the Creative Commons Attribution 4.0 License.
the Creative Commons Attribution 4.0 License.
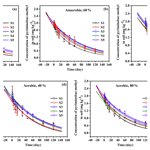
Environmental behaviors of (E) pyriminobac-methyl in agricultural soils
Wenwen Zhou
Haoran Jia
Lang Liu
Baotong Li
Yuqi Li
Meizhu Gao
E or pyriminobac-methyl (EPM), a pyrimidine benzoic acid esters herbicide, has a high potential as weedicide; nevertheless, its environmental behaviors are still not well understood. In this study, we systematically investigated, for the first time, the adsorption–desorption, degradation, and leaching behaviors of EPM in agricultural soils from five exemplar sites in China (Phaeozems – S1; Anthrosol – S2; Ferralsol – S3; Alisol – S4; Plinthosol – S5) through laboratory simulation experiments. Our results show that the EPM adsorption–desorption results were well fitted by the Freundlich model (R2>0.9999). In the analyzed soils, the Freundlich adsorption (i.e., Kf ads) and desorption (i.e., Kf des) coefficients of EPM varied between 0.85 and 32.22 mg L kg−1 and 0.78–5.02 mg L kg−1, respectively. The mobility of EPM in soils S1–S5 was categorized as immobile, slightly immobile, highly mobile, slightly mobile, and slightly mobile, respectively. Moreover, the degradation of EPM reflected first-order kinetics, where its half-life ranged between 37.46 and 66.00 d, depending on the environmental conditions, and abiotic degradation was predominant in the degradation of this compound. Overall, the high leaching ability and desorption capacity of EPM were accompanied by a low adsorption capacity, and there were no significant relationships between pH and the leaching rate of EPM in the five types of soils. In contrast, the organic matter content, cation exchange capacity, and soil clay content were the main components responsible for the observed leaching rates. We found that EPM degrades easily, has a high adsorption affinity, and a low mobility in S1, which results in a low contamination risk for groundwater systems. On the contrary, this compound degrades slowly in S2, S3, S4, and S5, due to a low adsorption affinity and moderate mobility, which results in a high contamination risk for groundwater systems. Therefore, our results may serve as a reference for evaluating the risks involved in the increasingly wide application of this compound.
- Article
(876 KB) - Full-text XML
-
Supplement
(606 KB) - BibTeX
- EndNote
Herbicides are usually applied to chemically control the growth of weeds associated with different types of crops, both in China and worldwide (Barchanska et al., 2021; Brillas, 2021). Unfortunately, with the applications of weedicides, they have been detected outside of their original application sites, meaning that they contribute to environmental contamination and food safety problems (Jiang et al., 2018; Perotti et al., 2020; Marvin and Bouzembrak, 2020). Therefore, the Guidelines for Good Herbicide Application (Ny/T, 2011) and the National Food Safety Standard – Maximum Residue Limits for Pesticides in Food have been established in China (Gb, 2021), which contain the maximum residual limit (MRL) and acceptable daily intakes (ADIs) of 548 commonly used pesticides; for example, the MRL of pyriminobac-methyl (PM) in paddy rice and brown rice is 0.2 and 0.1 ppm (parts per million), respectively, and the ADI of PM is 0.02 ppm. Most studies have reported that, with the increasing use of glyphosate (a nonselective herbicide), especially in tea plantations (the detected MRL of glyphosate is 4.12 ppm bigger than the limited value 1 ppm) and aquatic systems, the problem of excessive residues of glyphosate has attracted more and more attention, raising potential environmental threats and public health concerns (Liu et al., 2021a; Luo et al., 2019; Huang et al., 2016). Importantly, the environmental fate of herbicides in soil mainly depends on the adsorption–desorption, degradation, and leaching processes. In fact, herbicides can be transferred from soil to groundwater through surface runoff or leaching, resulting in groundwater pollution (Cueff et al., 2020; Gawel et al., 2020). Furthermore, the adsorption–desorption rate and the degradation capability of herbicides regulate the migration of herbicides; thus, the groundwater ubiquity score (GUS) can be used to evaluate their ecological and environmental safety (Acharya et al., 2020; Liu et al., 2021b). However, few scholars have assessed the effects of soil properties on the adsorption–desorption, degradation, and leaching behaviors of weedicide, especially the environmental consequences of these changes.
PM (methyl-2-(4,6-dimethoxy-2-pyrimidinyloxy)-6-(1-methoxyiminoethyl) benzoate; Fig. S1 in the Supplement), is composed of a mixture of its (E), which is isomer (I), and (Z), which is isomer (II), as the active ingredient due to its chemical structure that contains oxime (Song et al., 2010), a mixture of two isomers (I and II) in a >9:1 (major minor) ratio, which was developed from sulfonylurea by Kumiai Chemical Industry Co., Ltd. in 1996 (Tokyo, Japan; Tamaru and Saito, 1996). Tamaru et al. (1997) reported that (E), isomer (I), has been confirmed to restrain the plant enzyme acetolactate synthase (ALS) and prevent branched chain amino acid biosynthesis, and the (E), or pyriminobac-methyl (EPM), showed stronger soil adsorption and weaker hydrophilic properties than (Z), or pyriminobac-methyl (ZPM); thus, EPM was selected as the best compound to develop a commercial weedicide, which is commonly used to control the growth of sedges and both gramineous and annual weeds. The chemistry of EPM is well understood; the octanol–water partition coefficient is 2.31 (low) at pH 7 and 20 ∘C, the solubility in the water is 9.25 mg L−1 (low) at 20 ∘C, and the vapor pressure is just Pa (low) at 20 ∘C (Lewis et al., 2016). A distinct advantage of EPM as a weedicide is that this compound has an herbicidal activity 1.5–2 times higher and requires an application rate that is one-fifth to one-tenth lower than bensulfuron-methyl (a broad-spectrum herbicide) on Echinochloa crus-galli and Leptochloa chinensis (Iwakami et al., 2015; Shibayama, 2001; Song et al., 2010). Notably, EPM can prevent the growth of E. crus-galli and L. chinensis populations and suppress them effectively over long periods, while being nontoxic and eventually increasing the yield of paddy rice and subsequent crops (e.g., rape, cabbage, Astragalus smicus, wheat, and potato) (Iwafune et al., 2010; Qin et al., 2017; Tang et al., 2010; Yoshii et al., 2020). Nevertheless, few studies have discussed the environmental behaviors of EPM after it was widely used as herbicide in the farming industry.
Most former investigations on EPM as a weedicide mainly focused on the photo-transformation in water and low temperature storage stability in paddy rice. Inao et al. (2009) demonstrated that the photoconversion of PM in water is the main fate, and the main process is EPM ZPM, which reached equilibrium after approximately 4.5 h. Furthermore, the EPM ZPM ratio is about . Another researcher found that even if proper water management to prevent EPM surface runoff from paddy fields was practiced, a significant amount of EPM components were discharged into drainage channels through percolation (Sudo et al., 2018). Indeed, the harm of weedicide leaching has been frequently reported in groundwater. Several studies have indicated that the leaching risk potential of herbicides to groundwater is positively correlated with its mobility in soil (Chen et al., 2021; Wang et al., 2019; Silva et al., 2019; Kaur et al., 2021; Willett et al., 2020). Guimares et al. (2019), who found that hexazinone (herbicide) proved to be a potential contaminant of groundwater and metribuzin (herbicide) presented high leaching in the soil profile. In addition to metribuzin, atrazine was found to have accumulated in algal cells, which indicates that herbicide pollution might eventually affect the marine food web and even threaten the seafood safety of human beings (Yang et al., 2019). On the other hand, Kolakowski et al. (2020) and Mehdizadeh et al. (2021) reported that the residue levels of herbicides which were taken up by plants and the level of risk to consumers depend on the application technique, the environmental conditions, the stage of growth of plants, the volume of use, water quality, and the use of coadjuvants. EPM has also proved to be safe in rice. Jia et al. (2020) showed that the detected MRL of EPM in paddy rice is 0.0092 ppm, which is far less than the limited value of 0.2 ppm. Hence, previous knowledge of the physicochemical properties of soils cultivated with crops is essential for recommending the use of these herbicides in weed management.
In the paddy rice field, the half-life of the EPM calculated from 4.0 to 19.3 d (half-life ≤ 30 d; easily degradable; Gb, 2014c) was monitored in the Lake Biwa basin, Japan (Iwafune et al., 2010), and the sorption constants of the OC (soil organic carbon; Koc) values ranged from 372 to 741 (200<Koc≤1000; partial difficulty in absorbing the compound; Gb, 2014a) in soils in the cities of Habikino and Ushiku in Japan, indicating that EPM is a low-persistence herbicide, which results in a lower contamination risk for groundwater systems (Inao et al., 2009). The Japanese Environment Agency sets limits for residues in paddy rice discharge water by allowing for a 10-fold dilution in river water and applying the drinking water limit of EPM of 200 µg L−1 (Hamilton et al., 2003). In China, EPM has been registered to control grassy weeds in paddy rice and brown rice fields (Gb, 2021). Nevertheless, the effects of soil properties on the adsorption–desorption, degradation, and leaching behaviors of EPM have rarely been reported.
A number of researchers have reported that the soil matrix is a highly complicated system in which environmental processes (e.g., the sorption–desorption and leaching of herbicides) are affected by multiple factors, including the soil organic matter (OM) content, pH, cation exchange capacity (CEC), microbial or chemical degradation, chemical type, environmental conditions (e.g., temperature, humidity, and rainfall), and texture (Alonso et al., 2011; Rao et al., 2020; Xie et al., 2020; W. Zhou et al., 2019). Nevertheless, soil organic or inorganic colloids and pH (pH < pKa neutral state and pH > pKa negative charge) can influence soil–herbicide interactions. In this context, the leaching of anionic compounds is likely (Pérez-Lucas et al., 2020). Moreover, the leaching of herbicides in soil and the associated risk of water pollution are both affected by sorption and desorption (Xie et al., 2020).
Until now, the environmental fate of EPM in soils has not been studied in detail. Clarifying the adsorption and transport of EPM in soil is very important for the protection of surface water and groundwater from EPM pollution. Hence, this study aimed at (1) gaining an essential understanding of the adsorption–desorption, degradation, and leaching behaviors of EPM in agricultural soils through laboratory simulation experiments, (2) determining the effects of soil properties on the above behaviors in agricultural soils, and (3) conducting a basic evaluation of the safety and applicability of EPM in the environment. Overall, our results provide a scientific basis for the prevention or, at least, minimization of the possible effects of EPM on groundwater and for modeling the fate of EPM in the environment and the potentially associated risks.
