the Creative Commons Attribution 4.0 License.
the Creative Commons Attribution 4.0 License.
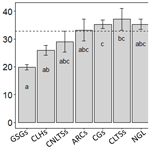
Nitrogen availability determines the long-term impact of land use change on soil carbon stocks in grasslands of southern Ghana
John Kormla Nyameasem
Thorsten Reinsch
Friedhelm Taube
Charles Yaw Fosu Domozoro
Esther Marfo-Ahenkora
Iraj Emadodin
Carsten Stefan Malisch
Enhancing the capacity of agricultural soils to resist soil degradation and to mitigate climate change requires long-term assessments of land use systems. Such long-term evaluations, particularly regarding low-input livestock systems, are limited. In the absence of suitable long-term experiments, this study assessed the outcome of C inputs and outputs across an array of plant functional groups in arable and permanent systems of a tropical savannah after more than 50 years of consistent land use. Soil samples were taken (0–30 cm depth) from arable crop fields, grazed–seeded grassland, cut–use permanent crops and native grassland. Soil organic carbon (SOC) stocks ranged from 17 to 64 Mg SOC ha−1 (mean ± sd = 32.9 ± 10.2 Mg ha−1). SOC stocks were lower for grazed–seeded grassland relative to cut–use grass, legume trees and shrubs. Accordingly, while the conversion of the native grassland to grazed pastures caused an estimated loss of 44 % of SOC over the period, the conversion to woody legumes resulted in slight (5 %), incremental gains. Within sown systems, nitrogen (N) availability seemed to be the most critical factor in determining the fate of the SOC stocks, with the soil N concentration and SOC being highly correlated (r – 0.86; p < 0.001). In total N, P and K were significant predictors of SOC density in the soils. Moreover, secondary plant metabolites in legumes, namely tannins, were identified as having an impact on SOC. The results from this study provide the theoretical basis for testing the hypothesis that improved soil fertility management and the use of tannin-rich plants have the potential to promote long-term SOC storage in the savannah ecological region. Our study also shows the potential of legume tree/shrub forage species as an environmentally sustainable land use option to mitigate agricultural CO2 emissions from low-input livestock systems in the grasslands of southern Ghana.
- Article
(1114 KB) - Full-text XML
-
Supplement
(240 KB) - BibTeX
- EndNote
Increments in increased carbon (C) sequestration rates in soils of 0.4 % per year have been suggested as a means of compensating for the global emissions of greenhouse gases from anthropogenic sources (Chabbi et al., 2017). Grasslands, on a global scale, sequester around 0.14 Mg C ha−1 year−1, thus storing 685 Gt C in the upper soil (to 1 m depth). This C pool size is nearly 50 % more than that of forests (346 Gt C) and 70 % more than wetlands (202 Gt C; Grace et al., 2006; Gobin et al., 2011; Conant et al., 2017). About 60 % of all grasslands occur in the tropics, and these contain 10 %–30 % of the global soil C stocks (Caquet et al., 2012). Besides their potential for mitigating climate change, increased soil C sequestration could enhance ecological efficiency and the delivery of other related ecosystem services, especially food, water and biodiversity (Stringer et al., 2012; Conant et al., 2017). However, large areas of native grasslands worldwide have undergone a substantial use intensification or have been converted into pasture and croplands (Sterling and Ducharne, 2008; Taube et al., 2014). This action has led either to reduced C sequestration rates or net losses of soil organic C (SOC; Johnston et al., 2009; Crews and Rumsey, 2017; Reinsch et al., 2018). This change is also visible in sub-Saharan Africa, where overgrazing and other land uses are factors that affect the C cycle (Grieco et al., 2012) and might constrain the attainment of the 4 ‰ agenda (Minasny et al., 2017) in the subregion.
Conversion of natural to managed ecosystems generally results in depleted soil C stocks, and the conversion of native grasslands to crop production results in an approximately 50 % loss of SOC in global grassland ecosystems (Lal, 2018) as a consequence of destabilizing stored SOC. Adequate organic matter (OM) input into soils is necessary for increased C sequestration. However, sequestered C is sensitive to management and land use changes, and particularly to grazing, and changes in species composition and mineral nutrient availability (Conant et al., 2017). Nevertheless, management practices that increase the supply of quality OM could promote C storage in soils. Accordingly, soil nutrients have implications for plant primary productivity and ecosystem functioning (Post et al., 2012; Marques et al., 2016); however, their exact effects on C sequestration, particularly in grasslands dominated by C4 species, are not well understood (Milne et al., 2016). For example, the impact of N fertilization on SOC dynamics remains controversial because of its dependence on other parameters such soil pH, available phosphorus (P) and potassium (K) and the frequency of tillage management operations (Khan et al., 2007; Lal, 2008; Reinsch et al., 2018).
Both the C input into soils derived from plant residues and organic manures and the carbon's stabilization in the soil are essential for increased soil C sequestration. Some SOC stabilization mechanisms that have been proposed include physico-chemical protection of SOC by micro- and macroaggregates, spatial separation of SOC from decomposers by encapsulation, occlusion and hydrophobicity and mineral–organic associations (Kleber et al., 2015; Song et al., 2018; Quesada et al., 2020). Moreover, plant secondary metabolites in both above- and below-ground biomass have recently been discussed to have an impact on both C and N cycles, with the potential to either increase or reduce C immobilization rates in soils (Kraus et al., 2003; Halvorson et al., 2011; Tamura and Tharayil, 2014; Chomel et al., 2016; Adamczyk et al., 2016, 2017; Kagiya et al., 2019). Condensed tannins (CTs), for example, enter the soil as leachates and via decomposed litter from plant leaves and roots (Hättenschwiler and Vitousek, 2000). Although most forage species naturally contain relatively low levels of CTs, remaining suitable for livestock feeding (Mueller-Harvey et al., 2019), the concentration of CTs may reach potent levels in the long-term because CTs are recalcitrant and could remain in the soil for decades (Tamura and Tharayil, 2014). However, the role of CTs in mitigating CO2 emissions, particularly from tropical soils, has received minimal attention.
In livestock systems, where a large part of the net primary production is exported from the soil as hay or silage or in livestock products, the below-ground biomass is a significant source of C input. Mechanisms driving SOC sequestration in livestock systems, particularly low-input systems in the tropics, are not well understood due to lack of research data and sometimes with conflicting results, even for such important factors as grazing (McSherry and Ritchie, 2013). Previous studies have shown mixed effects of grazing on soil C, including positive (Reeder and Schuman, 2002), neutral (Shrestha and Stahl, 2008) or adverse effects (Pei et al., 2008). Due to the complexity of the different above-described controlling factors on SOC stocks, long-term experiments are ultimately necessary to validate the assumptions that have been made. In the absence of long-term experiments in sub-Saharan Africa that were designed to answer this research question, we selected a research farm that has cultivated and managed plots of different plant species according to the same principles for 50 years. The research farm, located in the southern savannah belt of Ghana, was previously reserved government land. Around 50 years ago, parts of it were simultaneously converted to farmland and research plots. The site offers the unique opportunity to test the long-term effects of converting native grassland to agricultural land using the current SOC and nutrient stocks of the undisturbed grassland, which have not been converted within these 50 years, as a reference baseline. The homogeneous environmental conditions across the entire sampling area would enable estimation of the divergence in SOC and nutrient stocks, due to the different land use changes, when compared to the hypothetical SOC and nutrient stocks represented by the undisturbed grassland. Thus, the main aim of this study was to assess the impact of long-term land use practices on soil C storage and the potential role of tannin-rich forages and soil nutrient status.
In detail, the current case study was conducted to test the following hypotheses:
-
Soil C stocks in sub-Saharan Africa are profoundly affected by the type of land use management.
-
Soil macronutrients and CTs can predict long-term changes in SOC stocks of tropical grasslands.
-
Plant functional groups influence the relationship between SOC and soil N.
2.1 Site characteristics
The study was conducted at a research farm of the Council for Scientific and Industrial Research (CSIR), Ghana, located at 5∘70′ N, 0∘29′ W and 49 m above sea level (a.s.l), some kilometres from Accra, Ghana (Fig. 1). The mean monthly temperature ranges from 21 to 31 ∘C, and the monthly rainfall ranges from 13 to 205 mm (annual rainfall is approximately 800 mm). The major rainy season period is from April to mid-July, with a minor rainy season in October (Fig. 2). Thus, the climate is moist semi-arid, with a growing period lasting 120–180 d (Ghana Meteorological Agency, 2019). The surface lithology is of non-carbonate sedimentary, with coarse sandy loam soils belonging to the Haplic Acrisol group (IUSS, 2015). The soil at the site had the following features at the 0–10 cm soil depth: 80.34 % sand, 12.64 % silt, 7.02 % clay, 5.86 pH (water), 0.133 % N, 2.10 parts per million (ppm) available P, 55 ppm available K and 2.61 % OM (Barnes, 1999). The native land cover of the study location is a tropical grassland savannah.
