2017). However, pre- and post-treatment with oxidants such as H2O2 are common parts of the extraction of MP from soil samples with density fractionation (Büks and Kaupenjohann, 2020).
In winter, when the biotic effects are reduced, freeze–thaw cycles might be an additional factor of fragmentation. Studies on the effect of alternating freezing and thawing on the structure of plastic surfaces are sparse and only focus on composite materials that include non-plastic components (Wang et al., 2007; Adhikary et al., 2009; Zhou et al., 2014). However, water that has entered cracks of brittle plastic most likely contributes to its fragmentation through freezing and expansion and, thus, increases the surface area exposed to other aging factors.
While plastics are considered persistent, the above in vitro experiments indicate that degradation in soil is possible. The difference in factors leading to photooxidative and biochemical weathering makes it plausible for MP surface characteristics to develop differently in above- and belowground environments. If the physicochemical behavior of the microplastic is significantly affected by this, then the effects must be considered in the design of laboratory and field experiments. The focus on surfaces is particularly important in studies on (1) soil aggregation and structure that strongly depend on biofilm cover and surface charge/polarity of the involved primary particles, (2) adverse effects on the soil fauna that might be influenced by particle shape and sorption of (in)organic pollutants, (3) interactions with plants and microorganisms, and (4) the transport of colloidal MP within the soil pore space.
However, there are currently no studies that evaluate the efficacy of specific soil biogeochemical aging mechanisms. Recent work only showed the alteration of plastic surfaces during environmental weathering, indicating that future experiments have to be conducted with pre-weathered instead of pristine MP. It is still an open question as to whether there is effective soil biogeochemical aging beyond the photooxidative phase or whether the DIN EN ISO 4892-2/3 approach, as applied in recent work, is sufficient to imitate soil weathering conditions in future studies.
Only a few studies have integrated soil biogeochemical factors into pre-weathering approaches of artificial MP so far (Table 2; Büks et al., 2020a), which are, alas, fragmentary, heterogeneous, and often directly applied to pristine plastic. Tsunoda et al. (2010) heated plastic items within a water bath at 90 ∘C for 3 weeks and abraded the surface prior to feeding experiments with termites. This treatment attempted to make the surface more accessible for gnawing and also to extract soluble additives from the pristine plastic. In another experiment, the formation of biofilms on MP surfaces was induced by 4 weeks of incubation in seawater to make the material more attractive as a food source for the lugworm Arenicola marina (Gebhardt and Forster, 2018), an approach that can be likewise applied with soil solution. In order to remove soluble substances and fine particles from artificial MP, pristine plastics have been treated with organic solvents (Huerta Lwanga et al., 2016, 2017b; Rodrigues-Seijo et al., 2018, 2019; Wang et al., 2019; Yang et al., 2019). If the plastic type is prone to the solvents, the surface is roughened by the dissolution of oligomers and, thus, increased. However, these techniques are not assumed to increase the number of carbonyl groups and surface charge. Therefore, they do not change the interaction with the soil matrix and the soil fauna and have never been tested for the similarity to natural weathering.
In contrast, some authors avoided artificial aging and instead applied natural weathering over shorter periods between 2 weeks and 12 months, which can be used as a kind of plastic breeding (e.g., Martin-Closas et al., 2016; Zhang et al., 2018). This treatment changes the physiochemical characteristics of plastics similar to environmental short-term weathering belowground and is suitable for the alteration of large amounts of plastic. But, it is very costly in terms of time when the production of strongly weathered MP is needed.
Once we know the important biogeochemical aging factors, long-term weathering experiments will be extremely helpful to understand the dynamics of surface alteration of soil MP. These experiments must take into account not only ecosystem parameters (e.g., humidity, edaphon activity, and soil organic carbon) and starting conditions such as plastic type, particle surface, and protection by specific additives. The increase in surface area and charge density over time might cause a non-linear aging, while the biofilm cover cloaks the real MP surface characteristics – issues that should also be carefully included in the experimental design.
