the Creative Commons Attribution 4.0 License.
the Creative Commons Attribution 4.0 License.
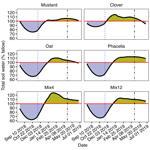
Soil nitrogen and water management by winter-killed catch crops
Norman Gentsch
Diana Heuermann
Jens Boy
Steffen Schierding
Nicolaus von Wirén
Dörte Schweneker
Ulf Feuerstein
Robin Kümmerer
Bernhard Bauer
Georg Guggenberger
Improving N cycling in agroecosystems is one of the key challenges in reducing the environmental footprint of agriculture. Further, uncertainty in precipitation makes crop water management relevant in regions where it has not been necessary thus far. Here, we focus on the potential of winter-killed catch crops (CCs) to reduce N leaching losses from N mineralization over the winter and from soil water management. We compared four single CCs (white mustard, phacelia, Egyptian clover and bristle oat) and two CC mixtures with 4 and 12 plant species (Mix4 and Mix12) with a fallow treatment. High-resolution soil mineral N (Nmin) monitoring in combination with the modelling of spatiotemporal dynamics served to assess N cycling under winter-killed CCs, while soil water was continuously monitored in the rooting zone. Catch crops depleted the residual Nmin pools by between 40 % and 72 % compared to the fallow. The amount of residual N uptake was lowest for clover and not significantly different among the other CCs. Catch crops that produce high N litter materials, such as clover and mustard leaves, showed an early N mineralization flush immediately after their termination and the highest leaching losses from litter mineralization over the winter. Except for clover, all CCs showed Nmin values between 18 % and 92 % higher on the sowing date of the following maize crop. However, only Mix12 was statistically significant. Catch crops depleted the soil water storage in the rooting zone during their growth in autumn and early winter, but preserved water later on when their residues covered the ground. The shallow incorporation of CC residues increased water storage capacity during the cropping season of the main crop even under reduced soil water availability. Hence, catch cropping is not just a simple plant cover for the winter but improves the growth conditions for the following crop with decreased N losses. Mixtures have been shown to compensate for the weaknesses of individual CC species in terms of nutrient capture, mineralization and transfer to the following main crop as well as for soil water management. Detailed knowledge about plant performance during growth and litter mineralization patterns is necessary to make optimal use of their potential.
- Article
(2624 KB) - Full-text XML
-
Supplement
(1839 KB) - BibTeX
- EndNote
Catch crops (CC, also named cover crops) are well-known tools for biological N management in arable soils of temperate climates (Thorup-Kristensen et al., 2003). Catch crops efficiently deplete residual soil mineral N (Nmin) pools derived from fertilizers and crop residue mineralization and thereby lower the risk of N leaching. Between 10 % and 35 % of the immobilized N is thereby stored in roots and rhizodeposits (Heuermann et al., 2022; Kanders et al., 2017). The aboveground biomass can be either harvested as fodder or act as green manure for fertilizing the following cash crop and improving soil quality. Catch crops replace fallow periods and protect soil against water and wind erosion. In practical applications, CCs are often fertilized with farmyard manure to increase their storage capacity before winter and maximize CC biomass production. Current legal regulations in Germany allow for fertilization of CCs up to 30 kg ammonium – N, 60 kg ha−1 total N or 120 kg ha−1 organic N as solid manure. Cover crops with >75 % legumes are restricted to fertilization with organic N from farm manure only. This generates high N loads in autumn, which have to be taken up by CCs, in addition to the N mineralized in soil.
The potential of a certain CC for soil Nmin depletion depends on the volume of their rooting zone as well as their aboveground biomass production. Dicot species, for example, were shown to deplete subsoil horizons (>0.5 m) more efficiently than monocot species (Thorup-Kristensen, 2001). Legumes are not as effective as other plants in N depletion due to their symbioses with rhizobia. This enables legumes to add substantial amounts of N from atmospheric N fixation (Thorup-Kristensen et al., 2003). Farmer decisions for a certain CC are mainly driven by fitting the crop rotation of main crops rather than fitting local weather conditions or the nutrient status of soils. Diverse CC mixtures have the advantage of combining several species with different rooting depths, nutrient acquisition strategies and growth optima, thus providing multifunctionality. Catch crop mixtures were shown to overyield the root biomass of pure stands and show higher potential for efficient soil exploration by roots (Heuermann et al., 2019). Increasing CC diversity also stimulates soil microbial diversity and biomass (Gentsch et al., 2020; Vukicevich et al., 2016), which represent a considerable reservoir for N during winter (Paul, 2007) and may contribute to N immobilization.
The advantage of winter-killed CCs over winter-hardy is the saving of energy for CC termination and the reduction of soil tillage (Gollner et al., 2020). Even no-till practices without herbicide applications are possible if CCs are reliably terminated by frost (Romdhane et al., 2019). The challenge of winter-killed CCs, however, is the risk of losing early N by leaching and reducing the N carry-over to the succeeding crops if the plant residues are mineralized too fast. The C:N ratio of the CC biomass is an important indicator for litter quality and its turnover during litter mineralization. In a previous study, the potential for N leaching increased with decreasing C:N ratio, while the potential to support nutrient demands of the following crop increased (Finney et al., 2016). The study of Finney et al. (2016) also suggested that a C:N threshold exists for the aboveground biomass. At approximately C:N 20, the N retention was still at a maximum, and the crop yield service was high. Customized CC mixtures with desired litter quality might therefore be favourable for soil N management. Research has made progress recently, demonstrating the multiple ecosystem services of CC mixtures in terms of residual N depletion and effective green manure services (Couëdel et al., 2018; Vogeler et al., 2019; Selzer and Schubert, 2021; White et al., 2017). Despite this, much uncertainty exists about the timing of N mineralization from winter-killed CC and the potential of CC mixtures to minimize N leaching in comparison to single species.
Soil water management is becoming a new challenge with increasing aridity due to climate extremes and drought spells. Catch crops require water for their establishment and deplete the soil water pools while they grow. Competition for water with the main crop could result in yield reduction in semiarid and arid environments (Unger and Vigil, 1998). In contrast, maintaining CC residues on the soil surface reduces evapotranspiration and increases the infiltration capacity of the soils. Therefore, the net effect of a CC on the soil water balance depends on the standing time, precipitation timing and capacity of the CC to reduce water losses (Bodner et al., 2007; Unger and Vigil, 1998).