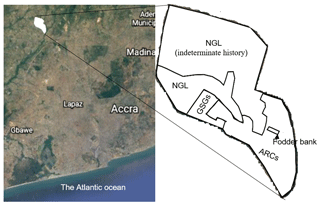
Figure 1Location and plan of the study site. The native grassland (NGL) has remained uncultivated since the farm was established in 1966. The portion of NGL labelled “indeterminate history” was excluded from the study due to high uncertainty associated with its history. The fodder bank consisted of cut–use grasses (CGs), legume herbs (CLHs), legume trees/shrubs (CLTSs) and non-legume trees/shrubs (CNLTSs). ARCs represent arable crop fields. Map from © Google Earth, 2020.
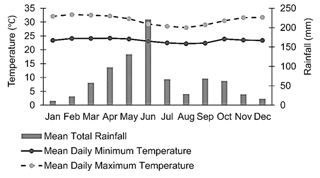
Figure 2Climate (30-year average) of the study area. (Data source: Ghana Meteorological Agency, 2019).
The research farm, located on the grazed–seeded grasslands (GSGs), keeps Sanga cattle, a cross between the humped Zebu-type cattle and the local West African Shorthorn known for their resistance to trypanosomiasis (adult weight range of 300–330 kg), and Djallonké sheep (adult weight range of 25–37 kg) that are managed semi-intensively. These animals are grazed rotationally on seeded pastures during the rainy season periods (April–October) and fed on conserved fodder harvested from arable fields and a fodder bank. While the exact stocking density is not recorded, typical stocking capacity around that region has been reported to be in the range of 3.5–5 LU ha−1 (Timpong-Jones et al., 2013). Compared to that, the native grasslands (NGLs) are infrequently grazed in a nomadic fashion, and no records about their stocking densities are recorded, yet grazing is limited to the dry season when feed is scarce at the GSG plots.
The site was selected due to its suitability for agriculture, its proximity to the capital city and its vegetation and climate, which are representative of the largest grassland type of Ghana (Guinea savannah). Ostensibly, the site had a uniform land usage until parts were converted for different uses in 1966 and in subsequent years (Fig. 1). Briefly, the land uses we encountered comprised arable field crops and grazed–seeded grasslands (both at field scales with at least 1 ha), and a fodder bank that also served as a forage botanical garden housing plant species brought from all over the world. Our study considered 59 species relevant to forage production (Table S1) and represented four plant functional groups: cut–use grasses (38 species), cut–use legume herbs (11 species), cut–use legume trees and shrubs (seven species) and cut–use non-legume trees and shrubs (three species) at plot scales (25–30 m2). As for a subset of species, several accessions of the same species were present, and we sampled 72 plots from the 59 species. The management practices associated with the land use types are summarized in Table 1.
2.2 Soil sampling
Field/plot selection was based on the following two main criteria: (I) that the field was established in 1966 and (II) that the management remained relatively stable over the 50-year time frame. Information regarding the management of fields and biomass productivity of the species was obtained from documentation available at the farm. Plots/fields that underwent some changes in management or were re-established for any reason were omitted from the study. Consequently, there were no replicated plots, as in a traditional agricultural experiment, in the case of the cut–use forage species to control for variation. Hence, we categorized the forage species into functional groups, with the individual species of each functional group constituting a pseudo replicate.
Soil samples were taken from the native grasslands and arable crop fields by zone-based composite sampling, where we divided the fields into three zones (northern, central and southern). For the seeded–grazed paddocks, we randomly selected three paddocks out of the nine for soil sampling. We collected 10–20 subsamples (depending on the size of the zone), spread evenly across each zone in a zigzag pattern, to constitute a composite sample, with each composite soil constituting a replicate. In the case of the plot-scale samples, the sampling procedure followed the recommendations by Saiz and Albrechts (2016). Sampling locations were determined by roughly locating the first at the centre of the field, with three replicates laid out according to a pattern of three axes separated by 120∘ to a primary axis pointing north. Replicates were selected along these axes at an approximate midpoint between the centre of the field and its boundaries.
Before any sampling, surface litter was removed by hand. For each sampling plot or field, soil samples were taken (0–30 cm soil depth) using a soil probe of 1 in. (25.4 mm) diameter. Replicate samples obtained were bulked and thoroughly mixed, and a representative sample was taken in a zipped polythene bag for analyses. Due to the higher number of accessions, the total number of soil samples obtained from the cut–use forages was 72, derived from 59 species. For the estimation of soil bulk density, three to eight sets of soil samples were taken from each experimental unit (replicate plots/fields), depending on size, using a stainless steel core sampler at depths of 0–5, 5–10, 10–15, 15–20 and 20–25 cm. Soil bulk density and C stocks were estimated according to methods by Guo and Gifford (2002).
2.3 Soil analyses
Soil samples meant for C and nutrient analysis were initially oven-dried at 30 ∘C for 48 h. Dried samples were sieved with a 2 mm sieve to remove coarse particles and plant roots. Sieved samples were milled and stored in a desiccator before analyses. Soil samples were analysed for C and total soil N (tN) with the C–N analyser (vario MAX CN; Germany), using aspirin (50 mg; N – 9.7 %; C – 34.0 %) and a standard soil sample (1 g; N – 1.2 %; C – 1.4 %) after every 10 tests of soil samples to aid in the calibration of the equipment. Bulk density was estimated after oven-drying at 105 ∘C. It was assumed that soil samples did not contain inorganic C because pH values were less than 7 and because no liming or any other amendment has been carried out during the past 50 years; therefore, total C was considered as SOC. Additional randomly performed HCl tests confirmed this assumption.
Soil pH was determined according to methods by Wiesmeier et al. (2012). Soil pH was measured directly with a pH meter (microprocessor pH/ION meter; PMX 3000; WTW) after adding 0.0125 M CaCl2 solution to each sample in the ratio of 1:2.5 (soil / CaCl2 solution). Plant available P (aP) and exchangeable K (eK) were extracted from 1 g air-dried fine soil (< 2 mm) using the Bray 2 solution, with the reagents being 0.1 M HCl and 0.03 M NH4F (Bray and Kurtz, 1945). eK was determined using flame photometry, and aP was measured calorimetrically at 882 nm (Miller and Arai, 2016) after a reaction with ammonium molybdate and the development of the molybdenum (Mo) blue colour (within 30 min).
2.4 Plant sampling and proanthocyanidin analyses
Plant samples for CTs determination were harvested from forage species at the time of soil sampling, which was during the late annual growth stage. Sampling included leaves and leaf stalks from dicots, which consisted of legume herbs and legume and non-legume tree and shrub species (Table S1 in the Supplement). After cutting, samples were immediately cooled on ice before being freeze-dried, milled with a ball mill and stored in a freezer at −28 ∘C until further analyses. Condensed tannins (CTs; synonym – proanthocyanidins), which consisted of extractable CTs (ECTs), protein-bound tannins (PCTs) and fibre-bound tannins (FCTs) were determined according to methods prescribed by Terrill et al. (1992). Despite newer analytical techniques being available, Terrill et al. (1992) provide the benefit of separating again between protein- and fibre-bound tannins, and hence, this was considered to be most suitable method. Extractable condensed tannins (ECTs) were extracted from 20 mg plant samples using an acetone and water mixture (80:20, v∕v), vortexed for 5 min and shaken on a plenary shaker (280 min−1) at 4 ∘C overnight. The samples were then centrifuged for 10 min (14 000 rpm) and decanted into 2 mL Eppendorf tubes. Residues were extracted again, using the same set-up with the plenary shaker now shaking for 3 h, and centrifuged, and the supernatant was decanted on top of the first extract. Acetone was evaporated in each case in an Eppendorf concentrator plus (Eppendorf, Hamburg, Germany) at room temperature for 90 min. Extracts were frozen overnight and, after that, freeze-dried for 24 h. A total of 1 mL of ultra-performance liquid chromatography (UPLC)-grade water was added to the freeze-dried extracts, vortexed and filtered with a polytetrafluoroethylene (PTFE) filter (0.2 µm).
Protein-bound condensed tannins (PCTs) were extracted twice from the residues by adding 10 g L−1 sodium dodecyl sulfate (SDS) and 50 g L−1 2-mercaptoethanol in 10 mM Tris–Cl adjusted to a pH of 8, vortexed for 5 min and then placed in a continually boiling water bath for 60 min and cooled on ice to room temperature. The mixture was then centrifuged for 10 min, and the supernatant was decanted into 2 mL Eppendorf tubes in triplicates. For analysis, 960 µL of n-Butanol and HCl (95:5, v∕v) solution was added to 240 µL of the extract of either ECTs or PCTs, vortexed for 5 min and heated in an oven at 90 ∘C for 90 min; thereafter, it was cooled on ice and transferred to a spectrophotometer (Libra S22; Biochrom) and analysed at 550 nm to determine the CT concentrations. For the fibre-bound condensed tannin (FBCT) concentration in the samples, 1200 µL of BuOH∕HCl (95:5, v∕v) and 120 µL SDS was added to the residues from the extracts, vortexed for 5 min, centrifuged for 10 min and heated in an oven for 90 min at 90 ∘C before being cooled on ice to room temperature, centrifuged for 1 min and then measured in the spectrophotometer at 550 nm. Total CT (tCT) was calculated as the sum of ECTs, PCTs and FCTs for each candidate species. Annual tCT was estimated by multiplying tCT with the mean biomass yield of each functional group. The data set and a brief description of the site, materials and methods adopted to generate data are available in Nyameasem et al. (2020).