There is a great incentive to develop pre-weathering approaches to create designer MP for laboratory experiments. Those closer-to-nature weathering protocols might contain full chains of aboveground and in-soil aging factors and can be diversified according to actual material and environmental conditions. When applied to coming experiments, they will help us to better understand and predict the short- and long-term effects of soil MP, the concentration of which is the result of decades of contamination and is still increasing.
All of the data are published within this paper.
FB developed the article concept, collected data, and prepared the paper. MK supervised the study by participating in structural discussions on the idea and concept of the paper and the final corrections.
The contact author has declared that neither they nor their co-author has any competing interests.
Publisher’s note: Copernicus Publications remains neutral with regard to jurisdictional claims in published maps and institutional affiliations.
This open-access publication was funded by Technische Universität Berlin.
This paper was edited by Peter Fiener and reviewed by two anonymous referees.
Abbasi, S., Turner, A., Hoseini, M., and Amiri, H.: Microplastics in the Lut and Kavir Deserts, Iran, Environ. Sci. Technol., 55, 5993–6000, https://doi.org/10.1021/acs.est.1c00615, 2021.
Adhikary, K. B., Pang, S., and Staiger, M. P.: Effects of the accelerated freeze-thaw cycling on physical and mechanical properties of wood flour-recycled thermoplastic composites, Polym. Compos., 31, 185–194, https://doi.org/10.1002/pc.20782, 2009.
Andrady, A. L., Pegram, J. E., and Tropsha, Y.: Changes in carbonyl index and average molecular weight on embrittlement of enhanced-photodegradable polyethylenes, J. Environ. Polym. Degrad., 1, 171–179, https://doi.org/10.1007/bf01458025, 1993.
Bertling, J., Zimmermann, T., and Rödig, L.: Kunststoffe in der Umwelt: Emissionen in landwirtschaftlich genutzte Böden, Fraunhofer Institut, UMSICHT Bericht, https://doi.org/10.24406/umsicht-n-633611, 2021.
Bhardwaj, H., Gupta, R., and Tiwari, A.: Communities of microbial enzymes associated with biodegradation of plastics, J. Polym. Environ., 21, 575–579, https://doi.org/10.1007/s10924-012-0456-z, 2013.
Brandon, A. M., Gao, S. H., Tian, R., Ning, D., Yang, S. S., Zhou, J., Wu, W. M., and Criddle, C. S.: Biodegradation of polyethylene and plastic mixtures in mealworms (larvae of Tenebrio molitor) and effects on the gut microbiome, Environ. Sci. Technol., 52, 6526–6533, https://doi.org/10.1021/acs.est.8b02301, 2018.
Bronick, C. J. and Lal, R.: Soil structure and management: a review, Geoderma, 124, 3–22, https://doi.org/10.1016/j.geoderma.2004.03.005, 2005.
Büks, F. and Kaupenjohann, M.: Global concentrations of microplastics in soils – a review, SOIL, 6, 649–662, https://doi.org/10.5194/soil-6-649-2020, 2020.
Büks, F., van Schaik, N., and Kaupenjohann, M.: What do we know about how the terrestrial multicellular soil fauna reacts to microplastic?, SOIL, 6, 245–267, https://doi.org/10.5194/soil-6-245-2020, 2020a.
Büks, F., van Schaik, N. L., and Kaupenjohann, M.: Mikroplastik aus Klärschlämmen hat das Potential Bodenleben zu schädigen, KW Korrespondenz Wasserwirtschaft, 9, 471–476, https://doi.org/10.3243/kwe2020.09.001, 2020b.
Büks, F., Kayser, G., Zieger, A., Lang, F., and Kaupenjohann, M.: Particles under stress: ultrasonication causes size and recovery rate artifacts with soil-derived POM but not with microplastics, Biogeosciences, 18, 159–167, https://doi.org/10.5194/bg-18-159-2021, 2021.