The objectives of this study were to investigate the N uptake efficiency in monoculture vs. mixed CC stands and explore the timing of N release into the soil after CC termination. Further, this study sought to evaluate the role of different CC treatments on crop water availability in comparison to fallow conditions. We followed the soil Nmin pool over 313 d from CC cultivation until the shoot elongation of the following maize crop. High-resolution soil sampling combined with modelling generated detailed insights into the winter mineralization pattern of CCs. We hypothesized that (I) the positive effects of CC on N uptake during growth and on N mineralization after death and its transfer to the following crop could be optimized in CC mixtures and that (II) CCs do not negatively affect the soil water balance of the main crop under reduced soil water availability.
2.1 Field site
The study was conducted at a long-term field experiment in Asendorf, northern Germany (49 m above sea level, 52∘45′48.4′′ N 9∘01′24.3′′ E). The soil developed from shallow loess cover over glaciofluvial sands and is classified as Stagnic Cambisol (IUSS Working Group WRB, 2014). The soil texture is silt loamy with 7 % clay, 73 % silt and 20 % sand. The climate is temperate oceanic with a mean average annual precipitation (MAP) of 751 mm and a mean annual temperature (MAT) of 9.3 ∘C (long-term mean, 1981–2010). The first frost usually appears at the end of November, and the long-term annual average of frost days is 54. Soil monitoring spanned a 313 d period from 15 August 2018 to 24 June 2019. Temperature and precipitation during that period are displayed in Fig. S1. The year 2018 was exceptionally dry, with 535 mm total precipitation, while 2019 had 780 mm.
Seven treatments in three replicates were investigated in a randomized block design with four pure CCs, two mixtures and a fallow with no CC. Total plot size was 9 m by 9 m. The pure stands were white mustard (Sinapis alba), lacy phacelia (Phacelia tanacetifolia), bristle oat (Avena strigosa) and Egyptian clover (Trifolium alexandrinum). A mixture of the four species was applied as Mix4 (percentage of the individual species in Table S1). For the highest species diversity (Mix12), we used a commercial 12 species mixture (TerraLife© Maize-Pro TR Greening, Deutsche Saatveredelung, Lippstadt, Germany) composed of field pea (Pisum sativum), crimson clover (Trifolium incarnatum), alsike clover (Trifolium hybridum), persian clover (Trifolium resupinatum), Hungarian vetch (Vicia pannonica) and non-legume species, including sorghum (Sorghum sudanense), common flax (Linum usitatissimum), lacy phacelia, deep-till radish (Raphanus sativus), ramtil (Guizotia abyssinica), sunflower (Helianthus annuus) and camelina (Camelina sativa). Seeding rations and seeding densities are presented in Table S1. The CCs followed winter wheat (Triticum aestivum), and maize (Zea mays) followed CCs. Catch crops were sown on 10 August 2018 and fertilized with 47 kg N ha−1 (UAN 28), which is 81 % of the legal N fertilization rate. The CC seedbed was prepared by a harrow/disc harrow combination and seeding was done by a seed drill to ensure that all seeds were covered by soil. Each CC plots, 9 m by 9 m wide, consisted of six 1.5 m wide subplots. Three of the subplots were within plot replicates for agronomic parameters (biomass, yield). The other three subplots were reserved for soil and root sampling only. Due to the different frost limits of plants, we wanted to have equal conditions for all cover crop treatments. Therefore, CC was terminated in one of the subplots immediately before the first frost event (9 November 2018). This ensured equal starting conditions for plant material decomposition. Catch crops were cut just above the soil surface and left as whole plants on the ground. In spring, CC residues were mulched and incorporated into the soil (maximum working depth 15 cm) 2 weeks before maize seeding with a harrow/disc harrow combination. Maize was sown on 24 April 2019 and harvested on 12 September 2019 with fertilizer applications according to Table S2. The timeline of all actions during the study is presented in Fig. S2.
2.2 Determination of catch crop biomass and silage maize yield
Catch crop plant biomass was determined at the end of the vegetation period (9 November 2018) in three replicates per plot in 1 m wide squares. In mixtures, plants were sorted according to the species and weight separately. Dry matter yield (DMY) was determined gravimetrically after drying of plant material at 60 ∘C until constant weight. For root biomass quantification, three soil cores were taken per plot, having a diameter of 6 cm and a length of 100 cm. Soil cores were cut into three pieces, representing 0–30, 30–60 and 60–100 cm soil depth. Buckets with a middle-placed high cylindric pipe, opened at the top and equipped with a 0.4 mm mesh-sized web at the bottom, and a continuous water flow around that pipe, were used to elutriate the samples. During this procedure, lightweight roots were separated from heavier soil particles and could be captured in the web at the bottom of the cylindric pipe while water ran over the top end of the pipe. Additional lightweight particles like seeds or straw were sorted manually from 10 randomly taken samples per soil depth. In these samples, roots were completely separated from remaining material. After drying root material at 60 ∘C to constant weight, dry mass could be determined. A mean value of 67.3 % of non-root material dry weight (mainly straw from pre-grown wheat) was applied to all samples of 0–30 cm depth, while in the deeper soil layers, shares of non-root material were neglectable. Total organic carbon (OC) and N (TN) concentrations in plant materials were measured with dry combustion in an elemental analyser (EuroEA3000, Hekatech, Wegberg, Germany).
Silage maize yield was determined from three individual subplots (1.5 m × 9 m) per replicate. Fresh matter yield was determined by a plot harvester in the field. An aliquot sample of each subplot was dried at 105 ∘C in the laboratory to calculate DMY in t ha−1.
2.3 Soil mineral nitrogen and volumetric water content
Soil samples were taken with a manual soil corer from four soil depths (0–10, 20–30, 50–60 and 70–80 cm). The sampling campaign comprised nine sampling points in the period from 15 August 2018 to 24 July 2019 (Fig. S2, all dates provided in Table 2). Soil samples were immediately stored in a cooler and transported to the laboratory for fresh sample extraction. Approximately 10 g of soil material was extracted with 40 mL of a 0.0125 M CaCl2 solution after being shaken overhead for 60 min. Soil Nmin was determined as the sum of the measured NH and NO concentrations by an auto analyser (SAN, Skalar Analytical B.V., Breda, The Netherlands). A sample aliquot was dried at 105 ∘C to determine the gravimetric soil water content (% dry mass) and relate Nmin values to the dry weight base. Bulk density (BD) was determined gravimetrically after drying undisturbed soil cores at 105 ∘C. Soil cores were taken by a stainless core cutter (100 cm3) only once per plot, and we assume that the data did not change significantly during the observation period. Field capacity was estimated from pedotransfer functions using soil texture and BD according to Ad-hoc-AG Boden (2005). The volumetric soil water content (vol %) was calculated based on the soil mass taken from gravimetric values. Total water storage was calculated as the sum of all soil depth increments and referred to the total soil volume to 80 cm depth. Nmin stocks were calculated for individual soil depth increments (for interpolation, see Sect. 2.4) with Eq. (1) and summarized to 80 cm soil depth:
2.4 Data logger
As a complement to our offline soil moisture measurements, we installed data loggers for continuous monitoring. We used CS650 multiparameter sensors connected to a CR300 data logger (Campbell Scientific, Inc., Logan, USA). The logger delivered hourly data on soil volumetric water content, temperature and bulk electrical conductivity for the upper 0 to 30 cm soil depth. In the following, we discuss the data on volumetric water content only. The loggers collected continuous data for 1 year (from 27 September 2018 to 24 September 2019) with short removals for soil preparation. Unfortunately, some loggers were seriously damaged by wild animals and we lost six replicates for the statistical evaluations.