2.5 Calculations and statistics
Due to lack of data regarding the initial SOC stocks of the farmland or the experimental plot, we estimated percent changes in soil C stocks using a pseudo baseline. This pseudo baseline is the natural grassland, which represents the SOC and nutrient stocks that all other sampled areas would have had they not been converted to farmland and agricultural research plots, respectively. We believe this assumption to be valid as all sampled areas were part of the same natural grassland until being converted, around the same time, to their current land use. With environmental conditions and atmospheric deposition being identical for all sites due to their proximity, the variation among plots is assumed to be a result of the land use change only. Thus, the natural grassland represents the trajectory for soil conditions as they would occur across the entire grassland region without land use change and, thus, represents the baseline against which all sites were tested. To analyse the effect of the different land use types on SOC and soil properties, we performed a one-way ANOVA using generalized linear models. Also, we tested the effects of plant functional groups (legume trees/shrubs, legume herbs and non-legume trees/shrubs) and plant parts (leaves and leaf stalks) on the polyphenol content of the forages in a two-way ANOVA, using linear mixed effect modelling, with species as a random factor. P values were estimated based on the type II sum of squares (SSs) in the case of the one-way ANOVA and type III SSs in the case of the two-way ANOVA due to the dissimilarity of the sample sizes (Fox and Weisberg, 2019). In cases where P values were significant, Tukey's post hoc tests, using the “lsmeans” function of the “multcomp” package (Bretz et al., 2011) were performed to permit pairwise comparisons of the means.
Before the ANOVA, data were checked for normality and the homogeneity of variance. In cases of abnormality or heteroscedasticity, data were log transformed or corrected using “White-adjusted heteroscedasticity-corrected standard errors” from the “car” package of R. Where data normality or the equality of variances were not confirmed even after log transformation, a non-parametric test (Kruskal–Wallis) was used, followed by Dunnett's post hoc tests to permit pairwise comparisons of means of the unequal sample sizes (Zar, 2010). Full correlations, a bivariate (linear and exponential) and a full hierarchical model were fitted to the data sets to establish relationships between the measured variables and SOC and to identify the main effects and interaction terms as predictors of SOC. Akaike's information criterion, with a small sample bias adjustment (AICC; Akaike, 1985; Burnham et al., 2011), was estimated and used to identify the best-fit model. All the statistics were performed using R (R Core Team, 2019).
3.1 Land use change, soil C stocks and soil chemical properties
The different land use types had varying effects on soil C stocks in the upper soil layer (0–30 cm), with group means ranging from 19.9 to 36.8 Mg C ha−1, and with a mean and standard deviation of 32.9 ± 10.2 Mg ha−1. Whereas SOC stocks did not differ between the agricultural soils and native grassland soils (p>0.05), grazed–seeded grassland soils had 86 % and 77 % less (p<0.01) SOC density relative to cut–use trees/shrubs and grasses, respectively (Fig. 3a). Among the cut–use forage systems, SOC was 36 % higher for cut–use grasses relative to legume herbs (p<0.05). Using the native grassland as a pseudo baseline, we observed SOC stock changes ranging from −44 % to 5 %, with a mean loss of −15 %, and the most considerable negative change occurring in grazed–seeded grassland soils (Fig. 3b). However, there appeared to be near-zero to positive changes in cut–use grass and legume trees/shrub soils. Among the cut–use fodder groups, SOC losses in soils of cut–use legume herbs were 26 % greater relative to cut–use grass soils.
The mean (± sd) OM, tN, aP and eK concentrations observed for the site were 1.32 ± 0.41 %, 0.057 ± 0.02 %, 10.4 ± 11.9 mg kg−1 soil and 68.1 ± 36.7 mg kg−1 soil, respectively. Whereas tN, aP and eK concentrations among the native grassland and agricultural soils (p>0.05), cut–use grasses soils contained 37 % more N compared to legume herb soils (Table 2). Arable crop soils contained 5, 7 and 12 times more aP density compared with cut–use grass, herbs and seeded–grazed soils (p<0.001), respectively (Table 2), while eK density in arable crop soils was 5 and 4 times higher (p<0.05) compared with seeded–grazed and legume herb soils, respectively (Table 2). CN ratio was 27 % higher in native grassland soils (p<0.05) compared with seeded–grazed and was lower (p<0.05) for grazed–seeded grasslands compared with non-legume trees/shrubs. It appears that C:N ratio values were not different among the forage species (p>0.05). Soil pH ranged from moderately acidic to neutral and was affected by the land use types, was more acidic in the cut–use herb soils relative to the grasses, arable crop and native grassland soils, and was more acid for seeded–grazed fields relative to arable crop soils (p<0.05).
Table 2Soil organic carbon, soil macronutrients and other chemical properties of soils under different long-term management.
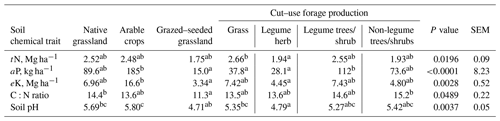
tN – soil total nitrogen; aP – plant-available phosphorus; eK – exchangeable potassium; SEM – standard error of the mean. abc Mean values in the same horizontal row with different letters are significantly different (p<0.05).
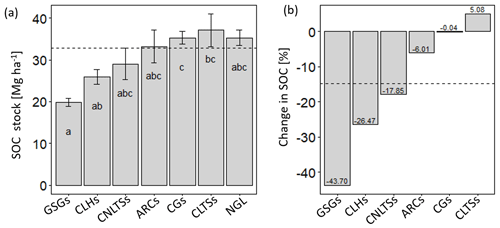
Figure 3Bar plots (mean with error bars – SEM) of SOC stocks (a) and percent changes in SOC stocks (b) due to native grassland conversion under different land use scenarios. Different lowercase letters indicate significant differences between the land use types (p<0.05; se – 1.13). CLTSs – cut–use legume trees/shrubs; NGL – native grassland; CGs – cut–use grasses; ARCs – arable crops; CNLTSs – cut–use non-legume trees/shrubs; CLHs – cut–use legume herbs; GSGs – grazed–seeded grasslands. Broken horizontal line indicates the mean.
3.2 Plant secondary metabolites in cut–use forages
The CT distribution in the forages was affected by plant functional groupings (p<0.001) but not by the part of plant analysed or by plant group–plant part interaction terms (p>0.05). Accordingly, the functional group effect on CT distribution in the forages is presented in Table 3. ECTs, PBCTs and FCTs in the legume herbs were 4, 3 and 2 times, respectively, lower (p<0.001) compared with legume trees/shrubs but not with non-legume trees/shrubs (p>0.05). However, tCT per dry matter (DM) and total annual tCT yield were 2–3 times and 4–6 times, respectively, higher (p<0.001) in both trees/shrubs compared with the herbs (Table 3).
3.3 Relationships between soil organic carbon and soil/plant chemical parameters
Pairwise correlation between the measured variables suggested significant (p<0.01) positive associations between SOC and all the soil chemical properties (Table 4), with the strongest association between SOC and tN (r – 0.86). Although the CT variables correlated positively with SOC and tN, the associations were weak and insignificant, except for annual tCT yield (Table 4). Whereas tN, eK and C:N ratio related exponentially with SOC, aP and soil pH related linearly with SOC (Fig. 4a–e). Generally, higher tN,aP and soil pH appeared to be associated with higher SOC stocks; however, there appears to be a weaker association between SOC and tN at higher soil N concentrations. Whereas the response of SOC to tN was similar for non-legume trees/shrubs, grasses and legume herbs (mean slope – 10.5), it was about 36 % higher compared with legume trees/shrubs (slope – 14); see Fig. 5. Higher soil K density was associated with higher SOC stocks, but amounts beyond 16 Mg ha−1 appeared to depress SOC accumulation, whilst the relationship between SOC and C:N ratio appeared positive only beyond a ratio of 15 (Fig. 4c–d). SOC related exponentially with tCT yield, with generally higher biomass CTs associating positively with SOC at increased rates beyond 200 kg ha−1 (Fig. 6). A full regression model predicting SOC density using the measured soil and plant chemical variables as predictors showed significant effects of tN, aP, eK and tCT and aP × pH and tN × aP × eK interaction terms (p<0.05) in the model, with tN having the greatest effect (Table 5).
Table 3Condensed tannin (CT) profile and CT yield from three plant functional groups growing at the experimental site.
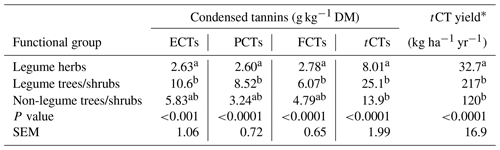
ECTs – extractable condensed tannins; PCTs – protein-bound condensed tannins; FCTs – fibre-bound condensed tannins; tCTs – total condensed tannins (ECT + PCT + FCT). With plant functional groups, values in the same column with different letters are significantly different. *Annual tCT yield was estimated using the mean annual dry matter yields.