Chai, B., Li, X., Liu, H., Lu, G., Dang, Z., and Yin, H.: Bacterial communities on soil microplastic at Guiyu, an E-Waste dismantling zone of China, Ecotox. Environ. Safe., 195, 110521, https://doi.org/10.1016/j.ecoenv.2020.110521, 2020.
Cole, M., Lindeque, P., Halsband, C., and Galloway, T. S.: Microplastics as contaminants in the marine environment: a review, Mar. Pollut. Bull., 62, 2588–2597, https://doi.org/10.1016/j.marpolbul.2011.09.025, 2011.
Corradini, F., Meza, P., Eguiluz, R., Casado, F., Huerta-Lwanga, E., and Geissen, V.: Evidence of microplastic accumulation in agricultural soils from sewage sludge disposal, Sci. Total Environ., 671, 411–420, https://doi.org/10.1016/j.scitotenv.2019.03.368, 2019.
Danso, D., Chow, J., and Streit, W. R.: Plastics: environmental and biotechnological perspectives on microbial degradation, Appl. Environ. Microbiol., 85, e01095-19, https://doi.org/10.1128/AEM.01095-19, 2019.
de Souza Machado, A. A., Lau, C. W., Till, J., Kloas, W., Lehmann, A., Becker, R., and Rillig, M. C.: Impacts of microplastics on the soil biophysical environment, Environ. Sci. Technol., 52, 9656–9665, https://doi.org/10.1021/acs.est.8b02212, 2018.
de Souza Machado, A. A., Lau, C. W., Kloas, W., Bergmann, J., Bachelier, J. B., Faltin, E., Becker, R., Görlich, A. S., and Rillig, M. C.: Microplastics can change soil properties and affect plant performance, Environ. Sci. Technol., 53, 6044–6052, https://doi.org/10.1021/acs.est.9b01339, 2019.
Dierkes, G., Lauschke, T., Becher, S., Schumacher, H., Földi, C., and Ternes, T.: Quantification of microplastics in environmental samples via pressurized liquid extraction and pyrolysis-gas chromatography, Anal. Bioanal. Chem., 411, 6959–6968, https://doi.org/10.1007/s00216-019-02066-9, 2019.
Dong, M., Zhang, Q., Xing, X., Chen, W., She, Z., and Luo, Z.: Raman spectra and surface changes of microplastics weathered under natural environments, Sci. Total Environ., 739, 139990, https://doi.org/10.1016/j.scitotenv.2020.139990, 2020.
Dussud, C., Meistertzheim, A. L., Conan, P., Pujo-Pay, M., George, M., Fabre, P., Coudane, J., Higgs, P., Elineau, A., Pedrotti, M. L., Gorsky, G., and Ghiglione, J. F.: Evidence of niche partitioning among bacteria living on plastics, organic particles and surrounding seawaters, Environ. Pollut., 236, 807–816, https://doi.org/10.1016/j.envpol.2017.12.027, 2018.
Edo, C., González-Pleiter, M., Leganés, F., Fernández-Piñas, F., and Rosal, R.: Fate of microplastics in wastewater treatment plants and their environmental dispersion with effluent and sludge, Environ. Pollut., 259, 113837, https://doi.org/10.1016/j.envpol.2019.113837, 2020.
Eerkes-Medrano, D., Thompson, R. C., and Aldridge, D. C.: Microplastics in freshwater systems: a review of the emerging threats, identification of knowledge gaps and prioritisation of research needs, Water Res., 75, 63–82, https://doi.org/10.1016/j.watres.2015.02.012, 2015.
Enders, K., Lenz, R., Beer, S., and Stedmon, C. A.: Extraction of microplastic from biota: recommended acidic digestion destroys common plastic polymers, ICES J. Mar. Sci., 74, 326–331, https://doi.org/10.1093/icesjms/fsw173, 2017.
Flemming, H. C. and Wingender, J.: The biofilm matrix, Nat. Rev. Microbiol., 8, 623–633, https://doi.org/10.1038/nrmicro2415, 2010.