2.1 Chemicals
EPM (99.0 %; chemical formula – C17H19N3O6; structure shown in Fig. S1) was obtained from ZZBIO Co., Ltd. (Shanghai, China). Moreover, we used only organic solvents of a chromatographic grade (Sigma-Aldrich, Germany). EPM was dissolved in acetonitrile, obtaining a 1000 mg L−1 test in a mother liquor. Moreover, a standard EPM working solution (0.01–5.00 mg L−1) was prepared by diluting the stock solution with a CaCl2 solution (0.01 mol L−1), which was used as an electrolyte to maintain a constant ionic strength and reduce the cationic exchange.
In March 2020, five different soils were sampled from the surface layer (0–20 cm) of paddy fields located in five Chinese provinces: Phaeozems (S1, from Heilongjiang), Anthrosol (S2, from Zhejiang), Ferralsol (S3, from Jiangxi), Alisol (S4, from Hubei), and Plinthosol (S5, from Hainan). The soil samples were all air-dried, ground, and passed through a 2 mm sieve before being used. Afterward, standard soil testing methods were applied to define the basic physicochemical properties of the soils (Table S1 in the Supplement; Gee, 1986; Jackson, 1958; Nelson, 1985), which were then classified based on the system of the World Reference Base for Soil Resources (WRB; L'huillier, 1998). Interestingly, the EPM residues in the analyzed soils were always below the detection limit.
2.2 Soils samples
The batch equilibration method suggested by the GB 31270.4-2014 guidelines of adsorption/desorption in soils for these soils (Gb, 2014a) was applied to conduct adsorption–desorption experiments. First, for the adsorption kinetics tests, each soil sample (2.0 g) was introduced in a centrifuge tube containing 10 mL of a EPM aqueous solution (1 mg L−1). For each of these tubes, we also analyzed a blank tube (which contained no herbicide) and a control tube (which contained no soil). All the tubes were then shaken by an oscillator at 25 ∘C ± 1 ∘C for different time intervals of 0.5, 1, 2, 4, 6, 8, 12, 16, 20, and 24 h.
The desorption kinetics were analyzed instead by taking 5 mL of supernatant from each tube after the adsorption equilibration and by replacing them with an equal volume of CaCl2 solution (which contained no EPM). A micro-vortex mixer was used to thoroughly mix the resulting solution, and an oscillator was used to shake it at 25 ∘C ± 1 ∘C for several time intervals, i.e., 0.5, 1, 2, 4, 6, 8, 12, and 24 h. Finally, for the high-performance liquid chromatography mass spectrometry (HPLC-MS/MS) analyses, the samples were centrifuged for 10 min at 2400× g, and the supernatants were filtered through 0.22 µm mixed cellulose ester filter membranes.
The adsorption–desorption equilibrium time of EPM in the five soils was 24 h (Fig. 1); moreover, the initial EPM concentrations adopted for these experiments were 0.01, 0.10, 0.50, 1.00, and 5.00 mg L−1. The concentration of EPM in the supernatant was determined after centrifugation. Then, the amount of adsorbed–desorbed EPM in each soil was calculated based on the concentration of EPM in the solution before and after the adsorption–desorption process. The supernatant removed after the adsorption experiments was replaced with 5 mL of CaCl2 containing no EPM; then, the tubes were shaken for 24 h and centrifuged. Finally, the EPM concentration was determined based on the supernatant collected after this procedure. Considering the results of preliminary experiments and with the aim of desorbing the majority of the adsorbed EPM, we decided to repeat the desorption process at least three times.
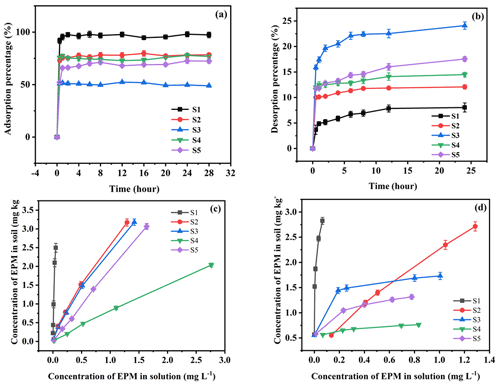
Figure 1Adsorption (a) and desorption (b) kinetic curves and adsorption (c) and desorption (d) isothermal curves of EPM in five different agricultural soils (S1 to S5, as defined in Table 1). Values are the means ± standard error (n=3).
2.3 Degradation experiments
By following the GB 31270.1-2014 guidelines (Gb, 2014c), we performed a series of EPM soil degradation experiments. To ensure aerobic conditions, 20 g of each type of agricultural soil were weighed and introduced in 250 mL Erlenmeyer flasks (in three replicates). Ultrapure water was added during the subsequent cultivation process in order to maintain the soil water content at 60 % of the maximum water holding capacity. We then spiked each soil sample with 400 µL of the 100 mg L−1 EPM working solution (achieving an initial concentration of 2 mg kg−1 in the soil, where the water-soluble, organic solvent volume was ≤ 1 %) and then cultured in the dark in an incubator kept at 25 ± 1 ∘C. Subsequently, we collected three parallel subsamples on 0, 1, 2, 4, 6, 10, 15, 30, 45, 60, 90, and 120 d, and the EPM content was determined by HPLC-MS/MS on the respective days of collection. The amount of water in the Erlenmeyer flasks was periodically adjusted during the culturing process with the aim of retaining the original water-holding state. Each treatment was done in triplicate, totalizing 60 samples per treatment (five soil samples per treatment per sampling day; 12 sampling days in total), The following experiment was done in the same way.
Another set of experiments was conducted under anaerobic conditions. In this case, we first cultured the soil samples for 30 d and then added a 2 cm thick water layer to each of them. To maintain the desired conditions, N2 was continuously introduced into the culture system. The soil samples were subsequently moved into an incubator and cultivated in the dark at 25± 1 ∘C. Finally, three parallel subsamples were collected on 0, 1, 2, 4, 6, 10, 15, 30, 45, 60, 90, and 120 d, and the EPM content was determined by HPLC-MS/MS on the respective days of collection.
A set of degradation experiments was performed under sterilized conditions. With this objective, the sterilized soils (20 g each) were weighed and introduced in 250 mL Erlenmeyer flasks in three replicates. Notably, in order to keep the soil water content at 60 % of the maximum water holding capacity, sterile water was added during the cultivation process. Then, each soil sample was spiked with 400 µL of the 100 mg L−1 EPM working solution, achieving an initial concentration of 2 mg kg−1 (the water-soluble, organic solvent volume was ≤1 %). The samples were then moved into an incubator and cultured in the dark at 25±1 ∘C. In total, three parallel subsamples were collected on 0, 1, 2, 4, 6, 10, 15, 30, 45, 60, 90, and 120 d, and the EPM content was determined by HPLC-MS/MS on the respective days of collection.
These experiments were done under different soil moisture conditions and aerobic conditions and at a EPM fortification level of 2 mg kg−1. After adjusting their moisture by adding water (water percentages of 40 %, 60 %, and 80 % of the total volume), the soils were incubated in the dark at 25±11 ∘C. During this last phase, we regularly added ultrapure water to keep the moisture at 40 %, 60 %, and 80 %.
2.4 Leaching experiments
The herbicide leaching process was investigated by following the GB 31270.5-2014 guidelines (Gb, 2014b). PVC columns (length of 35 cm; internal diameter of 4.5 cm), each hand-packed with 600–800 g of one soil type, were used to observe the downward movement of the herbicide. Notably, the top 3 cm and the bottom 2 cm were filled with quartz sand (to minimize soil disturbance) and glass wool and sea sand (to avoid soil loss). After packing each column, we removed any air still present in the column by adding 0.01 mol L−1 CaCl2; moreover, the excess water was eliminated by gravity. The pore volume (PV) was determined by subtracting the volume of water leached from that of the water added. Subsequently, 1 mL of acetonitrile solution containing 200 µg mL−1 of the herbicide (spiking level of 1 µg g−1) was added to the top of each column. Afterward, the adsorption equilibrium was achieved by infiltrating 700 µL of 100 mg L−1 EPM solution into the soil surface and leaving it to rest for 24 h. To simulate rainfall leaching, 2000 mL of the 0.01 mol L−1 CaCl2 solution (21 mL h−1) were added into the soil column at a peristaltic pump speed of 250 mL 12 h−1. The leachate was collected every 8 h with a conical flask. Subsequently, each soil column was extracted, cut into three parts (length of 10 cm), and analyzed by HPLC-MS/MS on the same day. The total mass of the leachate and soil fractions along the soil column was determined, together with the EPM and water contents within each of them.
2.5 Extraction and final analyses
The soil samples were transferred to centrifuge tubes and 10 mL of acetonitrile (containing 0.1 % of ammonia water) were added to each of them for extracting EPM. After shaking the tubes for 5 min, we added 2 g of NaCl and 3 g of MgSO4. Then, the tubes were capped and shaken again for 1 min and centrifuged at 2400× g for 5 min. The supernatant (1.5 mL) was transferred into a 2.5 mL single-use centrifuge tube that was already containing the sorbent (50 mg C18+150 mg MgSO4). Afterward, all the samples were shaken again for 1 min and centrifuged at 5000 rpm (revolutions per minute) for 5 min (H. R. Jia et al., 2019). Finally, the resulting supernatant was extracted with a sterile syringe, passed through a 0.22 µm organic membrane filter, and poured into vials for an HPLC system (1260 series; Agilent Technologies, Inc., USA) equipped with a triple quadrupole mass spectrometer (6460C series; Agilent Technologies, Inc., USA) using the positive ion mode in a multiple reaction monitoring (MRM) mode analysis. The instrument parameters for the Agilent 6460C QQQ HPLC-MS/MS analysis are as follows: the flow rate was maintained at 0.2 mL min−1, and the column (Agilent ZORBAX Eclipse XDB-C18; length 150 mm, inner diameter 4.6 mm, and 5 µm coating) was heated to 35 ∘C. The mobile phase A was water, which consisted of 0.1 % formate and mobile phase B was acetonitrile. The gradient condition was 0.0–0.5 min at 20 % B, 0.5–1.0 min at 20 %–80 % B, 1.0–4.0 min at 80 % B, and 4.0–5.0 min at 20 % B. The mass spectrometer was operated in an electrospray ionization positive range with MRM scanning mode, a dry gas temperature at 500 ∘C, the ion source temperature at 150 ∘C, the desolvation gas flow at 1000 L h−1, the capillary voltage at 2500 V and the cone voltage at 18 V, while the collision gas was argon, the dwell time was set to 50 ms, and the collision pressure was at 58 eV. Detailed information on the determination parameters of the chromatographic method, i.e., repeatability, reproducibility, recovery, measurement uncertainty, detection limit, and limit of quantification, are shown in the Supplement (Figs. S2–S4 and Tables S2–S4).