Table 4Pearson correlation coefficients showing the relationships between soil and plant chemical parameters.
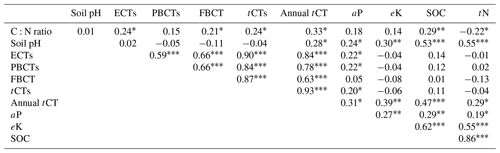
ECTs – extractable condensed tannins; PCTs – protein-bound condensed tannins; FCTs – fibre-bound condensed tannins; tCTs – total condensed tannins (ECT + PCT + FCT); annual tCT – annual tCT yield. Significance levels *<0.05, <0.01 and <0.001.
Table 5Results of full univariate ANOVA model to explain the status of soil organic carbon density at the study site (R2=0.848, adjusted R2=0.853, adjusted R2=0.830 and AICC=698.60*).
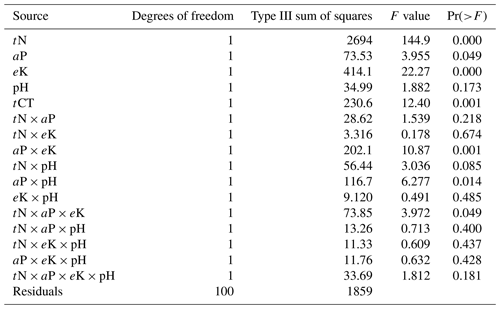
*Akaike's information criterion (AIC; Akaike, 1985; Burnham and Anderson, 1992) with the small sample bias adjustment (AICC [ln (SSE/n)] + 2K + [(2K × (K + 1))∕()]) (Hurvich and Tsai, 1995; Burnham and Anderson, 2002); tN – total N; tCTs – total condensed tannins; aP – available P.
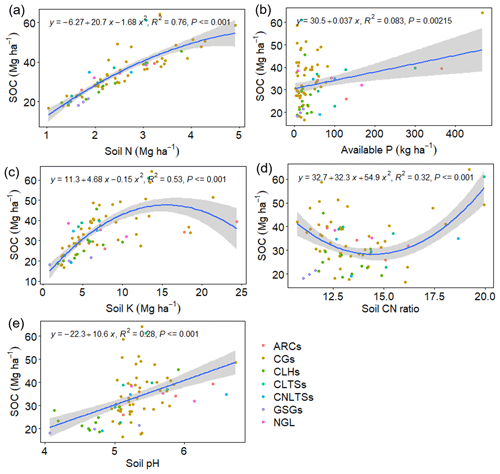
Figure 4Bivariate regression curves showing the relationship between SOC and the measured soil variables. The grey band shows the 95 % confidence interval. CLTSs – cut–use legume trees/shrubs; NGL – native grassland; CGs – cut–use grasses; ARCs – arable crops; CNLTSs – cut–use non-legume trees/shrubs; CLHs – cut–use legume herbs; GSGs – grazed–seeded grasslands.
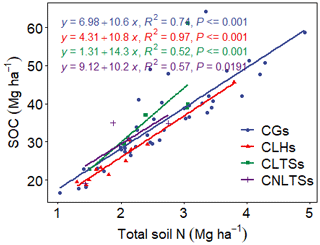
Figure 5Relationships between SOC and soil total N as influenced by plant functional groups. CLTSs – cut–use legume trees/shrubs; CGs – cut–use grasses; CNLTSs – cut–use non-legume trees/shrubs; CLHs – cut–use legume herbs.
4.1 Land use change and carbon stocks
We observed a wide range of SOC stocks (17–64 Mg SOC ha−1) across the land use types at the study site. This is in agreement with previously reported ranges of 10–50 Mg SOC ha−1 for vegetation gradients varying from the Sudanese–Sahelian savannah to a subtropical forest (Saiz et al., 2012; Bessah et al., 2016). Similar to previous reports (Olson, 2013; Bessah et al., 2016), the use of grassland for agriculture resulted in both positive and negative responses by soil C dynamics. Tan et al. (2009) projected changes of −4 % to −23 % in soil C stocks of cultivated savannahs of Ghana on a 100 year scale, depending on the climate change scenarios. Hence, the 15 % loss of SOC in 50 years appears to be on the higher side. Nevertheless, there was an indication of C sequestration in the case of cut–use legume trees/shrub production. Similarly, a previous study (Shanmugam et al., 2018) reported a mean annual change of 0.67 ± 0.95 Mg C ha−1 for tropical mineral soils of secondary woodland or savannah converted to cultivated pasture or cropland. Therefore, our observations are not surprising as the type of land management adopted after converting native vegetation affects soil C cycling and determines the ultimate C storage potential of soils (Amézquita et al., 2008; Stahl et al., 2016). From our study, SOC stocks of arable crop farming did not differ from the native grassland. Arable crop production is known to deplete SOC due to the tillage effect, which tends to facilitate SOC cycling. Among the three arable crop fields considered in this study, only one of them occasionally adopted conventional tillage. On that particular field, however, crop production was on a rotational basis and occurred only occasionally, i.e. tillage occurred only infrequently. On the other crop fields, soils were manually minimum tilled using simple farm implements such as hoes and sometimes herbicidal weed control. Thus, this is, of course, a much less invasive technique compared to regular ploughing (but a representative for many farms in that area), and hence, it appears that the net effect of the mixed management practices on the arable crop farms did not impact SOC stocks significantly.
The low SOC stock in grazed–seeded grasslands was slightly above half (20 vs. 35 t SOC ha−1) that of cut–use grasses and legume trees/shrubs, suggesting a utilization effect. The effect size of grazing on SOC stocks might be dependent on the interactions of several factors, including grazing intensity (McSherry and Ritchie, 2013). Overgrazing has been widely reported as being responsible for the loss of SOC and a decrease in soil fertility in West Africa (Schönbach et al., 2011; Saiz et al., 2012). Generally, higher grazing intensities decrease soil C and N by direct removal of above-ground herbaceous biomass, thus reducing the potential of CO2 fixation in photosynthetic tissue and reducing below-ground C inputs through lower root production and higher root litter turnover (Semmartin et al., 2010). On the other hand, moderate grazing may increase tiller density and above-ground productivity, particularly in C4-dominated grasslands (McSherry and Ritchie, 2013) compared to cut–use systems, thereby leading to higher C inputs because of additions from crop residues and leaf senescence, assuming appropriate supplies of abiotic factors such as irrigation and nutrient supply. Thus, reducing grazing intensity may not only help protect the above-ground biomass of grasslands but it may also lead to improved soil structure and enhanced accumulation of organic C (Xu et al., 2018).
The effect of plant functional differences on SOC stocks was evident in this study. Under similar growing conditions, we observed higher C stocks in fodder grass fields (36 % higher; p<0.01) compared to legume herb fields. Earlier reports (Alonso et al., 2012; Shanmugam et al., 2018) suggested that species composition of grasslands influences the quantity and quality of OM input and of C sequestration in the soil. Dry matter productivity is an essential factor that influences the accumulation of soil C. Above-ground biomass productivity is higher for fodder trees/shrubs relative to that of grasses and lowest in herbaceous legumes (Table 1). SOC stocks under the cut–use forages appear to follow a similar trend (Table 2; Fig. 3). Thus, the higher SOC stocks observed under legume trees/shrubs relative to legume herbs could be partly attributed to the greater ability of legume trees/shrubs to produce relatively high amounts of biomass (Barnes and Addo-Kwarfo, 1996; Adjolohoun et al., 2008) and to maintain soil fertility (as shown in Table 2), control runoff-related soil erosion (Franzel et al., 2014) and maintain SOM.
Grasslands containing more C4 grass species were reported to store more SOC compared to grasslands with more C3 and legume species (Yang et al., 2019). The grass species considered in this study consisted mainly of C4 grasses, whereas the legume herbs were mainly C3 shallow-rooted plants. C4 plants possess morphogenic and architectural traits that enhance their ability to out-perform C3 plants, particularly under harsh environmental conditions (Lattanzi, 2010). They tend to develop a high leaf area index, which enhances their ability to capture light, N and water compared to their C3 counterparts. Besides, C4 plants have higher photosynthetic efficiency in the use of water and N, and they yield higher quantum productivity (Taylor et al., 2010) than C3 plants. The inherently lower Rubisco concentration and the more lignified tissue in C4 plants limit organic matter decomposition, thus producing more recalcitrant organic C (Yang et al., 2019).
Moreover, C4 plants are reported to partition C towards roots in N limited situations; thus, they have a higher ability to fix soil C under N stress situations (Sage and Pearcy, 1987; Long, 1999). Another reason could be that C4 plants have a higher concentration of amino acids and organic acids in their root exudates compared to C3 plants (Nabais et al., 2011), which could protect SOC and N from microbial decomposition. Therefore, the relative differences in biomass characteristics could be responsible for the observed differences in SOC stocks under fodder grass and legume herbs fields.