2.5 Statistics and depth–time visualization
In total, 840 Nmin samples at nine time points were incorporated in the spatiotemporal visualization of the values (time points are shown in Fig. S2 and the primary data files). In the first steps to produce heat maps (Figs. 2 to 4) with R version 4.0.2, field replicates (N=3) were summarized per treatment for easier data handling (R Core Team, 2020). Next, horizontal interpolation was performed on a daily basis by a local polynomial regression model (span = 0.2). The same model was applied for depth interpolation on 50 equally distributed data points until 80 cm soil depth. With this procedure, we generated 15 700 data points per treatment and plotted them with a geom_raster in ggplot2 version 3.3.2 (Wickham, 2016). Differences in plant biomass and OC and TN concentrations were analysed by pairwise t tests (pairwise test package). Values for total plant OC and TN concentrations and C:N ratios were calculated as weighted means according to the equivalent mass ratio of roots and shoots. We used bootstrapping prior to pairwise t tests to compare Nmin stocks between treatments at specific sampling dates. All metadata and R-scripts can be publicly accessed from the DOI provided below (Sect. Code and data availability). All statistical statements, plots and calculations of data variability can be reproduced from the scripts.
3.1 Biomass and plant nitrogen accumulation
Total shoot biomass was highest for phacelia, followed by oat, the two mixtures and mustard (Table 1). Clover showed less than half of the shoot biomass of the other CC treatments. Root biomass summarized to 100 cm soil depth was highest for oat and decreased in the order Mix12, Mix4, phacelia, mustard and clover. Total plant biomass followed a similar pattern. The slow biomass development of clover encouraged voluntary winter wheat and several regional weeds to germinate. These were weeded manually once. The rest of the CC treatments suppressed weeds very well. Growth of weeds and volunteer winter wheat was documented and their contribution to total biomass was negligible. The highest average biomass N was found in Mix12, but differences with other treatments were not significant due to large data variability (Table 1). Despite the low biomass production, clover produced remarkably high biomass N, in the same range as mustard. The biomass C:N ratios clearly distinguished the different CC treatments, and the total C:N ratio increased in the order clover, Mix12, oat, mustard, phacelia and Mix4. C:N ratios were significantly higher in roots than in shoots, and the lowest root C:N ratio was found in Mix12. In total, the proportion of legumes in the shoot biomass of mixtures ranged from 0.5 % to 1 % in Mix4 (1.03 ± 0.16 % on average) and 7 % to 22 % in Mix12 (16.2 ± 2.6 % on average), and thus explained the lower C:N ratio of Mix12 as compared to Mix4.
3.2 Soil water budget
Calculations of the total soil water content showed that in all depth increments, the field capacity of the soil was never exceeded in the soil profile (Fig. S3). A period of higher rainfall starting at the end of January (Fig. S1) increased the soil water content in the upper 10 cm from 66 % to 95 % of field capacity.
Total soil water storage was calculated from the summarized volumetric water content to 80 cm soil depth, with the mean values of fallow as a reference (100 %) in Fig. 1. The periods of CC growth clearly deplete the soil water storage until its maximum growth. At the beginning of November 2019, relative moisture levels under CC reached their minimum, between 17 % and 33 % below the water storage of fallow, which was significant for all CCs except clover (Table S3). The soil water storage started to recover already before CC termination, and increased towards the fallow level until mid-December 2018 (Fig. 1). Thereafter, all CC treatments showed significantly higher soil water storage than the fallow treatments (Table S3). After soil preparation and maize sowing, all CCs still showed higher soil water storage than the fallow CCs (mustard +4 %, clover + 5 %, oat +6 %, phacelia +12 %, Mix4 +14 %, Mix12 +9 %), but only phacelia and Mix12 were statistically significant (p<0.05).
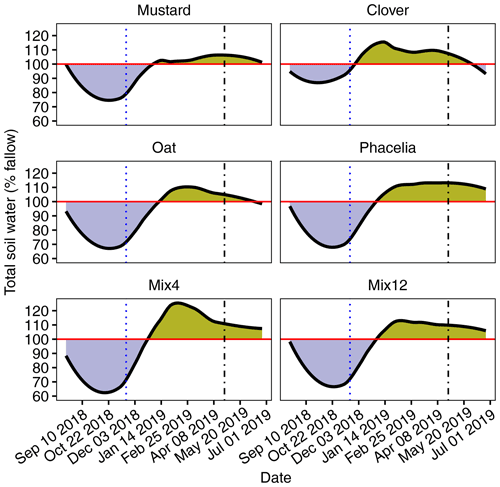
Figure 1Volumetric soil water content down to 80 cm soil depth, with fallow as a 100 % reference (red horizontal line). Blue areas mark soil water content below the fallow level and yellow/brown values above. Continuous black lines were achieved by logistic regression models from three replicates, and the differences between the treatments at the individual sampling dates are provided in Table S3. The blue dotted line marks the termination of CC, and the vertical dashed line marks the seeding date of the main crop maize.
The loggers delivered 1 year of continuous data of the volumetric water content to 30 cm soil depth and supported the results from our soil sample measurements. Due to the loss of replications, we were not able to apply proper statistical comparisons of treatments. Nevertheless, the data fit the measurements of the volumetric water content in the laboratory and allowed us to extend the interpretation beyond maize harvest. In agreement with the laboratory measurements, the logger data showed depletion of soil water by CC of all treatments (Figs. S4 and S5). Soil water was restored until soil preparation for maize seeding, and exceeded the fallow levels except for mustard (Fig. S4; mustard ±0 %, clover +13 %, oat +9 %, phacelia +12 %, Mix4 +13 %, Mix12 +14 %). During maize growth, all CC treatments except for Mix4, where we lost all replicates, exceeded the fallow level. This was the case particularly at the start of August 2019: when maize reached the stage of corn filling, the CC treatments exceeded the fallow by up to 200 % (Fig. S5).