Fotopoulou, K. N. and Karapanagioti, H. K.: Surface properties of beached plastic pellets, Mar. Environ. Res., 81, 70–77, https://doi.org/10.1016/j.marenvres.2012.08.010, 2012.
Fotopoulou, K. N. and Karapanagioti, H. K.: Surface properties of beached plastics, Environ. Sci. Pollut. Res., 22, 11022–11032, https://doi.org/10.1007/s11356-015-4332-y, 2015.
Fuller, S. and Gautam, A.: A procedure for measuring microplastics using pressurized fluid extraction, Environ. Sci. Technol., 50, 5774–5780, https://doi.org/10.1021/acs.est.6b00816, 2016.
Gebhardt, C. and Forster, S.: Size-selective feeding of Arenicola marina promotes long-term burial of microplastic particles in marine sediments, Environ. Pollut., 242, 1777–1786, https://doi.org/10.1016/j.envpol.2018.07.090, 2018.
He, P., Chen, L., Shao, L., Zhang, H., and Lü, F.: Municipal solid waste (MSW) landfill: A source of microplastics? – Evidence of microplastics in landfill leachate, Water Res., 159, 38–45, https://doi.org/10.1016/j.watres.2019.04.060, 2019.
Huang, Y., Liu, Q., Jia, W., Yan, C., and Wang, J.: Agricultural plastic mulching as a source of microplastics in the terrestrial environment, Environ. Pollut., 260, 114096, https://doi.org/10.1016/j.envpol.2020.114096, 2020.
Huerta Lwanga, E., Gertsen, H., Gooren, H., Peters, P., Salánki, T., van der Ploeg, M., Besseling, E., Koelmans, A. A., and Geissen, V.: Microplastics in the terrestrial ecosystem: implications for Lumbricus terrestris (Oligochaeta, Lumbricidae), Environ. Sci. Technol., 50, 2685–2691, https://doi.org/10.1021/acs.est.5b05478, 2016.
Huerta Lwanga, E., Vega, J. M., Quej, V. K., de los Angeles Chi, J., del Cid, L. S., Chi, C., Segura, G. E., Gertsen, H., Salánki, T., van der Ploeg, M., Koelmans, A. A., and Geissen, V.: Field evidence for transfer of plastic debris along a terrestrial food chain, Sci. Rep.-UK, 7, 14071, https://doi.org/10.1038/s41598-017-14588-2, 2017a.
Huerta Lwanga, E., Gertsen, H., Gooren, H., Peters, P., Salánki, T., van der Ploeg, M., Besseling, E., Koelmans, A. A., and Geissen, V.: Incorporation of microplastics from litter into burrows of Lumbricus terrestris, Environ. Pollut., 220, 523–531, https://doi.org/10.1016/j.envpol.2016.09.096, 2017b.
Jiang, P., Zhao, S., Zhu, L., and Li, D.: Microplastic-associated bacterial assemblages in the intertidal zone of the Yangtze Estuary, Sci. Total Environ., 624, 48–54, https://doi.org/10.1016/j.scitotenv.2017.12.105, 2018.
Johansen, M. P., Cresswell, T., Davis, J., Howard, D. L., Howell, N. R., and Prentice, E.: Biofilm-enhanced adsorption of strong and weak cations onto different microplastic sample types: Use of spectroscopy, microscopy and radiotracer methods, Water Res., 158, 392–400, https://doi.org/10.1016/j.watres.2019.04.029, 2019.
Kale, S. K., Deshmukh, A. G., Dudhare, M. S., and Patil, V. B.: Microbial degradation of plastic: a review, J. Biochem. Technol., 6, 952–961, ISSN 0974-2328, 2015.
Katsumi, N., Kusube, T., Nagao, S., and Okochi, H.: Accumulation of microcapsules derived from coated fertilizer in paddy fields, Chemosphere, 267, 129185, https://doi.org/10.1016/j.chemosphere.2020.129185, 2021.