The efficiency of the EPM extraction during the adsorption–desorption, degradation, and leaching experiments was evaluated based on the results of the recovery experiments. The average recovery rates of EPM in the adsorption–desorption experiments, at initial spiked concentrations of 0.1 and 1.0 mg kg−1 in the soils, varied between 94.3 % and 102.4 % (relative standard deviation – RSD = 1.1 %–3.8 %). Meanwhile, the average recovery rates of EPM in soil in the degradation experiments, at initial spiked concentrations of 0.01, 0.2, and 2.0 mg kg−1 in the soils, ranged between 92.6 % and 106.0 % (RSD = 1.1 %–2.9 %). Furthermore, the average recovery rates of EPM at the initial spiked concentrations of 0.0001, 0.01, and 0.1 mg L−1 in the supernatant of soils were 88.7 %–107.9 % (RSD = 1.7 %–4.9 %). Furthermore, the average recovery rates of EPM in the leaching experiments at initial spiked concentrations of 0.05 and 1.0 mg kg−1 in the soils were 95.8 %–109 % (RSD = 1.6 %–4.4 %).
2.6 Data analysis
The relationship between the concentrations of EPM sorbed in the soil and in the aqueous solution during the sorption–desorption equilibrium was described through the linear (Eq. 1) and Freundlich (Eq. 2) models as follows (Azizian et al., 2007; Yang et al., 2021):
where Cs (mg kg−1) indicates the adsorption of EPM in the soil, Ce (mg L−1) is the EPM concentration in the solution during the adsorption equilibrium, C (mg kg−1) is the amount of soil adsorption when the EPM concentration was 0 during the adsorption equilibrium, K (mL g−1) and Kf (mg L kg−1) are the adsorption–desorption constants of the linear and Freundlich models, respectively ( in the adsorption–desorption process), and is the adsorption empirical constant (which provides information about the nonuniformity of the adsorbent surface).
For the isothermal sorption tests, the amount of EPM adsorbed in the soil was estimated using the subtractive method (Eq. 3) as follows:
where C0 (mg L−1) is the amount of soil adsorption when the concentration of EPM was zero during the adsorption equilibrium, m is the soil mass (2.0 g), and V is the solution volume (10 mL).
The amount of EPM retained by the soil after desorption was obtained instead by using Eq. (4), while the hysteresis index (H) was estimated by applying Eq. (5) as follows (Fan et al., 2021; Y. Zhang et al., 2020):
where Csj (mg kg−1) is the concentration of EPM adsorbed by the soil after the jth desorption (i= 1–5), Cej (mg L−1) is the EPM concentration in the supernatant after the jth desorption, H is the hysteresis coefficient, and and 1 are the empirical adsorption and desorption constants, respectively.
The distribution coefficient (Kd) was calculated based on the distribution ratio of EPM in the water–soil system by using Eq. (6) as follows (Carballa et al., 2008; Ternes et al., 2004):
The sorption constants of the OC (KOC) and OM (KOM) contents were calculated through Eqs. (7) and (8) (Rae et al., 1998; Zhang et al., 2011), respectively. Moreover, the Gibbs free energy change of sorption (ΔG; kJ mol−1; C. S. Jia et al., 2019) and the GUS (Gustafson, 1989) were calculated as follows:
where the OM percent and OC percent represent the soil OM and OC contents, respectively, R is the molar gas constant (8.314 J K−1 mol−1), T (K) is the absolute temperature, and t is the half-life (in days) given by Eq. (12). Organic contaminants were categorized into five types, namely highly adsorbed compounds (KOC>20000), sub-highly adsorbed compounds (5000<KOC≤ 20 000), medium-adsorbed compounds (1000<KOC≤5000), partial-difficulty adsorbed compounds (200<KOC≤1000), and difficultly adsorbed compounds (KOC≤200) (Gb, 2014a).
The degradation data relative to herbicides in soil could be successfully fitted to a first-order kinetic model (Eq. 11), which was previously used in similar studies as follows (Bailey et al., 1968; Liu et al., 2021b; Ou et al., 2020):
where Ct (mg kg−1) and C0 (mg kg−1) are the concentrations of EPM in the soil at incubation times of t (d) and 0 (d), respectively, while k is the first-order rate constant (d−1).
The half-life (t) to be used in above model was calculated through Eq. (12) as follows (Yin and Zelenay, 2018):
A total of four categories of herbicide degradability were defined, i.e., easily degradable (t), moderately degradable (30< t), slightly degradable (90<t), and poorly degradable (t) (Gb, 2014c).
Based on the content of EPM in different sections of the soil columns and in the leachate (Eq. 13) (Gb, 2014b), we were able to calculate the leaching rate of EPM as follows:
where Ri (%) is the ratio of EPM content in each soil section or in the leachate to the total added amount, mi (mg) is the mass of EPM in each soil section (where i= 1, 2, 3, and 4, representing the 0–10, 10–20, and 20–30 cm soil sections and in the leachate, respectively), and m0 (mg) is the total added amount of EPM (m0=0.02 mg). Regarding the mobility scheme, we defined the following Ri ranges: class 1 (immobile; R1>50 %), class 2 (slightly mobile; %), class 3 (mobile; R3+R4>50 %), and class 4 (highly mobile; R4>50 %; Gb, 2014b).
The data fittings (to the linear and Freundlich models for the adsorption isotherms and to the simple first-order kinetic model for degradation) were conducted with OriginPro 8.05 (Originlab Corp., Northampton, USA). All the values reported here were calculated as the means of three replicates; furthermore, the differences between these means were statistically analyzed through Duncan's multiple range test, while their reciprocal relationships were determined though a Spearman's correlation analysis using SPSS Statistics 22.0 software (IBM SPSS, Somers, USA).
3.1 Adsorption–desorption kinetics
The adsorption and desorption kinetic curves of EPM in different types of agricultural soils are shown in Fig. 1. After EPM had been in contact with the soil solution for 1 h, the concentration of EPM exhibited a sharp drop (from 0 % to 95.35 %, 75.45 %, 51.57 %, 77.41 %, and, finally, 65.84 % between S1 and S5). This event corresponded to the fast sorption phase. After 2–8 h, the EPM soil system entered the slow adsorption stage, and there was a gradual increase in the sorption of EPM. This last process reached an equilibrium state of EPM sorption after 8 h, which was reflected by stable concentrations of EPM. The sorption of EPM decreased from the Phaeozems (S1; 97.99 %), the Anthrosol (S2; 79.69 %), Alisol (S4; 77.81 %), and Plinthosol (S5; 72.57 %) to Ferralsol (S3; 52.35 %; Fig. 1a). This trend reflected the soils' OM contents. Previous studies have also found that the sorption of organic chemicals in soils is mainly related to their OM contents (Xu et al., 2021; Z. Zhou et al., 2019).
The desorption equilibration of EPM in soil was slightly slower, and a hysteresis effect was observed. The rapid and slow desorption stages occurred between 0–2 and 2–12 h, respectively; afterward, the concentration of EPM remained unchanged until the desorption process reached its equilibrium state (within 24 h). Based on these data, we defined 24 h as the period of EPM adsorption–desorption. The desorption value of EPM observed in our experiments after 24 h increased from the Phaeozems (S1; 8.04 %), the Anthrosol (S2; 12.07 %), Alisol (S4; 14.48 %), and Plinthosol (S5; 17.55 %) to Ferralsol (S3; 24.08 %; Fig. 1b).
The sorption of OM in soil typically occurs during the rapid reaction and slow equilibrium phases (Calvet, 1989). The tendency of sorbed hydrophobic organic pollutant to become more strongly bound with increasing organic matter (OM) contents of the soils is well demonstrated for EPM. This is consistent with previously reported observations that for sorbents with an organic carbon content greater than 0.1 %. A highly significant positively correlation was found between the adsorption constants of nonpolar or weakly polar organic compounds and the OM of soils (Schwarzenbach and Westall, 1981; Chefetz et al., 2004). The main reason is that the OM of soils has special binding sites with organic pesticide molecules. With the increase in OM content, the adsorption sites also increased, thus increasing the herbicide adsorption capacity (Stevenson, 1972; Ahmad et al., 2001; Delle Site, 2001; Chianese et al., 2020). The role of different components of the OM in determining herbicide sorption has been clearly observed in previous studies. Major (1962) had speculated that the oily constituent of the OM might be responsible for uptake of nonionic compounds by the soil. The existence of such a lipid phase was supported by Schnitzer and Khan (1972), who reported the presence of fatty acids and alkanes at the surface of the OM resulting from long alkyl chains projecting from the surface. They suggested that interactions such as hydrogen bonding might be important in uptake of nonionic contaminants by this lipid fraction. The hydrophobicity of the OM has generally been reported to originate from aromatic and alkyl domains of the organic matter component (Ahmad et al., 2001). Murphy and Zachara (1995) suggested the presence of heterogeneous sorption sites on the OM and considered the most hydrophobic domains to be the most energetic and strong binding sites. Therefore, the reduction in the EPM content in the solution before and after the experiment was likely due to soil sorption. According to the above results, the soil sorption rate was inversely proportional to the soil desorption rate toward EPM.
3.2 Adsorption–desorption isotherms
Nonlinear adsorption–desorption isotherms of EPM were observed (Fig. 1). When the concentration of EPM was low, this compound was preferentially adsorbed by OM (which has a strong adsorption capacity); meanwhile, soils with higher OM contents (e.g., Phaeozems; S1) desorbed EPM slowly. The positive relationship between sorption and OM has been reported previously (Hochman et al., 2021; Obregón Alvarez et al., 2021; Patel et al., 2021). Moreover, the adsorption ability of EPM has been found to be high, similar to those of other herbicides (e.g., chlorsulfuron, imazamethabenz methyl, flumetsulam, and bispyribac sodium; Kalsi and Kaur, 2019; Medo et al., 2020; Spadotto et al., 2020). Generally, a low mobility of herbicides in soil is related to a high sorption constant. Hence, the EPM contained in the soils tested in this study (excluding the Phaeozems; S1) is likely to have been polluting the groundwater and surface water of the respective areas of origin.