3.3 Soil N depletion and temporal Nmin fluctuations
In Figs. 2 to 4, the dynamics of Nmin concentrations can be followed in temporal heat maps as model results from individual sampling points. After the exceptionally dry summer of 2018, the onset of precipitation in combination with mild weather generated high autumn N mineralization. This mineralization pulse became particularly relevant in the fallow treatments up to a soil depth of approximately 40 cm between the start of September and mid-November (Fig. 2a). Below this depth, the soil was still Nmin depleted from the previous wheat crop uptake. After the first frost periods (Fig. S1), the mineralization pulse decreased, and precipitation events resulted in descending soil water migration accompanied by diffuse Nmin leaching toward the subsoil. In the first half of December, the subsoil Nmin concentrations at 70 to 80 cm under fallow conditions increased significantly (p=0.0034), by threefold (from 1.1 ± 0.2 to 3.4 ± 0.3 g kg−1), and reached their maxima in January 2019 (8.7 ± 0.7 g kg−1). Thereafter, the Nmin concentrations at 70 cm to 80 cm decreased again until the end of the observation (4.5 ± 0.5 g kg−1). The fluctuations in N mineralization gains and N leaching losses were very prominent at 20 to 30 cm depth under fallow conditions. Here, the Nmin values dropped from a maximum of 19.3 ± 1.6 g kg−1 in November to 1.9 ± 0.6 g kg−1 at the last sampling before maize seeding and fertilization.
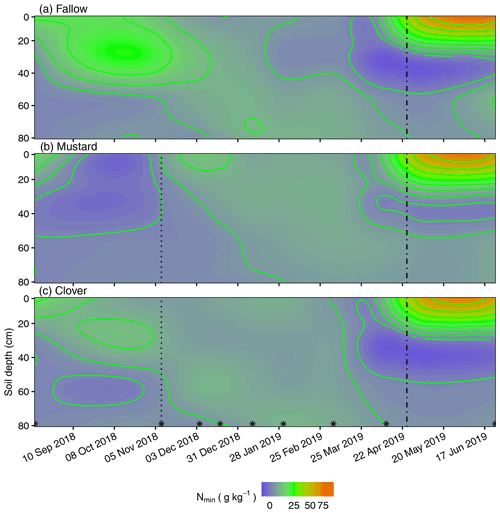
Figure 2Spatiotemporal resolution of Nmin concentrations in soil spanning a 313 d period under (a) fallow conditions, (b) mustard and (c) clover. The dotted lines indicate CC termination, dashed lines mark the seeding of maize following CCs or fallow and asterisks indicate sampling dates.
Clover was the only CC treatment that showed a similar pattern as bare fallow (Fig. 2a and c). The variability between the maxima and minima, however, was not as strong as in the fallow. Despite this, clover reduced the average Nmin stocks in November 2018 by 40 % compared to the fallow stocks, but the variance of the data was too large to be significantly different (Fig. 5, Table 2). All other CC treatments significantly reduced the Nmin pools in autumn by 66 % to 72 % compared to the fallow treatment (Fig. 5, Table 2). Mustard reduced the autumn mineralization pulse by 80.3 ± 6.9 kg ha but unlike phacelia and oat, for example, we observed a very early increase of higher Nmin loads from litter mineralization. Under mustard, the Nmin concentrations in the upper 20 cm increased strongly from 4.0 ± 0.4 g kg−1 at the termination date to 13.8 ± 2.4 g kg−1 in early December. This represents an increase in the Nmin stocks by 17.9 kg ha−1.
Table 2Pairwise comparison of soil mineral N stocks (Nmin in kg ha−1) from Fig. 5 at the individual sampling dates. R codes and data are provided in the Supplement. Small letters denote the contribution to significantly different groups.
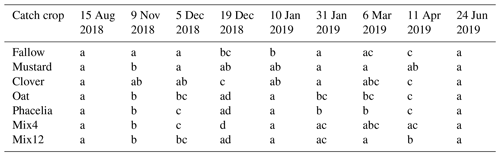
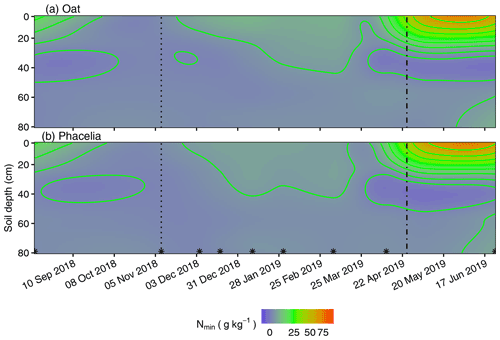
Figure 3Spatiotemporal resolution of Nmin concentrations in soil spanning a 313 d period under (a) oat and (b) phacelia. The dotted lines indicate CC termination, dashed lines mark the seeding of maize following CCs or fallow and asterisks indicate sampling dates.
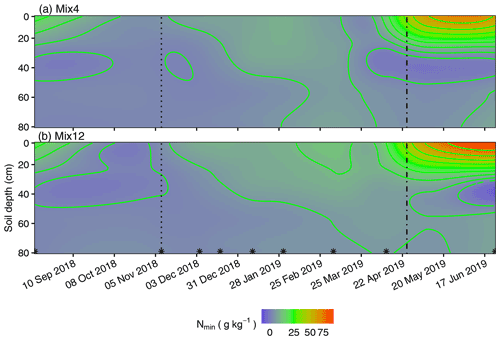
Figure 4Spatiotemporal resolution of Nmin concentrations in soil spanning a 313 d period under (a) Mix4 and (b) Mix12. The dotted lines indicate CC termination, dashed lines mark the seeding of maize following CCs or fallow and asterisks indicate sampling dates.
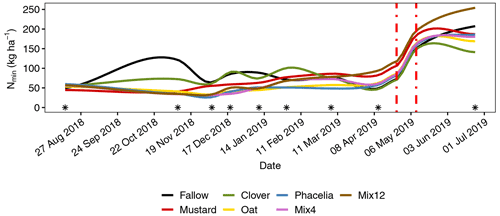
Figure 5Modelling of soil mineral N stocks (Nmin in kg ha−1) during the observation period under different CC treatments. Each line represents the mean of three replicates. Soil sampling events as baseline for local polynomial regression models are marked with asterisks. Differences between treatments at individual sampling events are provided in Table 2. The vertical dotted red lines mark the seeding with the first N fertilization and the second N fertilization (Table S2). The smoothing function of the model caused a gradual increase between sampling at 11 April 2019 and first maize fertilization but in reality it should increase more abruptly with the fertilization events.