Kockott, D.: Natural and artificial weathering of polymers, Polym. Degrad. Stabil., 25, 181–208, https://doi.org/10.1016/S0141-3910(89)81007-9, 1989.
Lenz, M., Creffield, J. W., Evans, T. A., Kard, B., Vongkaluang, C., Sornnuwat, Y., Lee, C.-Y., Yoshimura, T., and Tsunoda, K.: Resistance of polyamide and polyethylene cable sheathings to termites in Australia, Thailand, USA, Malaysia and Japan: a comparison of four field assessment methods, Int. Biodeter. Biodegr., 66, 53–62, https://doi.org/10.1016/j.ibiod.2011.11.001, 2012.
Liang, Y., Lehmann, A., Ballhausen, M., Muller, L., and Rillig, M. C.: Increasing temperature and microplastic fibers jointly influence soil aggregation by saprobic fungi, Front. Microbiol., 10, 2018, https://doi.org/10.3389/fmicb.2019.02018, 2019.
Lozano, Y. M., Lehnert, T., Linck, L. T., Lehmann, A., and Rillig, M. C.: Microplastic shape, polymer type, and concentration affect soil properties and plant biomass, Front. Plant Sci., 12, 616645, https://doi.org/10.3389/fpls.2021.616645, 2021.
Martin-Closas, L., J., Costa, A., Cirujeda, Aibar, J., Zaragoza, C., A., Pardo, Suso, M. L.,Moreno, M. M., Moreno, C., Lahoz, I., Macua, J. I., and Pelacho, A. M.: Above – soil and in-soil degradation of oxo- and bio-degradable mulches: a qualitative approach, Soil Res. 54, 225–236, https://doi.org/10.1071/SR15133, 2016.
McCormick, A., Hoellein, T. J., Mason, S. A., Schluep, J., and Kelly, J. J.: Microplastic is an abundant and distinct microbial habitat in an urban river, Environ. Sci. Technol., 48, 11863–11871, https://doi.org/10.1021/es503610r, 2014.
Ng, E. L., Lin, S. Y., Dungan, A. M., Colwell, J. M., Ede, S., Lwanga, E. H., Meng, K., Geissen, V., Blackall, L. L., and Chen, D.: Microplastic pollution alters forest soil microbiome, J. Hazard. Mater., 409, 124606, https://doi.org/10.1016/j.jhazmat.2020.124606, 2020.
Oberbeckmann, S. and Labrenz, M.: Marine microbial assemblages on microplastics: diversity, adaptation, and role in degradation, Annu. Rev. Mar. Sci., 12, 209–232, https://doi.org/10.1146/annurev-marine-010419-010633, 2020.
Oberbeckmann, S., Löder, M. G., and Labrenz, M.: Marine microplastic-associated biofilms – a review, Environ. Chem., 12, 551–562, https://doi.org/10.1071/EN15069, 2015.
Pickett, J. E.: Weathering of plastics, in Handbook of Environmental Degradation of Materials, 163–184, William Andrew Publishing, Oxford, ISBN 978-0-323-52472-8, 2018.
Raziyafathima, M., Praseetha, P. K., and Rimal, I. R. S.: Microbial degradation of plastic waste: a review, J. Pharm. ChemBiol. Sci., 4, 231–242, 2016.
Rodríguez-Seijo, A., da Costa, J. P., Rocha-Santos, T., Duarte, A. C., and Pereira, R.: Oxidative stress, energy metabolism and molecular responses of earthworms (Eisenia fetida) exposed to low-density polyethylene microplastics, Environ. Sci. Pollut. R., 25, 33599–33610, https://doi.org/10.1007/s11356-018-3317-z, 2018.
Rodríguez-Seijo, A., Santos, B., da Silva, E. F., Cachada, A., and Pereira, R.: Low-density polyethylene microplastics as a source and carriers of agrochemicals to soil and earthworms, Environ. Chem., 16, 8–17, https://doi.org/10.1071/EN18162, 2019.