4.2 Impact of differential land uses and plant types on soil quality
We observed a higher N concentration in the soil of cut–use grass fields compared to legume herb fields, although legumes are known to fix atmospheric N in soils. Indeed, in the semi-arid and savannah ecosystems of sub-Saharan Africa, legume herb and legume browse species are reported to fix 8–217 and 61–643 kg ha−1 yr−1 atmospheric N, respectively (Hassen et al., 2017). Accordingly, legume-based systems can produce high biomass yield even in the absence of N fertilizer application, showing a high N cycling efficiency (Schmeer et al., 2014) and generally increasing soil N concentration. However, several factors may affect N fixation in legumes, including environmental conditions, N uptake by plants and soil pH (Nutman, 1976; Sage and Pearcy, 1987). Pure grass stands may show a high fraction of C and N allocation below-ground (Loges et al., 2018), particularly when the functional traits equilibrium theory of the roots is considered (Brouwer, 1983) and the stands have amino-acid-rich roots (Nabais et al., 2011), leading, potentially, to a more excessive N and C accumulation in the soil. These attributes of grasses might explain why soils in grass plots had higher tN compared to the legume herbs. The fairly tight C:N ratio (14:1) observed for the agricultural systems reflects the stoichiometry of stable soil OM at the study site.
The site of this study has seen a general decline in soil fertility (tN and SOM), although there were marginal increases in available P and K compared with the values reported for the site by Barnes (1999). While P and K concentrations were moderate in arable crop soils, they were low in the other land use types (Bationo et al., 2018; Apal Agricultural Laboratory, 2020). The relatively high P and K values observed in the food crop fields may probably be due to their annual supply through fertilizer application (Table 1). Cut–use grasses and legume herbs appeared to deplete soil P, but this effect was not apparent for legume or non-legume trees and shrubs. This trend might be partly related to differences in the root architecture. The grasses and herbs are shallow rooted and, therefore, exploit nutrients from within the upper soil depth, while the trees/shrubs have roots below the 30 cm soil depth considered in this study and, therefore, might be less able to exploit nutrients extensively from the upper layer of the soil. The relatively low nutrient concentrations observed in the grazed–seeded grasslands matches the low C stocks (Table 2). It is particularly striking that P and K are much lower in grazed grassland despite the greater amounts of excreta deposition associated with grazing. Although we could not ascertain the stocking rate on the grazed–seeded pastures, it appears that there was frequent overgrazing of the pastures, which is a regular feature in this region, exposing the soils to nutrient loss via erosion (Bationo et al., 2018).
The soils were generally acidic, a common characteristic of tropical soils (Jayne et al., 2015). Associated effects of the <5.5 pH observed in grazed–seeded grasslands, cut–use legume herbs and non-legume trees/shrubs soils might cause aluminium and manganese toxicities. Low pH could also cause molybdenum, calcium, magnesium or potassium deficiencies and perhaps reduced microbial activity (Apal Agricultural Laboratory, 2020). Among the land use systems, cut–use legume herbs and seeded–grazed soils appeared more acidic relative to the others. Legume plants commonly form symbiotic associations with rhizobia and accumulate most of their N through symbiotic N fixation. During this process, legume plants take up more cations than anions and release more H+ ions from roots to the soil, leading to low pH values in both the rhizosphere and bulk soil (Zhao et al., 2009; Yang et al., 2016). This effect might differ between legume herbs and legume trees/shrubs due to differences in their root architecture. Grazing fields are associated with high N and C returns from animal excreta, but C and N cycles might cause acidification in grazed fields; for example, nitrate leaching might increase the concentration of H ions, which might lead to decreased soil pH (Ridley et al., 1990).
4.3 Condensed tannin concentration in cut–use forages as affected by functional group differences
The total CT concentration of below 67 g kg−1 DM observed in this study is similar to values reported for tropical forages, with lower concentrations in legume herbs than for browse species (Mupangwa et al., 2000; Sottie et al., 2016). CTs may have both positive and negative effects on livestock, depending on their concentration in the plants. The nutritional implications of CTs are particularly vital in the case of tropical shrubs and tree species, as high CTs are known to impair protein availability (Jayanegara et al., 2019). Previous authors have reported higher values of more than 70 g CT kg−1 DM for some tropical shrubs and trees (Jackson et al., 1996; Rosales, 1999; Pereira et al., 2018), which invariably limits their use as fodder for ruminant livestock.
The proportion of total CTs allocated to ECTs was similar to the range of 12 %–44 % reported by Mupangwa et al. (2000) but lower than the 70 %–95 % reported for some tropical browse species (Jackson et al., 1996). Compared with other tropical forage species, the tCT shares of below 7 % indicate comparably low tannin concentrations, which indicates their suitability as potential feeds for livestock (Mupangwa et al., 2000; Jackson et al., 1996). However, further analyses on forage quality parameters and tannin composition, and on other plant secondary metabolites, might be required to give reliable estimates of their digestibility value.
4.4 Relationships between SOC and soil and plant chemical properties
Our correlation and regression analyses (Fig. 4; Tables 4 and 5) indicated significant relationships between the soil chemical properties and SOC. Similar to reports of Stahl et al. (2016), we found a close association between C and N. Nevertheless, in the literature the relationship between N and SOC is ambiguous, with evidence of different responses of soil CO2 fluxes to N levels in soils (Yan et al., 2016), including increases (Wang et al., 2015), decreases (Jiang et al., 2010) and no significant differences (Li et al., 2012). The N cycle is strongly interconnected with the C cycle as N is required for the cell growth and division and, therefore, for the accumulation of biomass (and hence of C). Whereas Six et al. (2002) attributed the positive relationship between soil C and N to protection by both macro- and microaggregates against mineralization, Waldrop et al. (2004) explained that greater N availability reduces decomposition rate of SOC by regulating the production and activity of microbial extracellular enzymes. Indeed, when N becomes available in N-limited soils, photosynthetic reaction increases, thus enhancing SOC storage, but when amounts of N are low, this may limit the CO2 fertilization effect (Lal, 2018). Accordingly, Tan et al. (2009) observed that increasing N application to about 30–60 kg ha−1 yr−1 could result in positive changes in the soil C stocks of cultivated grasslands of Ghana. In the present study, the multiple linear regression model (Table 5) showed that tN had the most significant effect on SOC density and with a greater effect in size in legume trees/shrub species compared with the legume herbs, non-legume trees/shrubs and grasses (Fig. 5).
Interaction effects involving N, P and K and pH and K were significantly associated with SOC stocks (p<0.05), suggesting that the effect of N on SOC sequestration might be dependent on the availability of P and K and on the soil pH. The associative effect of N and P on SOC density was also reported in an earlier study (Bradford et al., 2008), where P and N additions led to a more significant C sequestration in soils. In a simulated study (Li et al., 2014), a combination of N and P fertilizers increased SOC storage but reduced microbial activity and C mineralization compared to adding N or P fertilizer alone or no fertilization. In contrast, Graham et al. (2014) reported a significant release of CO2 when soil N increased. In the current study, if the relative proportions of soil nutrients impact SOC storage and soils deficient in P are less able to use N efficiently, then this might increase N losses by gaseous emissions and leaching and, thereby, contribute to depletion of SOC pools.
The theoretical basis, according to linear mixed effect regression models (Table 5), suggested tCT concentration in above-ground biomass as being one of the potential factors that might affect SOC storage at the site (Table 5). This observation partly confirms our hypothesis that above-ground CT concentration might be influential in C storage. Although CT concentrations in the soils were not considered in this study, we speculate that the higher CT content in legume trees/shrubs (Table 3) might have slowed the decomposition rate of soil OM (Dong et al., 2016) to promote SOC accumulation (Kraus et al., 2003; Halvorson et al., 2011; Tamura and Tharayil, 2014; Chomel et al., 2016; Adamczyk et al., 2016, 2017; Kagiya et al., 2019). By extension, the higher potential of the trees/shrubs to produce more mass and more CTs per unit area might explain the higher SOC stocks under trees/shrubs relative to those for the herbs. However, the bivariate regression analysis showed that positive associations between tCT and SOC only occurred at concentrations above 200 kg ha−1 yr−1 (45 mg kg−1 DM). Although tropical herbs contained relatively higher CTs than their grass counterparts, the CT concentration might not be potent enough to impact SOC storage, as shown in this study (tCT = 10 ± 8; <45 mg kg−1 DM). Although tropical herbs contained higher amounts of CTs than their grass counterparts, SOC density was lower in soils under the herbs compared with grasses. Even when the CT concentration in the biomass is low, it might be that, in the rhizosphere, it is higher due to accumulation. Below-ground biotic and abiotic conditions, including the presence of some fungi (e.g. Basidiomycetes and Ascomycetes) and bacteria species (e.g. Pseudomonas), temperature, pH, oxygen and substrate availability, are factors that affect the degradation of phenolic compounds in soils (Min et al., 2015). Although the effect of pH on polyphenol degradation is not consistent, under laboratory conditions low soil pH (<5) was shown to be optimal for extracellular enzymes involved in degrading phenolic compounds in soils (Min et al., 2015). In our study, soil pH was lower in soils under the legume herbs compared with the grasses (Table 2). This low soil pH might have facilitated a faster degradation of the potentially accumulated CTs and mitigated the assumed effect of CTs on SOC.