The quantification of the N leaching from downward migration with the hydraulic gradient was not straightforward. However, we could estimate the leaching from fallow and clover by simple calculation of the difference between Nmin stock maxima and minima during the winter. We calculated a total N leaching to >80 cm soil depth of 103 kg ha−1 for the fallow and 81 kg ha−1 for the clover as the sum over three periods (exact calculation steps provided in the R files). Considering 47 kg N added by fertilization to the fallow, we end up with 54 kg ha−1 for fallow and 34 kg ha−1 for clover derived from mineralization of organic N pools throughout the winter. For the other CC treatments, we recorded a constant increase in total Nmin stocks in the soil profile (0–80 cm) over time. Therefore, it was not possible to simply summarize gains and losses. Instead, the gains and losses in the 70 to 80 cm soil depth were used as indicators of Nmin discharge. The calculated Nmin losses were 7.4 kg ha−1 for phacelia, 6.0 kg ha−1 for oat, 18.2 kg ha−1 for Mix4 and 10.0 kg ha−1 for Mix12. For mustard, we assume a constant input and output at 70 to 80 cm soil depth. The discharge rate was calculated from a linear regression to 0.11 kg ha−1 d−1, and the summarized Nmin loss over 156 d was 18.4 kg ha−1.
3.4 Maize yield
The DMY of silage maize was significantly higher than the fallow for all CC treatments except phacelia (Fig. 6). The Mix12 showed the highest average DMY, which was significantly higher than clover, oat and phacelia, but not statistic significant compared to mustard and Mix4.
4.1 Soil water budget
Catch crops deplete the available soil water during their growth (Fig. 1, Fig. S5). At the same time, the convective transport rate of pore water throughout the soil column is lower as under bare fallow conditions. The lower seepage water rate causes a reduction of N leaching losses in humid years. The extreme drought throughout 2018 did not result in seepage during the winter of 2018/2019, as winter precipitation was not enough to restore the field capacity of the soils (Fig. S3). Thus, the convective transport of water by the mass flow through the soil column was absent. Despite the missing seepage, our data indicate Nmin movements throughout the soil profile, with the hydraulic gradient and diffusive N transport via concentration gradients as the principle drivers (Cameron et al., 2013). We can exclude capillary rise from the subsoil, as the groundwater table was >3 m below the surface. The much drier subsoils than surface soils in winter 2018/2019 (Fig. S3) caused a strong hydraulic gradient toward the subsoil.
Our data showed that in mid-December 2018 (4 weeks after CC termination), the soil water budget of CC was equal to the fallow budget and exceeded their level thereafter (Fig. 1). We assume the combination of several factors as drivers for constantly higher soil water content by all CC variants. (I) The exposed bare soil of the fallow is prone to splash effects by precipitation. Soil pores can be clogged, and surface crust formation restricts water infiltration into the soil, causing surface runoff. Catch crop residues increase soil roughness, protect the soil surface from splash effects and minimize surface runoff (Unger and Vigil, 1998). (II) Catch crops produce biopores by decaying roots. Preferential flow paths follow these root channels (Kautz, 2015) and increase water infiltration. (III) The litter of frost-killed CCs acts as mulch and reduces surface dry out by wind and interception losses (Unger and Vigil, 1998; Bodner et al., 2007, 2015).
However, not all CC species are equally suitable for water conservation. Species vary in their transpiration rates, water use efficiency, rooting depth and canopy coverage. Bodner et al. (2007) demonstrated that phacelia and vetch had a substantially lower evapotranspiration than rye and mustard. Within their study, mustard showed by far the largest transpiration rates. Our data indicate that clover consumed only half of the water during its growth period as compared to the other CCs. This was due to the lower biomass and rooting depth (Table 1; Heuermann et al., 2019). The soil of the upper 0 to 30 cm following clover constantly showed a 10 % to 33 % higher water content than fallow during the whole maize cropping period (median 19 %, Fig. S5). Mix12 and Phacelia showed similar results until mid-July. When maize plants entered the generative stage, all CC treatments except clover showed up to 200 % higher soil water content than the fallow treatments (Fig. S5). Similar results of higher crop water availability for the following crop were reported by Kaye and Quemada (2017) and were explained by a stronger mulch effect and higher soil organic matter (OM) content. Interestingly, in our experiments, clover showed the least benefit for crop water availability during the generative maize stage. The only explanation we came up with thus far could be the lower root and shoot biomass of clover that produced less particulate OM and biopores compared to the other CC treatments. This could result in a lower mulch effect and slower infiltration in the upper 30 cm of the clover treatments. The reasons that caused the differences between clover and other CC treatments remain quite speculative. Infiltration experiments, measurements of evaporation rates, aggregate fractionation or evaluation of biopores could help future studies explore the potential of CCs for soil water management.
Overall, CCs deplete soil water while they are growing and preserve water when they are killed, and their residues cover the ground. Catch crops can thus be used as tools for soil water management. In drought years, early frost killing or termination by rolling, crimping or mulching will stop transpirational water loss from the soil. Further, the residual mulch will decrease evaporation and increase infiltration. In moist or normal years, winter-hardy CCs and late termination can be used to dry the soil to a level that is optimal for seed bed preparation (Kaye and Quemada, 2017). Phacelia and Mix12 showed the most consistent results concerning the temporal course of the water content. We thus conclude that CC mixtures with high diversity combine several positive effects for soil water management for the following crop.
4.2 Soil N management with winter-killed catch crops
This study clearly visualizes Nmin migration in soil following drought. Although there was only slow, unsaturated soil water migration, Nmin leaching toward the subsoil was recorded as a result of the hydraulic gradient. Our calculations indicated that the fallow lost at least 54 kg ha−1 Nmin derived from residual fertilizers of the preceding crop and the autumn mineralization pulse of crop residues and soil organic matter. In wetter years with high seepage rates, Nmin leaching of up to 80 kg ha−1 from the fallow at the same sites has been recorded (data not shown).
Clover reduced the soil Nmin stocks in autumn by 40 %. This was only half of the reduction of the other CC treatments and well in the ranges of similar studies (Couëdel et al., 2018). The low biomass and thus N uptake and the clover's lower dependence on N fertilizers due to N fixation are the main reasons for the weak performance in residual N depletion. Therefore, current legislation does not recommend the fertilization of legumes. Winter-hardy varieties such as winter vetch (Vicia villosa) or white clover (Trifolium repens) and/or inclusion in mixtures of various legume species can extend the N mineralization pulse to the main crop season. Legumes perform several ecosystem services other than residual N depletion. Nitrogen facilitation, the transfer of N from legumes to companion plants in mixtures (Paynel and Cliquet, 2003), is crucial to maximizing CC biomass without fertilizer application and reducing interspecific competition (Duchene et al., 2017). Legumes in cropping systems reduce the total amount of energy that is required for crop production by 12 % to 34 % (Jensen et al., 2012). Furthermore, the high litter quality of legumes is an integral part of supporting complex soil microbial abundance and diversity and improves microbial C use efficiency and soil OM build-up (Jensen et al., 2012).