Roohi, M., Bano, K., Kuddus, M., R Zaheer, M., Zia, Q., F Khan, M., Gupta, A., and Aliev, G.: Microbial enzymatic degradation of biodegradable plastics, Curr. Pharm. Biotechnol., 18, 429–440, https://doi.org/10.2174/1389201018666170523165742, 2017.
Shimao, M.: Biodegradation of plastics, Curr. Opin. Biotech., 12, 242–247, https://doi.org/10.1016/S0958-1669(00)00206-8, 2001.
Szewc, K., Graca, B., and Dołęga, A.: Atmospheric deposition of microplastics in the coastal zone: Characteristics and relationship with meteorological factors, Sci. Total Environ., 761, 143272, https://doi.org/10.1016/j.scitotenv.2020.143272, 2021.
ter Halle, A., Ladirat, L., Martignac, M., Mingotaud, A. F., Boyron, O., and Perez, E.: To what extent are microplastics from the open ocean weathered?, Environ. Pollut., 227, 167–174, https://doi.org/10.1016/j.envpol.2017.04.051, 2017.
Thiele-Bruhn, S., Bloem, J., de Vries, F. T., Kalbitz, K., and Wagg, C.: Linking soil biodiversity and agricultural soil management, Curr. Opin. Environ. Sustain., 4, 523–528, https://doi.org/10.1016/j.cosust.2012.06.004, 2012.
Thompson R. C., Swan S. H., Moore C. J., and vom Saal F. S.: Our plastic age, Philos. T. R. Soc. B, 364, 1973–1976, https://doi.org/10.1098/rstb.2009.0054, 2009.
Trainic, M., Flores, J. M., Pinkas, I., Pedrotti, M. L., Lombard, F., Bourdin, G., Gorsky, G., Boss, E., Rudich, Y., Lombard, F., Vardi, A., and Koren, I.: Airborne microplastic particles detected in the remote marine atmosphere, Nat. Comm. Earth Environ., 1, 1–9, https://doi.org/10.1038/s43247-020-00061-y, 2020.
Tsunoda, K., Rosenblat, G., and Dohi, K.: Laboratory evaluation of the resistance of plastics to the subterranean termite Coptotermes formosanus (Blattodea: Rhinotermitidae), Int. Biodeter. Biodegr., 64, 232–237, https://doi.org/10.1016/j.ibiod.2009.12.008, 2010.
Wang, J., Coffin, S., Sun, C., Schlenk, D., and Gan, J.: Negligible effects of microplastics on animal fitness and HOC bioaccumulation in earthworm Eisenia fetida in soil, Environ. Pollut., 249, 776–784, https://doi.org/10.1016/j.envpol.2019.03.102, 2019.
Wang, J., Huang, M., Wang, Q., Sun, Y., Zhao, Y., and Huang, Y.: LDPE microplastics significantly alter the temporal turnover of soil microbial communities, Sci. Total Environ., 726, 138682, https://doi.org/10.1016/j.scitotenv.2020.138682, 2020.
Wang, W. H., Wang, Q. W., Xiao, H., and Morrell, J. J.: Effects of moisture and freeze-thaw cycling on the quality of rice-hull-PE composite, Pigment. Resin Technol., 36, 344–349, https://doi.org/10.1108/03699420710831764, 2007.
Wei, R. and Zimmermann, W.: Microbial enzymes for the recycling of recalcitrant petroleum-based plastics: how far are we?, Microb. Biotechnol., 10, 1308–1322, https://doi.org/10.1111/1751-7915.12710, 2017.
Weithmann N., Möller J. N., Löder M. G., Piehl S., Laforsch C., and Freitag R.: Organic fertilizer as a vehicle for the entry of microplastic into the environment, Sci. Adv., 4, eaap8060, https://doi.org/10.1126/sciadv.aap8060, 2018.
Wiedner, K. and Polifka, S.: Effects of microplastic and microglass particles on soil microbial community structure in an arable soil (Chernozem), SOIL, 6, 315–324, https://doi.org/10.5194/soil-6-315-2020, 2020.