OM adsorption in soil is currently explained mainly by partitioning and adsorption site theories (Martins and Mermoud, 1998), which are well described by the linear and Freundlich isotherm models, respectively. Our isothermal sorption and desorption data were thus fitted to these two models, and the obtained fitting parameters are listed in Table 1. The average R2 value for the linear model (0.9950) was smaller than that for the Freundlich model (0.9999); moreover, the C values obtained for the Plinthosol (S5; ) by fitting the data to the linear model were negative (Table 1) and did not meet the experimental requirements, indicating that this type of model was not suitable for this experiment. Meanwhile, the sorption site theory was found to more accurately describe the sorption–desorption process, and the Freundlich model provided a more accurate description of the EPM sorption–desorption characteristics observed in this study.
Generally, larger Kf ads values correspond to higher sorption capacities (Carneiro et al., 2020; Khorram et al., 2018; Silva et al., 2019). Here, the Kf ads values of EPM ranged between 0.85 (in S4) and 32.22 (in S1; mg L kg−1), while the values ranged between 0.80 (S1) and 1.06 (S5; Table 1). In brief, S5 showed an S-type adsorption isotherm (since ), while S1, S2, S3, and S4 showed an L-type adsorption isotherm (since ). In this study, the H values of EPM ranged between 0.013 (Phaeozems; S1) and 0.845 (Ferralsol; S3). Since the H values were <0.7 in S1, S2, S4, and S5, these particular soils showed a positive hysteresis in that the desorption rate of EPM was lower than its sorption rate. Meanwhile, since the H values in S3 were between 0.7 and 1.0, the sorption and desorption rates were in equilibrium. S3 did not exhibit any obvious hysteresis. Similar results were reported that hysteresis was absent when 0.7<H<1 (Gao and Jiang, 2010; Yue et al., 2017; Barriuso et al., 1994).
Soil physicochemical properties are important factors influencing herbicide adsorption behaviors (Urach Ferreira et al., 2020; Wei et al., 2020). We determined the relationship between the Freundlich adsorption–desorption constant and the soil physicochemical (soil pH, CEC, soil clay content, OM content, and OC content) properties and carried out a linear correlation analysis based on the experimental data fitting (Table S5 in the Supplement). The results showed that the soil pH, CEC, soil clay content, OM content, and OC content were positively correlated with Kf des and Kf ads (slope >0). In soils, some polar contents, ionizable groups, and the CEC tend to increase during OM humification (Calvet, 1989; Meimaroglou and Mouzakis, 2019; Rae et al., 1998). This mechanism possibly explains the adsorption of EPM in soils high in OM and CEC. Our findings agree with those of Acharya et al. (2020) and García-Delgado et al. (2020), who stated that the soil humic acid and clay fractions (high in OM and CEC and possessing a high number of active sites) are capable of intense EPM adsorption; in contrast, the soil coarse sand fraction (low in OM and CEC) is characterized by a weaker EPM adsorption. Notably, the soil with the highest fumigant adsorption capacity was also possibly that with the highest OM abundance and CEC. For example, strong linear and positive correlations have been found between the adsorption–desorption of benzobicyclon hydrolysate and the soil clay content, OC content, OM content, and CEC, while moderate linear and negative correlations were observed between those processes and the soil pH (Rao et al., 2020).
The KOC value is typically used to indicate the EPM sorption capacity of a soil (FAO, 2000; Xiang, 2019; see Table 2). EPM was somewhat difficultly adsorbed in S2, S3, S4, and S5; this aspect was reflected by the KOC values, which ranged between 200 and 1000. However, in S1 the KOC values ranged between 1000 and 5000, indicating a medium adsorbance of EPM in this soil. Overall, an increasing trend in the mobility of EPM was observed from the Phaeozems (S1) to the Anthrosol (S2), Alisol (S4), Plinthosol (S5), and Ferralsol (S3). We, hence, infer that a relatively low soil adsorption capacity is linked to a relatively high mobility of EPM in that soil.
Table 2Empirical constants, Gibbs free energy, and groundwater ubiquity score (GUS) for the adsorption of EPM in five agricultural soils.
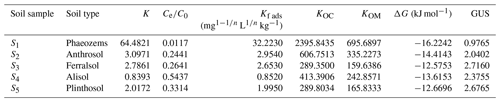
The degree of spontaneity of the adsorption process can be quantitatively evaluated based on variations in the ΔG values, where negative ΔG values generally indicate that an adsorption process is spontaneous and exothermic (Nandi et al., 2009). Notably, the change of free energy linked to physical adsorption is smaller than that linked to chemisorption. The former is in the range of −20 to 0 kJ mol−1, while the latter is in the range of −80 to −400 kJ mol−1 (Bulut and Aydın, 2006; Yu et al., 2004). We found that the ΔG values relative to EPM adsorption in all soils were comprised between −16.2242 and −12.5753 kJ mol−1. Therefore, the adsorption we observed in our experiments can be regarded as typically spontaneous and exothermic physical adsorption (Table 2).
3.3 Degradation of EPM in soil
To investigate the effects of aerobic and anaerobic microorganisms on EPM degradation, we sterilized the soil samples or removed all aerobic microorganisms. The soil samples were kept in the dark at 25 ∘C, maintaining a soil moisture of 60 %. The degradation kinetics of EPM under aerobic, anaerobic, and sterilized conditions are depicted in Fig. 2, while the fitted parameters are summarized in Table 3. The R2 values for EPM in the five soils ranged between 0.9313 and 0.9924, suggesting that the first-order kinetic model agreed with the correspondent degradation data. The half-life of EPM ranged between 37.46 and 58.25 d in the aerobic soils, between 41.75 and 59.74 d in the anaerobic soils, and between 60.87 and 66.00 d in the sterilized soils. A moderate degradation (30 d < t d) of EPM was observed under aerobic, anaerobic, and sterilized conditions. These results can be partly explained by aerobic and anaerobic transformations occurring in the soils, which have been described by the GB 31270.1-2014 guidelines for the testing of chemicals (Gb, 2014c). Overall, the half-life of EPM decreased from the aerobic to the anaerobic and sterilized soils. Understanding the degradation kinetics of herbicides is critical for predicting their persistence in soil and the soil parameters, which affect regional agronomic and environmental practices (Buerge et al., 2019; Buttiglieri et al., 2009). Under dark conditions, the degradation of herbicides in soil mainly results from microbial and abiotic degradation (Marín-Benito et al., 2019). In this study, when EPM was retained under dark conditions for 30 d; its degradation rates in all soils under sterilized conditions (35.44 %, 36.27 %, 33.27 %, 32.80 %, and 34.78 %) were a little slower than under anaerobic (48.60 %, 41.51 %, 35.92 %, 35.61 %, and 38.07 %) and aerobic conditions (53.32 %, 43.20 %, 36.73 %, 35.61 %, and 39.31%; Fig. 2). As the degradation rate increased only by 10 % compared to that observed under sterilized conditions, degradation under aerobic/anaerobic conditions appeared to be mainly abiotic degradation. In contrast, other studies have found that anaerobic microorganisms are predominant contributors in the degradation process and capable of accelerating it. For example, the degradation rates of phenazine-1-carboxamide (PCN) were much higher under anaerobic than aerobic conditions, due to its own structural characteristics (Ou et al., 2020). Between 30 and 120 d, there were no significant differences in the degradation rates of EPM between sterilized and unsterilized soils, suggesting that EPM degradation was largely abiotic in this time interval. This might be attributed to a low bioavailability of EPM for microbial degradation, which is derived from a high adsorption affinity of this compound under the right OM content and pH conditions (Liu et al., 2021b; Q. Wang et al., 2020). Overall, it appears that EPM decomposition in the tested soils was mainly driven by abiotic degradation.
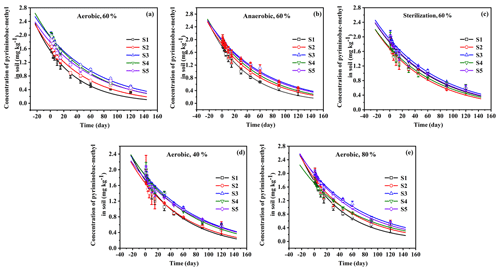
Figure 2Degradation kinetics of EPM under aerobic (a), anaerobic (b), and sterilization (c) conditions with 60 % moisture, under aerobic conditions with 40 % moisture (d), and with 80 % moisture (e) in five different agricultural soils (S1 to S5 are defined in Table 1). Values are the means ± standard error (n=3).
The degradation rate of EPM decreased from S1, S2, S4 to S5 and S3 under both aerobic and anaerobic conditions (Table 3). A negative correlation was noted between the half-life of EPM and the soil OM content and CEC under aerobic conditions (slope <0; P<0.05; R2=0.9478 and 0.8022, respectively); besides, a negative correlation was observed between the half-life of EPM and the soil OM content under aerobic conditions (slope <0, P<0.05; R2=0.8983). Notably, an abundance of OM and high CEC result in an increase in the carbon sources accessible to microorganisms, effectively stimulating their activity (Xu et al., 2020). In the presence of microorganisms, the particularly high OM and CEC, characterizing S1, resulted in the fastest EPM degradation among those observed in all soils under aerobic and anaerobic conditions. However, under sterilized conditions, the degradation rate of EPM decreased from S2, S4, S1, to S5, and S3 (Table 3); moreover, the half-life of EPM and the soil pH exhibited a negative correlation under these same conditions (slope <0; P<0.05; R2=0.8850; Table S6 in the Supplement). The rate of EPM hydrolysis is known to be positively affected by alkaline soil pH. This relationship explains why, in the presence of elevated hydrolysis and under sterilized conditions, the fastest degradation behavior among all the tested soils was observed in S2 (which was characterized by the highest pH). Notably, the highest differences in the degradation rate of EPM were observed under aerobic conditions. In order to comprehensively evaluate the influence of various factors on this degradation rate, we hence focused on the analysis of data collected under aerobic conditions.