For mustard, we observed a very early flush of Nmin from litter mineralization immediately after termination (Fig. 2b). In January 2019, we measured similar Nmin concentrations in 80 cm soil depth compared to fallow or clover. Although the C:N ratios of roots (30.0) and total shoots (19.3) did not differ significantly from phacelia and oat (Table 1), our previous studies showed that the C:N ratio of mustard leaves alone is between 8 and 11 (mean 10.5; see Table S4). The high N leaf litter material is most likely the main cause for the early mineralization flush and contributes to the higher Nmin leaching potential from mustard residues over the winter. The fibre-rich mustard stalks, however, showed C:N ratios between 23 and 41 (mean 34) and were left over on the soil surface after the winter (Fig. S5). The incorporation of low N CC materials is likely to cause microbial N immobilization with negative impacts on the main crop N supply and crop yield services at a C:N ratio of 25 (Finney et al., 2016). However, during the initial maize growth, we did not detect a pattern of N immobilization for mustard and even measured the highest N loads together with Mix12.
The lowest Nmin losses were calculated for the pure stands of phacelia and oat. Oat roots had wider C:N ratios (Tables 1 and S3), and the shoot litter remained as a thick litter mat on the soil surface (Fig. S6). This most likely extended oat litter mineralization into the early spring. Phacelia showed a similar N mineralization pattern as oat (Fig. 3), but the C:N ratios were quite narrow, particularly in phacelia roots (Tables 1 and S4). It is still unclear why the decomposition of phacelia litter is similarly retarded as for oat, as litter quality is unlikely to explain this observation.
Nitrogen losses during CC rotations can also occur as greenhouse gas losses in the forms of NH3, N2, NO or N2O. The latter has received much attention recently, because periods of high precipitation can turn CC rotations from a N2O sink to a source. In particular, legume CCs (Basche et al., 2014) and Brassica CCs (Bodner et al., 2017; Li et al., 2015) have been criticized for its high N2O emission potential from decaying biomass. Similar to Nmin leaching, the N2O emission potential depends on species/mixture composition, management practices of cover crop residues and the time point of termination. I was concluded that CC services do not appear with the cost of enhanced greenhouse gas emissions if multispecies CC are applied and pure stands of legume or Brassica species can be avoided (Bodner et al., 2017; Abdalla et al., 2019; Basche et al., 2014).
The mixing of different CC species can compensate for the weaknesses of individual single CCs with respect to residual N depletion, extension of winter mineralization, and N transfer towards the following maize crop. We demonstrated that CC mixtures with up to 22 % legumes in shoot biomass took up similar amounts of Nmin during the growing period from soil compared to pure non-legume CCs. Similar effects of mixed CCs were previously reported by Couëdel et al. (2018). At the same time, the N fixation service by legumes improved litter quality in Mix12 with low C:N ratios in shoot and root litter. Lower C:N ratios of litter materials have been linked to higher carbon-use efficiency from the microbial community (Manzoni et al., 2012), which triggers OM build-up in soil (Nicolardot et al., 2001; Liu et al., 2018). Mix4 did not perform well in terms of litter quality adjustment. Egyptian clover contributed only around 1 % to the total shoot biomass and was not competitive enough against mustard and phacelia. The high N availability might reduce legume competition in Mix4. Care must be taken to ensure a suitable balance between companion plants in low species mixtures and fertilization to achieve the desired functions. Nevertheless, Mix4 did not show a very early winter mineralization pulse compared to mustard and clover alone, and it extended the recovery of Nmin pools until January 2019.
The Nmin supply to the following crop was best for Mix12 and 92 % higher than the fallow level at the time of maize seeding, which was also confirmed in previous studies on CC mixtures. Farneselli et al. (2018), for example, found that vetch best supported the nutrient demand of processing tomatoes, but also resulted in high N leaching from the soil. In contrast, vetch mixed with barley ensured adequate tomato nutrition while minimizing leaching losses. Rinnofner et al. (2008) found that mixtures of legumes and non-legumes performed best in terms of a balanced biomass N yield, biological N fixation and soil N depletion compared to pure legumes or non-legumes. Crucifer–legume mixtures mineralized more N and had a higher green manure effect on the following crop than pure crucifer CCs (Couëdel et al., 2018). However, CC mixtures did not necessarily perform better than their best-performing constituent monoculture. Florence and McGuire (2020) analysed seven metrics of CC performance in a review of 27 studies. They found that the best monocultures and mixtures perform comparably if the metrics are compared independently to each other. The true potential of CC mixtures is, however, their multifunctionality. In our study, each of the four monocultures showed specific weaknesses in terms of biomass production, residual N uptake, N leaching, N mineralization and delivery toward the following maize crop and soil water conservation. In all of these metrics, the mixtures, particularly the high diversity Mix12, were always comparable to the best-performing monoculture and were found to be the best compromise in terms of multifunctionality. This is supported by the highest silage maize yield level of Mix12 followed by Mix4. Therefore, we conclude that mixtures can compensate for the individual weaknesses of monocultures and provide functional redundancy. Overall, high-diversity CC mixtures have strong agronomic potential for optimizing N cycling and crop water management in arable soils.
This study demonstrates that catch cropping during years with reduced soil water availability did not result in water shortages for the following crop. Catch crops deplete soil water while they are growing but reduce evaporation and preserve water compared to bare fallow after their dieback. The shallow incorporation of CC residues into the soil increased the infiltration and water storage capacity under the following main crop. The CC biomass and the percentage of soil cover from their residues determine the magnitude of the benefits for the following crop. Phacelia and Mix12 showed the most consistent combined results from data loggers and soil moisture measurements in the laboratory. High diversity mixtures most likely combine several positive aspects of soil water management that result from an optimized rooting depth and volume, plant transpiration, soil coverage by mulch and evaporation and OM input.