We observed significant positive associations between annual CT yield and all the measured soil parameters. A previous study (Mudau et al., 2007) demonstrated that, regardless of the season, application of N, P and K fertilizers increased the total polyphenols in bush tea (Athrixia phylicoides L.), suggesting that N, P and K limitation might affect the synthesis of CTs by plants and for SOC storage. The low nutrient density in the soils might partly explain the lower concentration of CTs in the forages compared to earlier reports on similar species (Jackson et al., 1996; Rosales, 1999; Pereira et al., 2018). Thus, the proportion of extractable CTs and bound CTs, even within species, might change with plant maturity and environmental conditions (Jackson et al., 1996) and might influence the feeding value of the forage. Consequently, increased soil fertility might have some implications for CT concentration in forages and, thus, for livestock feeding and SOC storage.
We speculate that, at the farm level where climatic and soil properties are relatively uniform, differences in land use might influence the quantity and quality of organic matter added to the soil. These variables might have induced plant–soil feedbacks to regulate soil C cycling at the micro level, causing local variations in C stocks (Post et al., 1982; Saiz et al., 2012; Chen et al., 2018).
4.5 Implications of the study
This study has shown that soil nutrients have implications for SOC input and removal and, by extension, for primary plant productivity and ecosystem functioning (Post et al., 2012; Marques et al., 2016). However, most Ghanaian soils are inherently infertile (Bationo et al., 2018) as result of leaching, soil erosion by rainfall (0.6–0.9 t ha−1) and the fact that nutrients removed by crop harvest are not replaced by the corresponding amount of plant nutrients (Bonsu, 1979; Bationo et al., 2018). Consequently, the low concentration of soil nutrients across the fields may partly explain the low SOC stocks observed for soils in the study site. These fragile soils would require sustainable forms of agricultural land use systems to ensure higher below-ground net primary productivity (NPP) for increased C stocks.
The forage legume herbs showed the lowest yields (Table 1), indicating low bioactivity in comparison with grasses, whereas the legume shrubs had the highest yields and, thus, captured a lot of N from the biomass. In addition, soils under the shrubs contained similar tN compared with grasses, suggesting that most of the tested forage legume herbs were not suitable species with regards to biomass yield for forage and soil carbon sequestration. Legume shrubs should be preferred, as they provide more forage and ecosystem services. Invariably, our findings further extend the benefits of cut–use trees/shrubs as they already contribute to food security, incomes and livelihoods in Africa. These plants are deep rooted, resistant to drought and maintain high protein levels during the dry season when high-quality feed is scarce (Wambugu et al., 2011).
We tested the hypothesis that the conversion of native grasslands can cause considerable losses in soil C stocks of sub-Saharan Africa. Although SOC stocks in the agricultural soils did not differ statistically from the native grassland soils, most of the land transformations resulted in declining SOC stocks, questioning the potential of such farms to achieve the 4 ‰ target. However, the observed wide range of SOC stocks suggests an enormous potential for SOC storage in the area. Our findings further extend the benefits of cut–use trees/shrubs as they already contribute to food security, incomes and livelihoods in Africa. The inclusion of cut–use shrubs/trees or their integration with grass fodder production could be researched further as they show the potential to store more C. All the measured soil chemical properties correlated positively and significantly with SOC, endorsing the fact that soil chemicals are essential drivers of NPP in ecosystems. Hence a boost in productivity is also most likely to result in a boost in SOC. Condensed tannins were positively associated with SOC and could be explored further to harness their potential to extend the residence time of SOC in soils. Inappropriate pasture management, with regards to livestock stocking rate, and choices relating to grazing or forage harvest, species selection and nutrient management could affect the transformation efficiency of plant CTs to soil CTs. We hypothesize that sustainable soil management practices, coupled with the adoption of CT-rich forages, might improve the SOC storage capacity of livestock production systems in this ecological region of Ghana.
All data used in the analysis and the tables and figures are available at https://doi.org/10.6084/m9.figshare.12016158.v1 (Nyameasem et al., 2020).
The supplement related to this article is available online at: https://doi.org/10.5194/soil-6-523-2020-supplement.
JKN acquired the funding to carry out the study. JKN, CSM and TR conceptualized the research experiment and the hypotheses. JKN, CYFD and EMA carried out the data collection, JKN performed the soil and plant analyses, developed the model code and performed the data analysis. JKN, CM, TR and IE prepared the paper, with contributions from all co-authors. FT oversaw the project and took leadership responsibility for the research activity planning and execution.
The authors declare that they have no conflict of interest.
Our thanks go to the management of the Christian Albrechts University (CAU), Kiel, Germany, and the Council for Scientific and Industrial Research (CSIR), Ghana, for allowing access to their facilities during the study. We are also indebted to Rita Kopp at the soil laboratory of the Grassland and Forage Science and Organic Agriculture Department, CAU, and Christof Kruß for their immense technical assistance during the chemical analyses of soil samples and the datasets, respectively.
This research has been supported by the German Academic Exchange Programme (DAAD).
This paper was edited by Nikolaus J. Kuhn and reviewed by two anonymous referees.
Adamczyk, B., Karonen, M., Adamczyk, S., Engström, M. T., Laakso T., Saranpä, P., Kitunen, V., Smolander, A., and Simonc, J.: Tannins can slow-down but also speed-up soil enzymatic activity in a boreal forest, Soil Biol. Biochem., 107, 60–67, https://doi.org/10.1016/j.soilbio.2016.12.027, 2017.
Adamczyk, S., Kitunen, V., Lindroos, A. J., Adamczyk, B., and Smolander, A.: Soil carbon and nitrogen cycling processes and composition of terpenes five years after clear-cutting a Norway spruce stand: Effects of logging residues, For. Ecol. Manag., 381, 318–326, https://doi.org/10.1016/j.foreco.2016.09.034, 2016.
Adjolohoun, S., Bindelle, J., Adandedjan, C., and Buldgen, A.: Some suitable grasses and legumes for ley pastures in Sudanian Africa: the case of the Borgou region in Benin, Biotechnol. Agron. Soc. Environ., 12, 405–419, 2008.
Akaike, H.: Prediction and entropy, in: A Celebration of Statistics: The ISI Centenary Volume, edited by: Atkinson, A. C. and Fienberg, S. E., Springer-Verlag, New York, pp. 1–24, 1985.
Alonso, I., Weston, K., Gregg, R., and Morecroft, M.: Carbon storage by habitat – Review of the evidence of the impacts of management decisions and condition on carbon stores and sources, Natural England Research Reports, NERR043, 2012.
Amézquita, M. C., Amézquita, E., Casasola, F., Ramírez, B. L., Giraldo, H., Gómez, M. E., Llanderal, T., Velásquez, J., and Ibrahim, M.A.: C stocks and sequestration, in: Carbon sequestration in tropical grassland ecosystems, edited by: Mannetje, L.‘t, Amézquita, M. C., Buurman, P., and Ibrahim, M. A., Wageningen Academic Publishers, Wageningen, the Netherlands, pp. 49–67, ISBN 978-90-8686-026-5, 2008.
Apal Agricultural Laboratory: Soil Test Interpretation Guide, available at: https://pdf4pro.com/view/soil-test-interpretation-guide-apal-agricultural-laboratory-514162.html, last access: 2 November 2020.
Barnes, P.: Forage yield and soil improvement potential of some annual and short-term perennial legumes at two sites in Ghana, J. Agr. Sci., 32, 47–51, 1999.
Barnes, P. and Addo-Kwafo, A.: Research note; Evaluation of introduced forage accessions for fodder production at a sub-humid site in southern Ghana, Trop. Grassl., 30, 422–425, 1996.
Bationo, A., Fening, J. O., and Kwaw, A.: Assessment of soil fertility status and integrated soil fertility management in Ghana, in: Improving the profitability, sustainability and efficiency of nutrients through site specific fertilizer recommendations in West Africa agro-ecosystems, edited by: Bationo, A., Ngaradoum, D., Youl, S., Lompo, F., and Fening, J., Springer, Cham, https://doi.org/10.1007/978-3-319-58789-9_7, 2018.
Bessah, E., Bala, A., Agodzo, S. K., and Okhimamhe, A. A.: Dynamics of soil organic carbon stocks in the Guinea Savannah and transition agro-ecology under different land-use systems in Ghana, Cogent Geosci., 2, 1–11, https://doi.org/10.1080/23312041.2016.1140319, 2016.
Bonsu, M.: Soil erosion studies under different cultural practices within the various ecological zones of Ghana, Lecture Notes, FAO Training Course on Soil Conservation and Management, Kwadaso, Kumasi, 1979.
Bradford, M. A., Fierer, N., Jackson, R. B., Maddox, T. R., and Reynolds, J. F.: Nonlinear root-derived carbon sequestration across a gradient of nitrogen and phosphorous deposition in experimental mesocosms, Glob. Change Biol., 14, 1113–1124, https://doi.org/10.1111/j.1365-2486.2008.01564.x, 2008.
Bray, R. H. and Kurtz, L. T.: Determination of total, organic, and available forms of phosphorus in soils, Soil Sci., 59, 39–45, 1945.