The data regarding the degradation behavior of EPM in the tested soils (Table 4 and Fig. 2) conform to first-order kinetics (R2>0.8769). The half-life of EPM varied depending on the moisture conditions. It diminished from soils with a 60 % moisture to those with a moisture level of 80 % and 40 %. Additionally, after 120 d, the degradation rates of EPM in soils with a 40 % moisture level (74.59 %, 73.93 %, 69.98 %, 73.21 %, and 71.25 % for S1–S5, respectively) were obviously lower than those in soils with 80% (77.55 %, 75.38 %, 72.79 %, 75.44 %, and 73.62 % for S1–S5, respectively) and 60% (80.04 %, 77.31 %, 75.43 %, 77.78 %, and 75.77 % for S1–S5, respectively) moisture levels (Table 4 and Fig. 2d, e). These results show that, when the soil moisture increased from 40 % to 60 %, the decay rate of EPM accelerated, possibly due to the stimulation of a degradation pathway (e.g., through aerobic microorganisms and chemical hydrolysis) linked to the increase in soil moisture (Wang et al., 2014; Liu et al., 2021b). Conversely, EPM showed a slower decay when the soil moisture increased from 60 % to 80 %. This phenomenon might have been caused by an increase in sorption, which would have made EPM less bioavailable. This effect was more or less important according to the predominance of different biotic pathways of degradation (Bento et al., 2016; García-Valcárcel and Tadeo, 1999).
3.4 Leaching potential
The corresponding results are shown in Fig. 3. It was found that the fluidity of EPM was lower in S1 than in S2, S3, S4, or S5. Furthermore, the Ri values of this compound in S1, S2, S3, S4, and S5 were R1=99 %, %, R4=71.95 %, %, and %, respectively. Based on the Test Guidelines on Environmental Safety Assessment for Chemical Pesticide – Part 5: Leaching in soil (Gb, 2014b), the mobility of EPM in the soils S1–S5 was categorized as immobile, slightly mobile, highly mobile, slightly mobile, and slightly mobile, respectively. The soil OM content was found to be the most important soil property influencing the mobility of molecular herbicides, followed by the clay content and the CEC. A lower clay content is usually associated with a higher sand content, a higher proportion of large pores, a smaller specific surface area per soil unit volume, and a lower adsorption affinity for herbicides, which, overall, result in a greater herbicide mobility (Boyd et al., 1988; De Matos et al., 2001; Kulshrestha et al., 2004; Temminghoff et al., 1997). We found that a lower soil OM content corresponded to a weaker adsorption affinity, a weaker tendency of EPM to pass from the soil solution to the solid phase, a higher availability of EPM for leaching, and a stronger mobility of this same compound. Notably, the OM content increased from the Ferralsol (S3) to the Plinthosol (S5), Alisol (S4), Anthrosol (S2), and Phaeozems (S1), while the mobility of EPM increased from the Phaeozems (S1) to the Anthrosol (S2), Alisol (S4), Plinthosol (S5), and Ferralsol (S3). This mobility tendency is the opposite compared to the adsorption affinity tendency of EPM in the five soils. As a matter of fact, it is generally known that the mobility of EPM in soil increases as its adsorption affinity decreases. Similar conclusions were reached through the study of other herbicides (Acharya et al., 2020; S. Zhang et al., 2020).
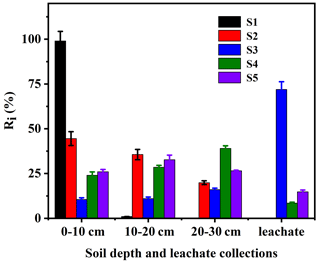
Figure 3Distribution of EPM in soil column and leachate of five different agricultural soils (S1 to S5 are defined in Table 1).
Here, the GUS was also used to estimate both the leaching potential of chemicals and the risk of contaminants into groundwater. The GUS values of EPM in S1, S2, S3, S4, and S5 were 0.9765, 2.0402, 2.7160, 2.3755, and 2.6765, respectively (Table 2). The GUS value in S1 was considerably lower than 1.8, so EPM should have little leaching potential in this soil (Gustafson, 1989; Z. Wang et al., 2020); meanwhile, since the GUS values in the S2, S3, S4, and S5 soils were between 1.8 and 2.8, EPM has a considerable leaching potential there, and, possibly, the ability to pollute groundwater (Huang, 2019; Martins et al., 2018). Overall, we can infer that the risk of groundwater contamination by EPM is low in Phaeozems (S1), due to the low mobility of this compound; however, the risk is much higher when the same compound is contained in Anthrosol (S2), Ferralsol (S3), Alisol (S4), and Plinthosol (S5).
In this study, we found that EPM degrades easily, has a high adsorption affinity and a low mobility in Phaeozems (S1), which results in a low contamination risk for groundwater systems. On the contrary, this compound degrades slowly in Anthrosol (S2), Ferralsol (S3), Alisol (S4), and Plinthosol (S5), due to a low adsorption affinity and moderate mobility, which results in a high contamination risk for groundwater systems. The adsorption–desorption, degradation, and leaching of EPM were systematically explored in five agricultural soils. We noticed that physical adsorption was the main mode of EPM adsorption. The effects of soil physicochemical properties on the adsorption and desorption of this compound were quantified by linear regression analysis. In this regard, the Freundlich adsorption (Kf ads) and desorption (Kf des) constants were linearly and positively correlated with the soil OC content, OM content, and CEC, while nonsignificant correlations were observed among the above constants and the soil pH and clay content.
The dissipation of EPM depended mainly on soil conditions (i.e., moisture, pH, and soil type). EPM degradation was most likely derived from abiotic degradation mechanisms; furthermore, the leaching ability of EPM increased from the Phaeozems (S1) to the Anthrosol (S2), Alisol (S4), Plinthosol (S5), and Ferralsol (S3). Overall, the high leaching ability and desorption capacity of EPM were accompanied by a low adsorption capacity, and there were no significant relationships between pH and the leaching rate of EPM in the five types of soils. In contrast, the OM content, CEC, and soil clay content were mainly responsible for the observed leaching rates.
To completely understand the fate of EPM in the environment, it is necessary to perform additional studies on the microbial community structures and functional diversities of other types of soil besides those analyzed here. As a matter of fact, there are still only a few studies on the environmental fate of EPM; therefore, our results may serve as a reference for evaluating the risks involved in the increasingly wide application of this compound.
The code and data generated in this study are available from the corresponding authors upon reasonable request.
The supplement related to this article is available online at: https://doi.org/10.5194/soil-8-237-2022-supplement.
WZ conceived the idea, designed the study, performed the analyses, and took the lead in writing the paper. HJ and LL assisted with sample extractions and HPLC-MS/MS measurements. MG conducted adsorption and leaching analyses. BL and YL supervised the project. The paper was finalized through contributions of all authors.
The contact author has declared that neither they nor their co-authors have any competing interests.
Publisher’s note: Copernicus Publications remains neutral with regard to jurisdictional claims in published maps and institutional affiliations.
We are grateful to the students Chuanfei Bian, Yue Zhang, Wei Li, and Tianqi Wu, for their assistance during the laboratory work. Last, but not least, we thank the two anonymous reviewers and Canping Pan, for their valuable comments.
This research has been supported by the National Key Research and Development Program of China (grant no. 2017YFD0301604).
This paper was edited by Jerzy Weber and reviewed by two anonymous referees.
Acharya, S. P., Johnson, J., and Weidhaas, J.: Adsorption kinetics of the herbicide safeners, benoxacor and furilazole, to activated carbon and agricultural soils, J. Environ. Sci., 89, 23–34, https://doi.org/10.1016/j.jes.2019.09.022, 2020.
Ahmad, R., Kookana, R. S., Alston, A. M., and Skjemstad, J. O.: The Nature of Soil Organic Matter Affects Sorption of Pesticides. 1. Relationships with Carbon Chemistry as Determined by 13C CPMAS NMR Spectroscopy, Environ. Sci. Technol., 35, 878–884, https://doi.org/10.1021/es001446i, 2001.
Alonso, D. G., Koskinen, W. C., Oliveira, R. S., Constantin, J., and Mislankar, S.: Sorption–Desorption of Indaziflam in Selected Agricultural Soils, J. Agr. Food Chem., 59, 13096–13101, https://doi.org/10.1021/jf203014g, 2011.
Azizian, S., Haerifar, M., and Basiri-Parsa, J.: Extended geometric method: A simple approach to derive adsorption rate constants of Langmuir–Freundlich kinetics, Chemosphere, 68, 2040–2046, https://doi.org/10.1016/j.chemosphere.2007.02.042, 2007.
Bailey, G. W., White, J. L., and Rothberg, T.: Adsorption of Organic Herbicides by Montmorillonite: Role of pH and Chemical Character of Adsorbate, 32, 222–234, Soil Sci. Soc. Am. J., https://doi.org/10.2136/sssaj1968.03615995003200020021x, 1968.
Barchanska, H., Tang, J., Fang, X., Danek, M., Płonka, J., and Sajdak, M.: Profiling and fingerprinting strategies to assess exposure of edible plants to herbicides, Food Chem., 335, 127658, https://doi.org/10.1016/j.foodchem.2020.127658, 2021.
Barriuso, E., Laird, D., Koskinen, W., and Dowdy, R.: Atrazine Desorption From Smectites, Soil Sci. Soc. Am. J., 58, 1632–1638, https://doi.org/10.2136/sssaj1994.03615995005800060008x, 1994.
Bento, C. P. M., Yang, X., Gort, G., Xue, S., van Dam, R., Zomer, P., Mol, H. G. J., Ritsema, C. J., and Geissen, V.: Persistence of glyphosate and aminomethylphosphonic acid in loess soil under different combinations of temperature, soil moisture and light/darkness, Sci. Total Environ., 572, 301–311, https://doi.org/10.1016/j.scitotenv.2016.07.215, 2016.
Boyd, S. A., Lee, J.-F., and Mortland, M. M.: Attenuating organic contaminant mobility by soil modification, Nature, 333, 345–347, https://doi.org/10.1038/333345a0, 1988.
Brillas, E.: Recent development of electrochemical advanced oxidation of herbicides. A review on its application to wastewater treatment and soil remediation, J. Clean. Prod., 290, 125841, https://doi.org/10.1016/j.jclepro.2021.125841, 2021.