All CC treatments depleted the soil Nmin pools while they grew. The magnitude of depletion depended on the standing biomass and the rooting volume. Our results demonstrated that even mixtures with up to 22 % legumes in biomass were not limited in their N uptake efficiency compared to non-legume CCs. Winter mineralization of frost-killed CCs can produce variable loads of Nmin in the soil profile. The starting point of N mineralization is controlled by the first frost that killed the CC or by the induced termination. In particular, clover and mustard produced high N litter materials (specifically mustard leaves) that underwent fast degradation. These two CCs showed the highest Nmin losses until the next vegetation period. Additionally, Mix4, with 50 % mustard in litter, showed similar Nmin leaching losses as mustard, but the time point was extended by approximately 6 weeks toward the new seeding. Litter quality in terms of low C:N ratios was not always a good explanation for fast N mineralization. Phacelia had low C:N ratios, particularly in roots and leaves, but showed a low winter N mineralization potential and leaching losses. Future studies could explore the potential of phacelia for use in substances that inhibit the N mineralization cycle. With respect to the investigated parameters of the N cycle, CC mixtures were always comparable to the best-performing monoculture and compensated for the individual weaknesses of monocultures. In order to minimize leaching losses from CC mineralization, future studies on CC mixtures should therefore consider their litter N mineralization potential. It might also be advisable to apply a certain percentage of frost hardy CC that could be easily terminated right before seedbed preparation. The adjustment of CC mixtures to the following crop is a challenge for seed suppliers. The potential of single CCs to support crop rotation diseases must be taken into account, as well as the nutrient demand of the following crop. The applied Mix12 was specifically designed as a pre-crop to maize and responded in the highest silage maize yields. In this example, the mineralization of Mix12 litter fit the continuously rising N demand of maize plants well, particularly in the later stages, when fields can no longer be accessed. Manipulating the plant diversity of CC rotations is a promising approach for optimizing N cycling, reducing N fertilization rates and minimizing leaching losses from agroecosystems.
All metadata and R codes required to reproduce the content of this study are publicly available under Creative Commons Attribution 3.0 Germany. The files are accessible from the Zenodo archive at the following DOI: https://doi.org/10.5281/zenodo.5603221 (Gentsch, 2021).
The supplement related to this article is available online at: https://doi.org/10.5194/soil-8-269-2022-supplement.
NG planned the research activity, participated in fieldwork and provided the statistical evaluation and R codes. StS, DH, RK, BB and DS participated in field and laboratory work and provided metadata. JB, NvW, UF and GG were involved in conceptualization, funding acquisition, project coordination and supervision. NG prepared the manuscript with contributions from all authors.
The contact author has declared that neither they nor their co-authors have any competing interests.
Publisher’s note: Copernicus Publications remains neutral with regard to jurisdictional claims in published maps and institutional affiliations.
This work is part of the BonaRes (Soil as a Sustainable Resource for the Bioeconomy) project CATCHY (Catch-cropping as an agrarian tool for continuing soil health and yield increase). We are grateful to Silke Bokeloh and the whole laboratory team from the Institute of Soil Science, Leibniz Universität Hannover, for assistance with sample preparation and measurements.
This research has been funded by the Bundesministerium für Bildung und Forschung (grant no. 031A559C).
The publication of this article was funded by the open-access fund of Leibniz Universität Hannover.
This paper was edited by Rafael Clemente and reviewed by two anonymous referees.
Abdalla, M., Hastings, A., Cheng, K., Yue, Q., Chadwick, D., Espenberg, M., Truu, J., Rees, R. M., and Smith, P.: A critical review of the impacts of cover crops on nitrogen leaching, net greenhouse gas balance and crop productivity, Glob. Change Biol., 25, 2530–2543, https://doi.org/10.1111/gcb.14644, 2019.
Ad-hoc-AG Boden: Bodenkundliche Kartieranleitung, 5th ed., E. Schweizerbart'sche Verlagsbuchhandlung, Hannover, 438 pp., 2005.
Basche, A. D., Miguez, F. E., Kaspar, T. C., and Castellano, M. J.: Do cover crops increase or decrease nitrous oxide emissions? A meta-analysis, J. Soil Water Conserv., 69, 471–482, https://doi.org/10.2489/jswc.69.6.471, 2014.
Bodner, G., Loiskandl, W., and Kaul, H.-P.: Cover crop evapotranspiration under semi-arid conditions using FAO dual crop coefficient method with water stress compensation, Agr. Water Manag., 93, 85–98, https://doi.org/10.1016/j.agwat.2007.06.010, 2007.
Bodner, G., Nakhforoosh, A., and Kaul, H.-P.: Management of crop water under drought: a review, Agron. Sustain. Dev., 35, 401–442, https://doi.org/10.1007/s13593-015-0283-4, 2015.
Bodner, G., Mentler, A., Klik, A., Kaul, H.-P., and Zechmeister-Boltenstern, S.: Do cover crops enhance soil greenhouse gas losses during high emission moments under temperate Central Europe conditions?, Die Bodenkultur: Journal of Land Management, Food and Environment, 68, 171–187, https://doi.org/10.1515/boku-2017-0015, 2017.
Cameron, K. C., Di, H. J., and Moir, J. L.: Nitrogen losses from the soil/plant system: a review, Ann. Appl. Biol., 162, 145–173, https://doi.org/10.1111/aab.12014, 2013.
Couëdel, A., Alletto, L., Tribouillois, H., and Justes, É.: Cover crop crucifer-legume mixtures provide effective nitrate catch crop and nitrogen green manure ecosystem services, Agriculture, Ecosyst. Environ., 254, 50–59, https://doi.org/10.1016/j.agee.2017.11.017, 2018.
Duchene, O., Vian, J.-F., and Celette, F.: Intercropping with legume for agroecological cropping systems: Complementarity and facilitation processes and the importance of soil microorganisms. A review, Agr. Ecosyst. Environ., 240, 148–161, https://doi.org/10.1016/j.agee.2017.02.019, 2017.
Farneselli, M., Tosti, G., Onofri, A., Benincasa, P., Guiducci, M., Pannacci, E., and Tei, F.: Effects of N sources and management strategies on crop growth, yield and potential N leaching in processing tomato, Eur. J. Agr., 98, 46–54, https://doi.org/10.1016/j.eja.2018.04.006, 2018.
Finney, D. M., White, C. M., and Kaye, J. P.: Biomass Production and Carbon/Nitrogen Ratio Influence Ecosystem Services from Cover Crop Mixtures, Agron. J., 108, 39–52, https://doi.org/10.2134/agronj15.0182, 2016.
Florence, A. M. and McGuire, A. M.: Do diverse cover crop mixtures perform better than monocultures? A systematic review, Agron. J., 112, 3513–3534, https://doi.org/10.1002/agj2.20340, 2020.
Gentsch, N.: Gentsch et al.: Soil nitrogen and water management by winter-killed catch crops. Manuscript for SOIL. Open data and R scripts (1.0), Zenodo [data set and code], https://doi.org/10.5281/zenodo.5603221, 2021.
Gentsch, N., Boy, J., Batalla, J. D. K., Heuermann, D., von Wirén, N., Schweneker, D., Feuerstein, U., Groß, J., Bauer, B., Reinhold-Hurek, B., Hurek, T., Céspedes, F. C., and Guggenberger, G.: Catch crop diversity increases rhizosphere carbon input and soil microbial biomass, Biol. Fertil Soils, 56, 943–957, https://doi.org/10.1007/s00374-020-01475-8, 2020.