Bretz, F., Hothorn, T., and Westfall, P.: Multiple comparisons using R., Chapman and Hall/CRC, New York, https://doi.org/10.1201/9781420010909, 2011.
Brouwer, R.: Functional equilibrium: sense or nonsense?, Neth. J. Agr. Sci., 31, 335–348, 1983.
Burnham, K. P. and Anderson, D. R.: Data-based selection of an appropriate biological model: the key to modern data analysis, in: Wildlife 2001: Populations, edited by: McCullough, D. R. and Barrett, R. H., Elsevier, London, 16–30, 1992.
Burnham, K. P. and White, G. C.: Evaluation of some random effects methodology applicable to bird ringing data, J. Appl. Stat., 29, 245–264, 2002.
Burnham, K. P., Anderson, D. R., and Huyvaert, K. P.: AIC model selection and multimodel inference in behavioural ecology: some background, observations, and comparisons, Behav. Ecol. Sociobiol., 65, 23–35, https://doi.org/10.1007/s00265-010-1029-6, 2011.
Caquet, B., De Grandcourt, A., Thongo M'bou, A., Epron, D., Kinana, A., Saint André, L., and Nouvellon, Y.: Soil carbon balance in a tropical grassland: Estimation of soil respiration and its partitioning using a semi-empirical model, Agr. Forest Meteorol., 158–159, 71–79, https://doi.org/10.1016/j.agrformet.2012.02.008, 2012.
Chabbi, A., Lehmann, J., Ciais, P., Loescher, H. W., Cotrufo, M. F., Don, A., SanClements, M., Schipper, L., Six, J., Smith, P., and Rumpel, C.: Aligning agriculture and climate policy, Nat. Clim. Change, 7, 307–309, https://doi.org/10.1038/nclimate3286, 2017.
Chen, S., Wang, W., Xu, W., Wang, Y., Wan, H., Chen, D., Tang, Z., Tang, X., Zhou, G., Xie, Z., Zhou, D., Shangguan, Z., Huang, J., He, J. S., Wang, Y., Sheng, J., Tang, L., Li, X., Dong, M., Wu, Y., Wang, Q., Wang, Z., Wu, J., Chapin, F. S., and Bai, Y.: Plant diversity enhances productivity and soil carbon storage, P. Natl. Acad. Sci. USA, 115, 4027–4032, https://doi.org/10.1073/pnas.1700298114, 2018.
Chomel, M., Guittonny-Larchevêque, M., Fernandez, C., Gallet, C., DesRochers, A., Paré, D., Jackson, B. G., and Baldy, V.: Plant secondary metabolites: a key driver of litter decomposition and soil nutrient cycling, J. Ecol., 104, 1527–1541, https://doi.org/10.1111/1365-2745.12644, 2016.
Conant, R. T., Cerri, C. E. P., Osborne, B. B., and Paustian K.: Grassland management impacts on soil carbon stocks: A new synthesis, Ecol. Appl., 27, 662–668, https://doi.org/10.1002/eap.1473, 2017.
Crews, T. and Rumsey, B.: What agriculture can learn from native ecosystems in building soil organic matter: A review, Sustainability, 9, 578, https://doi.org/10.3390/su9040578, 2017.
Dong, L., Mao, Z., and Sun, T.: Condensed tannin effects on decomposition of very fine roots among temperate tree species, Soil Biol. Biochem., 103, 489–492. https://doi.org/10.1016/j.soilbio.2016.10.003, 2016.
Fox, J. and Weisberg, S.: An R Companion to Applied Regression, 3rd Edition, Sage, Thousand Oaks CA, 2019.
Franzel, S., Carsan, S., Lukuyu, B., Sinja, J., and Wambugu, C.: Fodder trees for improving livestock productivity and smallholder livelihoods in Africa, Curr. Opin. Env. Sust., 6, 98–103, https://doi.org/10.1016/j.cosust.2013.11.008, 2014.
Ghana Meteorological Agency: Climate data of Pokuase, General Services, Ghana Meteorological Agency, Legon, Accra, available at: https://www.meteo.gov.gh/gmet/, last access: 3 June 2019.
Gobin, A., Campling, P., Janssen, L., Desmet, N., van Delden, H., Hurkens, J., Lavelle, P., and Berman, S.: Soil organic matter management across the EU – best practices, constraints and trade-offs, Final Report for the European Commission's DG Environment, https://doi.org/10.2779/17252, 2011.
Grace, J., José, J. S., Meir, P., Miranda, H. S., and Montes, R. A.: Productivity and carbon fluxes of tropical savannas, J. Biogeogr., 33, 387–400, https://doi.org/10.1111/j.1365-2699.2005.01448.x, 2006.
Graham, S. L., Hunt, J. E., Millard, P., McSeveny, T., Tylianakis, J. M., and Whitehead, D.: Effects of soil warming and nitrogen addition on soil respiration in a New Zealand tussock grassland, PLoS One, 9, e91204, https://doi.org/10.1371/journal.pone.0091204.t001, 2014.
Grieco, E., Chiti, T., and Valentini, R.: Land use change and carbon stocks dynamics in sub-Saharan Africa – a Case study of Western Africa – Ghana, EGU General Assembly, Vienna, Austria, 22–27 April 2012, 12218, 2012.
Guo, L. B. and Gifford, R. M.: Soil carbon stocks and land-use change: a meta-analysis, Glob. Change Biol., 8, 345–360, https://doi.org/10.1046/j.1354-1013.2002.00486.x, 2002.
Halvorson, J. J., Gonzalez, J. M., and Hagerman, A. E.: Repeated applications of tannins and related phenolic compounds are retained by the soil and affect cation exchange capacity, Soil Biol. Biochem., 43, 1139–1147, https://doi.org/10.1016/j.soilbio.2011.01.023, 2011.
Hassen, A., Talore, D. G., Tesfamariam, E. H., Friend, M. A., and Mpanza, T. D. E.: Potential use of forage-legume intercropping technologies to adapt to climate change impacts on mixed crop-livestock systems in Africa: a review, Reg. Environ. Change, 17, 1713–1724, https://doi.org/10.1007/s10113-017-1131-7, 2017.
Hättenschwiler, S. and Vitousek, P. M.: The role of polyphenols in terrestrial ecosystem nutrient cycling, Trends Ecol. Evol., 15, 238–242, https://doi.org/10.1016/S0169-5347(00)01861-9, 2000.
Hurvich, C. M. and Tsai, C.-L.: Model selection for extended quasi-likelihood models in small samples, Biometrics, 51, 1077–1084, 1995.
International Union of Soil Science (IUSS) Working Group: World Reference Base for Soil Resources 2014, International soil classification system for naming soils and creating legends for soil maps, World Soil Resources Reports No. 106, FAO, Rome, ISBN 978-92-5-108369-7, 2015
Jackson, F. S., Barry, T. N., Lascano, C., and Palmer, B.: The extractable and bound condensed tannin content of leaves from tropical tree, J. Sci. Food Agr., 71, 103–110, https://doi.org/10.1002/(SICI)1097-0010(199605)71:1<103::AID-JSFA554>3.0.CO;2-8, 1996.
Jayanegara, A., Sujarnoko, T. U. P., Ridla, M., Kondo, M., and Kreuzer, M.: Silage quality as influenced by concentration and type of tannins present in the material ensiled: A meta-analysis, J. Anim. Physiol. An. N., 103, 456–465, https://doi.org/10.1111/jpn.13050, 2019.
Jayne, T., Kolavalli, S., Debrah, K., Ariga, J., Brunache, P., Kabaghe, C., Nunez-Rodriguez, W., Owusu Baah, K., Bationo, A. A., Jeroen Huising, E., Lambrecht, I., Diao, X., Yeboah, F., Benin, S., and Andam, K.: Towards a sustainable soil fertility strategy in Ghana, Report submitted to the Ministry of Food and Agriculture Government of Ghana, Feed the Future Innovation Lab for 85 Food Security Policy Research Papers 258733, Michigan State University, Department of Agricultural, Food, and Resource Economics, Feed the Future Innovation Lab for Food Security (FSP), https://doi.org/10.22004/ag.econ.258733, 2015.
Jiang, C., Yu, G., Fang, H., Cao, G., and Li, Y.: Short-term effect of increasing nitrogen deposition on CO2, CH4 and N2O fluxes in an alpine meadow on the Qinghai-Tibetan Plateau, China, Atmos. Environ., 44, 2920–2926, https://doi.org/10.1016/j.atmosenv.2010.03.030, 2010.
Johnston, A. E., Poulton, P. R., and Coleman, K.: Soil organic matter: its importance in sustainable agriculture and carbon dioxide fluxes, Adv. Agron., 101, 1–57, https://doi.org/10.1016/S0065-2113(08)00801-8, 2009.
Kagiya, N., Reinsch, T., Taube, F., Salminen, J.-P., Kluß, C., Hasler, M., and Malisch, C. S.: Turnover rates of roots vary considerably across temperate forage species, Soil Biol. Biochem., 139, 107614, https://doi.org/10.1016/j.soilbio.2019.107614, 2019.