Buerge, I. J., Bächli, A., Kasteel, R., Portmann, R., López-Cabeza, R., Schwab, L. F., and Poiger, T.: Behavior of the Chiral Herbicide Imazamox in Soils: pH-Dependent, Enantioselective Degradation, Formation and Degradation of Several Chiral Metabolites, Environ. Sci. Technol., 53, 5725–5732, https://doi.org/10.1021/acs.est.8b07209, 2019.
Bulut, Y. and Aydın, H.: A kinetics and thermodynamics study of methylene blue adsorption on wheat shells, Desalination, 194, 259–267, https://doi.org/10.1016/j.desal.2005.10.032, 2006.
Buttiglieri, G., Peschka, M., Frömel, T., Müller, J., Malpei, F., Seel, P., and Knepper, T. P.: Environmental occurrence and degradation of the herbicide n-chloridazon, Water Res., 43, 2865–2873, https://doi.org/10.1016/j.watres.2009.03.035, 2009.
Calvet, R.: Adsorption of Organic Chemicals in Soils, Environ. Health Persp., 83, 145–177, https://doi.org/10.2307/3430653, 1989.
Carballa, M., Fink, G., Omil, F., Lema, J. M., and Ternes, T.: Determination of the solid–water distribution coefficient (Kd) for pharmaceuticals, estrogens and musk fragrances in digested sludge, Water Res., 42, 287–295, https://doi.org/10.1016/j.watres.2007.07.012, 2008.
Carneiro, G. D. O. P., Souza, M. d. F., Lins, H. A., Chagas, P. S. F. d., Silva, T. S., Teófilo, T. M. d. S., Pavão, Q. S., Grangeiro, L. C., and Silva, D. V.: Herbicide mixtures affect adsorption processes in soils under sugarcane cultivation, Geoderma, 379, 114626, https://doi.org/10.1016/j.geoderma.2020.114626, 2020.
Chefetz, B., Bilkis, Y. I., and Polubesova, T.: Sorption–desorption behavior of triazine and phenylurea herbicides in Kishon river sediments, Water Res., 38, 4383–4394, https://doi.org/10.1016/j.watres.2004.08.023, 2004.
Chen, Y., Han, J., Chen, D., Liu, Z., Zhang, K., and Hu, D.: Persistence, mobility, and leaching risk of flumioxazin in four Chinese soils, J. Soil. Sediment., 21, 1743–1754, https://doi.org/10.1007/s11368-021-02904-3, 2021.
Chianese, S., Fenti, A., Iovino, P., Musmarra, D., and Salvestrini, S.: Sorption of Organic Pollutants by Humic Acids: A Review, Molecules, 25, 918, 2020.
Cueff, S., Alletto, L., Dumény, V., Benoit, P., and Pot, V.: Adsorption and degradation of the herbicide nicosulfuron in a stagnic Luvisol and Vermic Umbrisol cultivated under conventional or conservation agriculture, Environ. Sci. Pollut. R., 28, 15934–15946, https://doi.org/10.1007/s11356-020-11772-2, 2020.
Delle Site, A.: Factors Affecting Sorption of Organic Compounds in Natural Sorbent/Water Systems and Sorption Coefficients for Selected Pollutants. A Review, J. Phys. Chem. Ref. Data, 30, 187–439, https://doi.org/10.1063/1.1347984, 2001.
de Matos, A. T., Fontes, M. P. F., da Costa, L. M., and Martinez, M. A.: Mobility of heavy metals as related to soil chemical and mineralogical characteristics of Brazilian soils, Environ. Pollut., 111, 429–435, https://doi.org/10.1016/S0269-7491(00)00088-9, 2001.
Fan, X., Zou, Y., Geng, N., Liu, J., Hou, J., Li, D., Yang, C., and Li, Y.: Investigation on the adsorption and desorption behaviors of antibiotics by degradable MPs with or without UV ageing process, J. Hazard. Mater., 401, 123363, https://doi.org/10.1016/j.jhazmat.2020.123363, 2021.
FAO: Assessing soil contamination A reference manual, Parameters of pesticides that influence processes in the soil, FOOD AND AGRICULTURE ORGANIZATION OF THE UNITED NATIONS Rome, 75–79, ISBN: 9788170355038, 2000.
Gao, H.-J. and Jiang, X.: Effect of Initial Concentration on Adsorption-Desorption Characteristics and Desorption Hysteresis of Hexachlorobenzene in Soils, Pedosphere, 20, 104–110, https://doi.org/10.1016/S_1002-0160(09)60289-7, 2010.
García-Delgado, C., Marín-Benito, J. M., Sánchez-Martín, M. J., and Rodríguez-Cruz, M. S.: Organic carbon nature determines the capacity of organic amendments to adsorb pesticides in soil, J. Hazard. Mater., 390, 122162, https://doi.org/10.1016/j.jhazmat.2020.122162, 2020.
García-Valcárcel, A. I. and Tadeo, J. L.: Influence of Soil Moisture on Sorption and Degradation of Hexazinone and Simazine in Soil, J. Agr. Food Chem., 47, 3895–3900, https://doi.org/10.1021/jf981326i, 1999.
Gawel, A., Seiwert, B., Sühnholz, S., Schmitt-Jansen, M., and Mackenzie, K.: In-situ treatment of herbicide-contaminated groundwater–Feasibility study for the cases atrazine and bromacil using two novel nanoremediation-type materials, J. Hazard. Mater., 393, 122470, https://doi.org/10.1016/j.jhazmat.2020.122470, 2020.
GB: Test Guidelines of the Environmental Safety Assessment for Chemical Pesticides-Part 4: Adsorption/Desorption in Soils, ISBN: 9787109251830, 2014a.
GB: Test Guidelines on Environmental Safety Assessment for Chemical Pesticides: Part 5: Leaching in soil, ISBN: 9787109251830, 2014b.
GB: Test Guidelines of the Environmental Safety Assessment for Chemical Pesticides-Part 1 (Transformation in Soils), ISBN: 9787109251830, 2014c.
GB: National food safety standard – Maximum residue limits for pesticides in food, ISBN: GB27632021, 2021.
Gee, G. W. and Bauder, J. W. : Partcle-size analysis, in: Methods of soil analysis, part-I, Physical and mineralogical methods, Madison, WI, American Society of Agronomy and Soil Science Society of America, edited by: Klute, A., Soil Science Society of America, https://doi.org/10.2136/sssabookser5.1.2ed.c5, 1986.
Guimares, A., Mendes, K. F., Campion, T. F., Christoffoleti, P. J., and Tornisielo, V. L.: Leaching of Herbicides Commonly Applied to Sugarcane in Five Agricultural Soils, Planta Daninha, 37, e019181505, https://doi.org/10.1590/S0100-83582019370100029, 2019.
Gustafson, D. I.: Groundwater ubiquity score: A simple method for assessing pesticide leachability, Environ. Toxicol. Chem., 8, 339–357, https://doi.org/10.1002/etc.5620080411, 1989.
Hamilton, D. J., Ambrus, Á., Dieterle, R. M., Felsot, A. S., Harris, C. A., Holland, P. T., Katayama, A., Kurihara, N., Linders, J., Unsworth, J., and Wong, S.-S.: Regulatory limits for pesticide residues in water (IUPAC Technical Report), Pure Appl. Chem., 75, 1123–1155, https://doi.org/10.1351/pac200375081123, 2003.
Major, J., Woodford, E., and Sagar, G. R.: Herbicides and the Soil, Am. Midl. Nat., 68, 249, https://doi.org/10.2307/2422653, 1962.
Hochman, D., Dor, M., and Mishael, Y.: Diverse effects of wetting and drying cycles on soil aggregation: Implications on pesticide leaching, Chemosphere, 263, 127910, https://doi.org/10.1016/j.chemosphere.2020.127910, 2021.
Huang, B., Yan, D. D., Wang, X. N., Wang, X. L., Fang, W. S., Zhang, D. Q., Ouyang, C. B., Wang, Qi. X., and Cao, A. C.: Soil fumigation alters adsorption and degradation behavior of pesticides in soil, Environ. Pollut., 246, 264–273, https://doi.org/10.1016/j.envpol.2018.12.003, 2019.
Huang, J. L., Xiu-Ying, L. I., Lin, S. Y., and Guo, X. D.: Determination of glyphosate residues in tea by ion chromatography, J. Food Safe. Food Qual., 7, 1895–1900, https://doi.org/10.19812/j.cnki.jfsq11-5956/ts.2016.05.028, 2016.
Inao, K., Mizutani, H., Yogo, Y., and Ikeda, M.: Improved PADDY model including photoisomerization and metabolic pathways for predicting pesticide behavior in paddy fields: Application to the herbicide pyriminobac-methyl, J. Pestic. Sci., 34, 273–282, https://doi.org/10.1584/jpestics.G09-20, 2009.
Iwafune, T., Inao, K., Horio, T., Iwasaki, N., Yokoyama, A., and Nagai, T.: Behavior of paddy pesticides and major metabolites in the Sakura River, Ibaraki, Japan, J. Pestic. Sci., 35, 114–123, https://doi.org/10.1584/jpestics.G09-49, 2010.
Iwakami, S., Hashimoto, M., Matsushima, K.-i., Watanabe, H., Hamamura, K., and Uchino, A.: Multiple-herbicide resistance in Echinochloa crus-galli var. formosensis, an allohexaploid weed species, in dry-seeded rice, Pestic. Biochem. Phys., 119, 1–8, https://doi.org/10.1016/j.pestbp.2015.02.007, 2015.
Jackson, M.: Soil Chemical Analysis, prentice Hall. Inc, Englewood Cliffs, NJ, https://doi.org/10.1002/jpln.19590850311, 1958.
Jia, C.-S., Zhang, L.-H., Peng, X.-L., Luo, J.-X., Zhao, Y.-L., Liu, J.-Y., Guo, J.-J., and Tang, L.-D.: Prediction of entropy and Gibbs free energy for nitrogen, Chem. Eng. Sci., 202, 70–74, https://doi.org/10.1016/j.ces.2019.03.033, 2019.
Jia, H. R., Zhang, Y., Li, W., and Li, B. T.: HPLC- tandem Mass Spectrometry Method for the Determination of Pyriminobac- methyl 10% WP, Agrochemicals, 58, 106–108, 2019.