Woodall, L. C., Sanchez-Vidal, A., Canals, M., Paterson, G. L., Coppock, R., Sleight, V., Calafat, A., Rogers, A. D., Narayanaswamy, B. E., and Thompson, R. C.: The deep sea is a major sink for microplastic debris, R. Soc. Open Sci., 1, 140317, https://doi.org/10.1098/rsos.140317, 2014.
Wu, C., Zhang, K., and Xiong, X.: Microplastic pollution in inland waters focusing on Asia, in: Freshwater Microplastics, edited by: Wagner, M. and Lambert, S., 85–99, The Handbook of Environmental Chemistry, Vol. 58, Springer, Cham, https://doi.org/10.1007/978-3-319-61615-5_5, 2018.
Yang, X., Lwanga, E. H., Bemani, A., Gertsen, H., Salanki, T., Guo, X., Fu, H., Xue, S., Ritsema, C., and Geissen, V.: Biogenic transport of glyphosate in the presence of LDPE microplastics: A mesocosm experiment, Environ. Pollut., 245, 829–835, https://doi.org/10.1016/j.envpol.2018.11.044, 2019.
Yan, Y., Chen, Z., Zhu, F., Zhu, C., Wang, C., and Gu, C.: Effect of polyvinyl chloride microplastics on bacterial community and nutrient status in two agricultural soils, B. Environ. Contam. Tox., 107, 1–8, https://doi.org/10.1007/s00128-020-02900-2, 2020.
Yi, M., Zhou, S., Zhang, L., and Ding, S.: The effects of three different microplastics on enzyme activities and microbial communities in soil, Water Environ. Res., 93, 24–32, https://doi.org/10.1002/wer.1327, 2020.
Yoon, M. G., Jeon, H. J., and Kim, M. N.: Biodegradation of polyethylene by a soil bacterium and AlkB cloned recombinant cell, J. Bioremed. Biodegrad., 3, 1–8, https://doi.org/10.4172/2155-6199.1000145, 2012.
Yoshida, S., Hiraga, K., Takehana, T., Taniguchi, I., Yamaji, H., Maeda, Y., Toyohara, K., Miyamoto, K., Kimura, Y., and Oda, K.: A bacterium that degrades and assimilates poly (ethylene terephthalate), Science, 351, 1196–1199, https://doi.org/10.1126/science.aad6359, 2016.
Zettler, E. R., Mincer, T. J., and Amaral-Zettler, L. A.: Life in the “plastisphere”: microbial communities on plastic marine debris, Environ. Sci. Technol., 47, 7137–7146, https://doi.org/10.1021/es401288x, 2013.
Zhang, L., Sintim, H. Y., Bary, A. I., Hayes, D. G., Wadsworth, L. C., Anunciado, M. B., and Flury, M.: Interaction of Lumbricus terrestris with macroscopic polyethylene and biodegradable plastic mulch, Sci. Total Environ., 635, 1600–1608, https://doi.org/10.1016/j.scitotenv.2018.04.054, 2018.
Zhang, M., Zhao, Y., Qin, X., Jia, W., Chai, L., Huang, M., and Huang, Y.: Microplastics from mulching film is a distinct habitat for bacteria in farmland soil, Sci. Total Environ., 688, 470–478, https://doi.org/10.1016/j.scitotenv.2019.06.108, 2019.
Zhou, L., Wang, T., Qu, G., Jia, H., and Zhu, L.: Probing the aging processes and mechanisms of microplastic under simulated multiple actions generated by discharge plasma, J. Hazard. Mater., 398, 122956, https://doi.org/10.1016/j.jhazmat.2020.122956, 2020.
Zhou, X., Huang, S., Su, G., Yu, Y., and Chen, L.: Freeze-thaw cycles weathering degrading properties of bamboo flour-polypropylene foamed composites, Trans. Chin. Soc. Agr. Eng., 30, 285–292, https://doi.org/10.3969/j.issn.1002-6819.2014.10.036, 2014.