Gollner, G., Fohrafellner, J., and Friedel, J. K.: Winter-hardy vs. freeze-killed cover crop mixtures before maize in an organic farming system with reduced soil cultivation, Org. Agr., 10, 5–11, https://doi.org/10.1007/s13165-020-00294-3, 2020.
Heuermann, D., Gentsch, N., Guggenberger, G., Reinhold-Hurek, B., Schweneker, D., Feuerstein, U., Heuermann, M.C., Groß, J., Kümmerer, R., Bauer, B., von and Wirén, N.: Catch crop mixtures have higher potential for nutrient carry-over than pure stands under changing environments, Eur. J. Agron., accepted, 2022.
Heuermann, D., Gentsch, N., Boy, J., Schweneker, D., Feuerstein, U., Groß, J., Bauer, B., Guggenberger, G., and Wirén, N. von: Interspecific competition among catch crops modifies vertical root biomass distribution and nitrate scavenging in soils, Sci. Rep., 9, 1–11, https://doi.org/10.1038/s41598-019-48060-0, 2019.
IUSS Working Group WRB: World Reference Base for Soil Resources 2014. International soil classification system for naming soils and creating legends for soil maps, Food and Agriculture Organization, Rome, 2014.
Jensen, E. S., Peoples, M. B., Boddey, R. M., Gresshoff, P. M., Hauggaard-Nielsen, H., J. R. Alves, B., and Morrison, M. J.: Legumes for mitigation of climate change and the provision of feedstock for biofuels and biorefineries. A review, Agron. Sustain. Dev., 32, 329–364, https://doi.org/10.1007/s13593-011-0056-7, 2012.
Kanders, M. J., Berendonk, C., Fritz, C., Watson, C., and Wichern, F.: Catch crops store more nitrogen below-ground when considering Rhizodeposits, Plant Soil, 417, 287–299, https://doi.org/10.1007/s11104-017-3259-0, 2017.
Kautz, T.: Research on subsoil biopores and their functions in organically managed soils: A review, Renew. Agr. Food Syst., 30, 318–327, https://doi.org/10.1017/S1742170513000549, 2015.
Kaye, J. P. and Quemada, M.: Using cover crops to mitigate and adapt to climate change. A review, Agron. Sustain. Dev., 37, 4, https://doi.org/10.1007/s13593-016-0410-x, 2017.
Li, X., Petersen, S. O., Sørensen, P., and Olesen, J. E.: Effects of contrasting catch crops on nitrogen availability and nitrous oxide emissions in an organic cropping system, Agriculture, Ecosyst.Environ., 199, 382–393, https://doi.org/10.1016/j.agee.2014.10.016, 2015.
Liu, W., Qiao, C., Yang, S., Bai, W., and Liu, L.: Microbial carbon use efficiency and priming effect regulate soil carbon storage under nitrogen deposition by slowing soil organic matter decomposition, Geoderma, 332, 37–44, https://doi.org/10.1016/j.geoderma.2018.07.008, 2018.
Manzoni, S., Taylor, P., Richter, A., Porporato, A., and AAgren, G. I.: Environmental and stoichiometric controls on microbial carbon-use efficiency in soils, New Phytol., 196, 79–91, 2012.
Nicolardot, B., Recous, S., and Mary, B.: Simulation of C and N mineralisation during crop residue decomposition: A simple dynamic model based on the C:N ratio of the residues, Plant Soil, 228, 83–103, https://doi.org/10.1023/A:1004813801728, 2001.
Paul, E. A. (Ed.): Soil microbiology, ecology, and biochemistry, 3rd ed., Elsevier Academic Press, 582 pp., https://doi.org/10.1016/C2009-0-02816-5, 2007.
Paynel, F. and Cliquet, J.: N transfer from white clover to perennial ryegrass, via exudation of nitrogenous compounds, Agronomie, 23, 503–510, https://doi.org/10.1051/agro:2003022, 2003.
R Core Team: R: A Language and Environment for Statistical Computing, R Foundation for Statistical Computing, Vienna, Austria, 2020.
Rinnofner, T., Friedel, J. K., Kruijff, R. de, Pietsch, G., and Freyer, B.: Effect of catch crops on N dynamics and following crops in organic farming, Agron. Sustain. Dev., 28, 551–558, https://doi.org/10.1051/agro:2008028, 2008.
Romdhane, S., Spor, A., Busset, H., Falchetto, L., Martin, J., Bizouard, F., Bru, D., Breuil, M.-C., Philippot, L., and Cordeau, S.: Cover Crop Management Practices Rather Than Composition of Cover Crop Mixtures Affect Bacterial Communities in No-Till Agroecosystems, Front. Microbiol., 10, 1618, https://doi.org/10.3389/fmicb.2019.01618, 2019.
Selzer, T. and Schubert, S.: Nutrient uptake of catch crops under non-limiting growth conditions, J. Plant Nutr. Soil Sci., 184, 709–722, https://doi.org/10.1002/jpln.202100142, 2021.
Thorup-Kristensen, K.: Are differences in root growth of nitrogen catch crops important for their ability to reduce soil nitrate-N content, and how can this be measured?, Plant Soil, 230, 185–195, https://doi.org/10.1023/A:1010306425468, 2001.
Thorup-Kristensen, K., Magid, J., and Jensen, L. S.: Catch crops and green manures as biological tools in nitrogen management in temperate zones, Adv. Agron., 79, 227–302, 2003.
Unger, P. W. and Vigil, M. F.: Cover crop effects on soil water relationships, J. Soil Water Conserv., 53, 200–207, 1998.
Vogeler, I., Hansen, E. M., Thomsen, I. K., and Østergaard, H. S.: Legumes in catch crop mixtures: Effects on nitrogen retention and availability, and leaching losses, J. Environ. Manag., 239, 324–332, https://doi.org/10.1016/j.jenvman.2019.03.077, 2019.
Vukicevich, E., Lowery, T., Bowen, P., Úrbez-Torres, J. R., and Hart, M.: Cover crops to increase soil microbial diversity and mitigate decline in perennial agriculture. A review, Agron. Sustain. Dev., 36, 48, https://doi.org/10.1007/s13593-016-0385-7, 2016.
White, C. M., DuPont, S. T., Hautau, M., Hartman, D., Finney, D. M., Bradley, B., LaChance, J. C., and Kaye, J. P.: Managing the trade off between nitrogen supply and retention with cover crop mixtures, Agr. Ecosyst. Environ., 237, 121–133, https://doi.org/10.1016/j.agee.2016.12.016, 2017.
Wickham, H.: ggplot2: Elegant Graphics for Data Analysis, Springer-Verlag New York, 2016.