Jia, H. R., Zhang, Y., Li, W., Li, B. T., and Zhou, W. W.: Residue dynamics and dietary risk assessment of pyriminobac-methyl in rice, Acta Scientiae Circumstantiae, 4, 1491–1499, 2020.
Jiang, R., Wang, M., Chen, W., and Li, X.: Ecological risk evaluation of combined pollution of herbicide siduron and heavy metals in soils, Sci. Total Environ., 626, 1047–1056, https://doi.org/10.1016/j.scitotenv.2018.01.135, 2018.
Kalsi, N. K. and Kaur, P.: Dissipation of bispyribac sodium in aridisols: Impact of soil type, moisture and temperature, Ecotox. Environ. Safe., 170, 375–382, https://doi.org/10.1016/j.ecoenv.2018.12.005, 2019.
Kaur, P., Kaur, H., Kaur Kalsi, N., and Bhullar, M. S.: Evaluation of leaching potential of penoxsulam and bispyribac sodium in Punjab soils under laboratory conditions, Int. J. Environ. An. Ch., 101, 1–19, https://doi.org/10.1080/03067319.2021.1970148, 2021.
Khorram, M. S., Sarmah, A. K., and Yu, Y.: The Effects of Biochar Properties on Fomesafen Adsorption-Desorption Capacity of Biochar-Amended Soil, Water Air Soil Poll., 229, 60, https://doi.org/10.1007/s11270-017-3603-2, 2018.
Kolakowski, B. M., Miller, L., Murray, A., Leclair, A., and Riet, J. M. V. D.: Analysis of Glyphosate Residues in Foods on the Canadian Retail Market between 2015–2017, J. Agr. Food Chem., 68, 5201–5211, https://doi.org/10.1021/acs.jafc.9b07819, 2020.
Kulshrestha, P., Giese, R. F., and Aga, D. S.: Investigating the Molecular Interactions of Oxytetracycline in Clay and Organic Matter: Insights on Factors Affecting Its Mobility in Soil, Environ. Sci. Technol., 38, 4097–4105, https://doi.org/10.1021/es034856q, 2004.
Lewis, K. A., Tzilivakis, J., Warner, D. J., and Green, A.: An international database for pesticide risk assessments and management, Hum. Ecol. Risk Assess., 22, 1050–1064, https://doi.org/10.1080/10807039.2015.1133242, 2016.
L'Huillier, L., Dupont, S., Dubus, I., Becquer, T., and Bourdon, E.,: Carence et fixation du phosphore dans les sols ferrallitiques ferritiques de Nouvelle-Caledonie, XVIe Congres Mondial de Science du Sol, Montpellier, France, 20–26 August 1998, 20–26, https://www.researchgate.net/publication/50280619 (last access: 10 September 2015), 1998.
Liu, J., Dong, C., Zhai, Z., Tang, L., and Wang, L.: Glyphosate-induced lipid metabolism disorder contributes to hepatotoxicity in juvenile common carp, Environ. Pollut., 269, 116186, https://doi.org/10.1016/j.envpol.2020.116186, 2021a.
Liu, J., Zhou, J. H., Guo, Q. N., Ma, L. Y., and Yang, H.: Physiochemical assessment of environmental behaviors of herbicide atrazine in soils associated with its degradation and bioavailability to weeds, Chemosphere, 262, 127830, https://doi.org/10.1016/j.chemosphere.2020.127830, 2021b.
Luo, F. M., Wu, X. D., and liu, X. Y.: Determination of Pu'er Tea by High Performance Liquid Chromatography Tandem Mass Spectrometry Uncertainty Evaluation of Glyphosate Residues, Analysis and Testing, 22, 144–148, 2019.
Marín-Benito, J. M., Carpio, M. J., Sánchez-Martín, M. J., and Rodríguez-Cruz, M. S.: Previous degradation study of two herbicides to simulate their fate in a sandy loam soil: Effect of the temperature and the organic amendments, Sci. Total Environ., 653, 1301–1310, https://doi.org/10.1016/j.scitotenv.2018.11.015, 2019.
Martins, E. C., de Freitas Melo, V., Bohone, J. B., and Abate, G.: Sorption and desorption of atrazine on soils: The effect of different soil fractions, Geoderma, 322, 131–139, https://doi.org/10.1016/j.geoderma.2018.02.028, 2018.
Martins, J. M. and Mermoud, A.: Sorption and degradation of four nitroaromatic herbicides in mono and multi-solute saturated/unsaturated soil batch systems, J. Contam. Hydrol., 33, 187–210, https://doi.org/10.1016/S0169-7722(98)00070-9, 1998.
Marvin, H. J. P. and Bouzembrak, Y.: A system approach towards prediction of food safety hazards: Impact of climate and agrichemical use on the occurrence of food safety hazards, Agr. Syst., 178, 102760, https://doi.org/10.1016/j.agsy.2019.102760, 2020.
Medo, J., Hricáková, N., Maková, J., Medová, J., Omelka, R., and Javoreková, S.: Effects of sulfonylurea herbicides chlorsulfuron and sulfosulfuron on enzymatic activities and microbial communities in two agricultural soils, Environ. Sci. Pollut. R., 27, 41265–41278, https://doi.org/10.1007/s11356-020-10063-0, 2020.
Mehdizadeh, M., Mushtaq, W., Siddiqui, S. A., Ayadi, S., Kaur, P., Yeboah, S., Mazraedoost, S., Duraid, K. A. A.-T., and Tampubolon, K.: Herbicide Residues in Agroecosystems: Fate, Detection, and Effect on Non-Target Plants, Reviews in Agricultural Science, 9, 157–167, https://doi.org/10.7831/ras.9.0_157, 2021.
Meimaroglou, N. and Mouzakis, C.: Cation Exchange Capacity (CEC), texture, consistency and organic matter in soil assessment for earth construction: The case of earth mortars, Constr. Build. Mater., 221, 27–39, https://doi.org/10.1016/j.conbuildmat.2019.06.036, 2019.
Murphy, E. M. and Zachara, J. M.: The role of sorbed humic substances on the distribution of organic and inorganic contaminants in groundwater, Geoderma, 67, 103–124, https://doi.org/10.1016/0016-7061(94)00055-F, 1995.
Nandi, B. K., Goswami, A., and Purkait, M. K.: Adsorption characteristics of brilliant green dye on kaolin, J. Hazard. Mater., 161, 387–395, https://doi.org/10.1016/j.jhazmat.2008.03.110, 2009.
Nelson, D. and Sommers, L.: Total carbon, organic carbon and organic matter, in: Methods of Soil Analysis, edited by: Page, A., American Society of Agronomy, USA, 539–579, https://doi.org/10.2134/agronmonogr9.2.2ed.c29, 1985.
NY/T: Guidelines for good herbicide application, ISBN: NY/T1997-2011, 2011.
Obregón Alvarez, D., Mendes, K. F., Tosi, M., Fonseca de Souza, L., Campos Cedano, J. C., de Souza Falcão, N. P., Dunfield, K., Tsai, S. M., and Tornisielo, V. L.: Sorption-desorption and biodegradation of sulfometuron-methyl and its effects on the bacterial communities in Amazonian soils amended with aged biochar, Ecotox. Environ. Safe., 207, 111222, https://doi.org/10.1016/j.ecoenv.2020.111222, 2021.
Ou, J., Li, H., Ou, X., Yang, Z., Chen, M., Liu, K., Teng, Y., and Xing, B.: Degradation, adsorption and leaching of phenazine-1-carboxamide in agricultural soils, Ecotox. Environ. Safe., 205, 111374, https://doi.org/10.1016/j.ecoenv.2020.111374, 2020.
Patel, K. F., Tejnecký, V., Ohno, T., Bailey, V. L., Sleighter, R. L., and Hatcher, P. G.: Reactive oxygen species alter chemical composition and adsorptive fractionation of soil-derived organic matter, Geoderma, 384, 114805, https://doi.org/10.1016/j.geoderma.2020.114805, 2021.
Pérez-Lucas, G., Gambín, M., and Navarro, S.: Leaching behaviour appraisal of eight persistent herbicides on a loam soil amended with different composted organic wastes using screening indices, J. Environ. Manage., 273, 111179, https://doi.org/10.1016/j.jenvman.2020.111179, 2020.
Perotti, V. E., Larran, A. S., Palmieri, V. E., Martinatto, A. K., and Permingeat, H. R.: Herbicide resistant weeds: A call to integrate conventional agricultural practices, molecular biology knowledge and new technologies, Plant Sci., 290, 110255, https://doi.org/10.1016/j.plantsci.2019.110255, 2020.
Qin, M., Chai, S., Ma, Y., Gao, H., Zhang, H., and He, Q.: Determination of pyriminobac-methyl and bispyribac-sodium residues in rice by liquid chromatography-tandem mass spectrometry based on QuEChERS, SEPU Chinese Journal of Chromatography, 35, 719–723, https://doi.org/10.3724/sp.J.1123.2017.02032, 2017.
Rae, J. E., Cooper, C. S., Parker, A., and Peters, A.: Pesticide sorption onto aquifer sediments, J. Geochem. Explor., 64, 263–276, https://doi.org/10.1016/S0375-6742(98)00037-5, 1998.
Rao, L., Luo, J., Zhou, W., Zou, Z., Tang, L., and Li, B.: Adsorption–desorption behavior of benzobicyclon hydrolysate in different agricultural soils in China, Ecotox. Environ. Safe., 202, 110915, https://doi.org/10.1016/j.ecoenv.2020.110915, 2020.
Schnitzer, M. and Khan, S. U.: In Humic Substances in the Environment, Soil Sci., 117, 130 p., https://doi.org/10.1097/00010694-197402000-00012, 1972.
Schwarzenbach, R. P. and Westall, J.: Transport of nonpolar organic compounds from surface water to groundwater. Laboratory sorption studies, Environ. Sci. Technol., 15, 1360–1367, https://doi.org/10.1021/es00093a009, 1981.
Shibayama, H.: Weeds and weed management in rice production in Japan, Weed Biol. Manag., 1, 53–60, https://doi.org/10.1046/j.1445-6664.2001.00004.x, 2001.
Silva, T. S., de Freitas Souza, M., Maria da Silva Teófilo, T., Silva dos Santos, M., Formiga Porto, M. A., Martins Souza, C. M., Barbosa dos Santos, J., and Silva, D. V.: Use of neural networks to estimate the sorption and desorption coefficients of herbicides: A case study of diuron, hexazinone, and sulfometuron-methyl in Brazil, Chemosphere, 236, 124333, https://doi.org/10.1016/j.chemosphere.2019.07.064, 2019.
Song, H., Mao, H., and Shi, D.: Synthesis and Herbicidal Activity of α-Hydroxy Phosphonate Derivatives Containing Pyrimidine Moiety, Chin. J. Chem., 28, 2020–2024, https://doi.org/10.1002/cjoc.201090337, 2010.
Spadotto, C. A., Locke, M. A., Bingner, R. L., and Mingoti, R.: Estimating sorption of monovalent acidic herbicides at different pH levels using a single sorption coefficient, Pest Manag. Sci., 76, 2693–2698, https://doi.org/10.1002/ps.5815, 2020.
Stevenson, F. J.: Organic Matter Reactions Involving Herbicides in Soil, J. Environ. Qual., 1, 333–343, https://doi.org/10.2134/jeq1972.00472425000100040001x, 1972.
Sudo, M., Goto, Y., Iwama, K., and Hida, Y.: Herbicide discharge from rice paddy fields by surface runoff and percolation flow: A case study in paddy fields in the Lake Biwa basin, Japan, J. Pestic. Sci., 43, 24–32, https://doi.org/10.1584/jpestics.D17-061, 2018.
Tamaru, M. and Saito, Y.: Studies of the New Herbicide KIH-6127, Part I, Novel Synthesis of Methyl 6-Acetylsalicylate as a Key Synthetic Intermediate for the Preparation of 6-Acetyl Pyrimidin-2-yl Salicylates and Analogues, Pestic. Sci., 47, 125–130, https://doi.org/10.1002/(SICI)1096-9063(199606)47:2<125::AID-PS394>3.0.CO;2-X, 1996.
Tamaru, M., Masuyama, N., Sato, M., Takabe, F., Inoue, J., and Hanai, R.: Studies of the New Herbicide KIH-6127, Part III, Synthesis and Structure–Activity Studies of Analogues of KIH-6127 against Barnyard Grass (Echinochloa oryzicola)∗, Pestic. Sci., 49, 76–84, https://doi.org/10.1002/(SICI)1096-9063(199701)49:1<76::AID-PS491>3.0.CO;2-E, 1997.
Tang, W., Yu, Z.-H., and Shi, D.-Q.: Synthesis, crystal structure, and herbicidal activity of pyrimidinyl benzylamine analogues containing a phosphonyl group, Heteroatom Chem., 21, 148–155, https://doi.org/10.1002/hc.20589, 2010.
Temminghoff, E. J. M., Van der Zee, S. E. A. T. M., and de Haan, F. A. M.: Copper Mobility in a Copper-Contaminated Sandy Soil as Affected by pH and Solid and Dissolved Organic Matter, Environ. Sci. Technol., 31, 1109–1115, https://doi.org/10.1021/es9606236, 1997.
Ternes, T. A., Herrmann, N., Bonerz, M., Knacker, T., Siegrist, H., and Joss, A.: A rapid method to measure the solid–water distribution coefficient (Kd) for pharmaceuticals and musk fragrances in sewage sludge, Water Res., 38, 4075–4084, https://doi.org/10.1016/j.watres.2004.07.015, 2004.
Urach Ferreira, P. H., Ferguson, J. C., Reynolds, D. B., Kruger, G. R., and Irby, J. T.: Droplet size and physicochemical property effects on herbicide efficacy of pre-emergence herbicides in soybean (Glycine max (L.) Merr), Pest. Manag. Sci., 76, 737–746, https://doi.org/10.1002/ps.5573, 2020.
Wang, H. Z., Zuo, H. G., Ding, Y. J., Miao, S. S., Jiang, C., and Yang, H.: Biotic and abiotic degradation of pesticide Dufulin in soils, Environ. Sci. Pollut. R., 21, 4331–4342, https://doi.org/10.1007/s11356-013-2380-8, 2014.
Wang, Q., Fu, Y., Zhang, l., Ling, S., and Wu, Y.: Determination of pyriminobac-methyl isomers in paddy and its storage stability, J. Food Saf. Food Qual., 20, 7429–7435, 2020.
Wang, W., Liang, Y., Yang, J., Tang, G., Zhou, Z., Tang, R., Dong, H., Li, J., and Cao, Y.: Ionic Liquid Forms of Mesotrione with Enhanced Stability and Reduced Leaching Risk, ACS Sustain. Chem. Eng., 7, 16620–16628, https://doi.org/10.1021/acssuschemeng.9b03948, 2019.
Wang, Z., Yang, L., Cheng, P., Yu, Y., Zhang, Z., and Li, H.: Adsorption, degradation and leaching migration characteristics of chlorothalonil in different soils, Eur. J. Remote Sens., 54, 1–10, https://doi.org/10.1080/22797254.2020.1771216, 2020.
Wei, L., Huang, Y., Huang, L., Li, Y., Huang, Q., Xu, G., Müller, K., Wang, H., Ok, Y. S., and Liu, Z.: The ratio of is a useful parameter to predict adsorption of the herbicide metolachlor to biochars, Environ. Res., 184, 109324, https://doi.org/10.1016/j.envres.2020.109324, 2020.
Willett, C. D., Grantz, E. M., Sena, M. G., Lee, J. A., Brye, K. R., and Clarke, J. A.: Soil sorption characteristics of benzobicyclon hydrolysate and estimated leaching risk in soils used for rice production, Environ. Chem., 17, 445–456, https://doi.org/10.1071/EN19189, 2020.
Xiang, L., Wang, X. D., Chen, X. H., Mo, C. H., Li, Y. W., Li, H., Cai, Q. Y., Zhou, D. M., Wong, M. H., and Li, Q. X.: Sorption Mechanism, Kinetics, and Isotherms of Di-n-butyl Phthalate to Different Soil Particle-Size Fractions, J. Agr. Food Chem., 67, 4734–4745, https://doi.org/10.1021/acs.jafc.8b06357, 2019.
Xie, G., Li, B., Tang, L., Rao, L., and Dong, Z.: Adsorption-desorption and leaching behaviors of broflanilide in four texturally different agricultural soils from China, J. Soils Sediments, 21, 724–735, https://doi.org/10.1007/s11368-020-02831-9, 2020.
Xu, Y., Liu, J., Cai, W., Feng, J., Lu, Z., Wang, H., Franks, A. E., Tang, C., He, Y., and Xu, J.: Dynamic processes in conjunction with microbial response to disclose the biochar effect on pentachlorophenol degradation under both aerobic and anaerobic conditions, J. Hazard. Mater., 384, 121503, https://doi.org/10.1016/j.jhazmat.2019.121503, 2020.
Xu, Y., Yu, X., Xu, B., Peng, D., and Guo, X.: Sorption of pharmaceuticals and personal care products on soil and soil components: Influencing factors and mechanisms, Sci. Total Environ., 753, 141891, https://doi.org/10.1016/j.scitotenv.2020.141891, 2021.
Yang, L., Li, H., Zhang, Y., and Jiao, N.: Environmental risk assessment of triazine herbicides in the Bohai Sea and the Yellow Sea and their toxicity to phytoplankton at environmental concentrations, Environ. Int., 133, 105175, https://doi.org/10.1016/j.envint.2019.105175, 2019.
Yang, R., Jia, A., He, S., Hu, Q., Sun, M., Dong, T., Hou, Y., and Zhou, S.: Experimental investigation of water vapor adsorption isotherm on gas-producing Longmaxi shale: Mathematical modeling and implication for water distribution in shale reservoirs, Chem. Eng. J., 406, 125982, https://doi.org/10.1016/j.cej.2020.125982, 2021.
Yin, X. and Zelenay, P.: (Invited)Kinetic Models for the Degradation Mechanisms of PGM-Free ORR Catalysts, ECS Transactions, 85, 1239–1250, https://doi.org/10.1149/08513.1239ecst, 2018.
Yoshii, K., Okada, M., Tsumura, Y., Nakamura, Y., Ishimttsu, S., and Tonogai, Y.: Supercritical Fluid Extraction of Ten Chloracetanilide Pesticides and Pyriminobac-Methyl in Crops: Comparison with the Japanese Bulletin Method, J. AOAC Int., 82, 1239–1245, https://doi.org/10.1093/jaoac/82.5.1239, 2020.
Yue, L., Ge, C., Feng, D., Yu, H., Deng, H., and Fu, B.: Adsorption–desorption behavior of atrazine on agricultural soils in China, J. Environ. Sci., 7, 180–189, 2017.
Yu, Y., Zhuang, Y.-Y., Wang, Z.-H., and Qiu, M.-Q.: Adsorption of water-soluble dyes onto modified resin, Chemosphere, 54, 425–430, https://doi.org/10.1016/S0045-6535(03)00654-4, 2004.
Zhang, C.-L., Qiao, G.-L., Zhao, F., and Wang, Y.: Thermodynamic and kinetic parameters of ciprofloxacin adsorption onto modified coal fly ash from aqueous solution, J. Mol. Liq., 163, 53–56, https://doi.org/10.1016/j.molliq.2011.07.005, 2011.
Zhang, S., Han, B., Sun, Y., and Wang, F.: Microplastics influence the adsorption and desorption characteristics of Cd in an agricultural soil, J. Hazard. Mater., 388, 121775, https://doi.org/10.1016/j.jhazmat.2019.121775, 2020.
Zhang, Y., Li, W., Zhou, W., Jia, H., and Li, B.: Adsorption-desorption characteristics of pyraclonil in eight agricultural soils, J. Soils Sediment., 20, 1404–1412, https://doi.org/10.1007/s11368-019-02471-8, 2020.
Zhou, W., Zhang, Y., Li, W., Jia, H., Huang, H., and Li, B.: Adsorption isotherms, degradation kinetics, and leaching behaviors of cyanogen and hydrogen cyanide in eight texturally different agricultural soils from China, Ecotox. Environ. Safe., 185, 109704, https://doi.org/10.1016/j.ecoenv.2019.109704, 2019.
Zhou, Z., Yan, T., Zhu, Q., Bu, X., Chen, B., Xue, J., and Wu, Y.: Bacterial community structure shifts induced by biochar amendment to karst calcareous soil in southwestern areas of China, J. Soils Sediment., 19, 356–365, https://doi.org/10.1007/s11368-018-2035-y, 2019.