Khan, S. A., Mulvaney, R. L., Ellsworth, T. R., and Boast, C. W.: The myth of nitrogen fertilization for soil carbon sequestration, J. Environ. Qual., 36, 1821–1832, https://doi.org/10.2134/jeq2007.0099, 2007.
Kleber, M., Eusterhues, K., Keiluweit, M., Mikutta, C., Mikutta, R., and Nico, P. S.: Mineral-organic associations: formation, properties, and relevance in soil environments, Adv. Agron., 130, 1–114, https://doi.org/10.1016/bs.agron.2014.10.005, 2015.
Kraus, T. E. C., Dahlgren, R. A., and Zasoski, R. J.: Tannins in nutrient dynamics of forest ecosystems – A review, Plant Soil, 256, 41–66, https://doi.org/10.1023/A:1026206511084, 2003.
Lal, R.: Sequestration of atmospheric CO2 into global carbon pool, Energ. Environ. Sci., 1, 86–100, https://doi.org/10.1039/B809492F, 2008.
Lal, R.: Digging deeper: a holistic perspective of factors affecting soil organic carbon sequestration in agroecosystems, Glob. Change Biol. 24, 3285–3301, https://doi.org/10.1111/gcb.14054, 2018.
Lattanzi F. A.: C3/C4 grasslands and climate change, Grassland Sci. Eur., 15, 3–13, 2010.
Li, J. H., Yang, Y. J., Li, B. W., Li, W. J., Wang, G., and Knops J. M. H.: Effects of nitrogen and phosphorus fertilization on soil carbon fractions in alpine meadows on the Qinghai-Tibetan Plateau, PLoS ONE, 9, e103266, https://doi.org/10.1371/journal.pone.0103266, 2014.
Li, K., Gong, Y., Song, W., He, G., Hu, Y., Tian, C., and Liu, X.: Responses of CH4, CO2 and N2O fluxes to increasing nitrogen deposition in alpine grassland of the Tianshan Mountains, Chemosphere, 88, 140–143, https://doi.org/10.1016/j.chemosphere.2012.02.077, 2012.
Loges, R., Bunne, I., Reinsch, T., Malisch, C., Kluß, C., Herrmann, A., and Taube, F.: Forage production in rotational systems generates similar yields compared to maize monocultures but improves soil carbon stocks, Eur. J. Agron., 97, 11–19, https://doi.org/10.1016/j.eja.2018.04.010, 2018.
Long, S. P.: Environmental responses, in: C4 Plant Biology, edited by: Sage, R. F. and Monson, R. K., Academic Press, San Diego, USA, pp. 215–249, 1999.
Marques, J., Luizao, F., Teixeira, W., Vitel, C., and Marques, E.: Soil organic carbon, carbon stock and their relationships to physical attributes under forest soils in central Amazonia, Rev. Arvore, 40, 197–208, https://doi.org/10.1590/0100-67622016000200002, 2016.
McSherry, M. E. and Ritchie, M. E.: Effects of grazing on grassland soil carbon: A global review, Glob. Change Biol., 19, 1347–1357, https://doi.org/10.1111/gcb.12144, 2013.
Miller, A. P. and Arai, Y.: Comparative evaluation of phosphate spectrophotometric methods in soil test phosphorus extracting solutions, Soil Sci. Soc. Am. J., 80, 1543–1550, https://doi.org/10.2136/sssaj2016.08.0256n, 2016.
Milne, E., Aynekulu, E., Bationo, A., Batjes, N. H., Boone, R., Conant, R. Davies, J., Hanan, N., Hoag, D., Herrick, J. E., Knausenberger, W., Neely, C., Njoka, J., Ngugi, M., Parton, B., Paustian, K., Reid, R., Said, M., Shepherd, K., Swift, D., Thornton, P., Williams, S., Miller, S., and Nkonya, E.: Grazing lands in Sub-Saharan Africa and their potential role in climate change mitigation: What we do and don't know, Environ. Dev., 19, 70–74, https://doi.org/10.1016/j.envdev.2016.06.001, 2016.
Mudau, F. N., Soundy, P., and Du Toit, E. S.: Effects of nitrogen, phosphorus, and potassium nutrition on total polyphenol content of bush tea (Athrixia phylicoides L.) leaves in shaded nursery environment, HortScience, 42, 334–338, https://doi.org/10.21273/HORTSCI.42.2.334, 2007.
Mueller-Harvey, I., Bee, G., Dohme-Meier, F., Hoste, H., Karonen, M., Kölliker, R., Lüscher, A., Niderkorn, V., Pellikaan, W. F., Salminen, J.-P., Skøt, L., Smith, L. M. J., Thamsborg, S. M., Totterdell, P., Wilkinson, I., Williams, A. R., Azuhnwi, B. N., Baert, N., Brinkhaus, A. G., Copani, G., Desrues, O., Drake, C., Engström, M., Fryganas, C., Girard, M., Huyen, N. T., Kempf, K., Malisch, C., Mora-Ortiz, M., Quijada, J., Ramsay, A., Ropiak, H. M., and Waghorn, G. C.: Benefits of condensed tannins in forage legumes fed to ruminants: Importance of structure, concentration, and diet composition, Crop Sci., 59, 861–885, https://doi.org/10.2135/cropsci2017.06.0369, 2019.
Min, K., Freeman, C., Kang, H., and Choi, S. U.: The regulation by phenolic compounds of soil organic matter dynamics under a changing environment, Biomed. Res. Int., 2015, 825098, https://doi.org/10.1155/2015/825098, 2015.
Minasny, B., Malone, B. P., McBratney, A. B., Angers, D. A., Arrouays, D., Chambers, A., Chaplot, V., Chen, Z.-S., Cheng, K., Das, B. S., Field, D. J., Gimona, A., Hedley, C. B., Hong, S. Y., Mandal, B., Marchant, B. P., Martin, M., McConkey, B. G., Mulder, V. L., O'Rourke, S., Richer-de-Forges, A. C., Odeh, I., Padarian, J., Paustian, K., Pan, G., Poggio., L., Savin, I., Stolbovoy, V., Stockmann, U., Sulaeman, Y., Tsui, C.-C., Vågen, T.-G., van Wesemael, B., and Winowiecki, L.: Soil carbon 4 per mille, Geoderma, 292, 59–86, https://doi.org/10.1016/j.geoderma.2017.01.002, 2017.
Mupangwa, J. F., Acamovic, T., Topps, J. H., Ngongoni, N. T., and Hamudikuwanda, H.: Content of soluble and bound condensed tannins of three tropical herbaceous forage legumes, Anim. Feed Sci. Tech., 83, 139–144, https://doi.org/10.1016/S0377-8401(99)00117-0, 2000.
Nabais, C., Labuto, G., Gonçalves, S., Buscardo, E., Semensatto, D., Nogueira, A. R., and Freitas, H.: Effect of root age on the allocation of metals, amino acids and sugars in different cell fractions of the perennial grass Paspalum notatum (Bahiagrass), Plant Physiol. Bioch., 49, 1442–1447, https://doi.org/10.1016/j.plaphy.2011.09.010, 2011.
Nutman, P. S. (Ed.): IBP field experiments on nitrogen fixation by nodulated legumes, in: Symbiotic nitrogen fixation by plants, Cambridge Univ. Press, London, 211–238, 1976.
Nyameasem, J. K., Reinsch, T., Taube, F., Domozoro, C. Y. F., Ahenkora-Marfo, E., Emadodin, I., and Malisch, C. S.: Nitrogen availability determines the long-term impact of land-use change on soil carbon stocks in Ghana, Figshare, https://doi.org/10.6084/m9.figshare.12016158.v1, last access: 2 November 2020.
Olson, K. R.: Soil organic carbon sequestration, storage, retention and loss in US croplands: Issues paper for protocol development, Geoderma, 195–206, https://doi.org/10.1016/j.geoderma.2012.12.004, 2013.
Pei, S. F., Fu, H., and Wan, C. G.: Changes in soil properties and vegetation following exclosure and grazing in degraded Alxa desert steppe of Inner Mongolia, China, Agr. Ecosyst. Environ., 124, 33–39, https://doi.org/10.1016/j.agee.2007.08.008, 2008.
Pereira, T. P., Modesto, E. C., Nepomuceno, D. de D., de Oliveira, O. F., de Freitas, R. S. X., Muir, J. P., Dubeux Jr., J. C. B., and de Carvalho Almeida, J. C.: Characterization and biological activity of condensed tannins from tropical forage legumes, Pesqui. Agropecu. Bras., 53, 1070–1077, https://doi.org/10.1590/S0100-204X2018000900011, 2018.
Post, W., Emanuel, W. R., Zinke, P. J., and Stangenberger, A. G.: Soil carbon pools and world life zones, Nature, 298, 156–159, https://doi.org/10.1038/298156a0, 1982.
Post, W., Izaurralde, R., West, T., Liebig, M., and King, A.: Management opportunities for enhancing terrestrial carbon dioxide sinks, Front Ecol. Environ., 10, 554–561, https://doi.org/10.1890/120065, 2012.
Quesada, C. A., Paz, C., Oblitas Mendoza, E., Phillips, O. L., Saiz, G., and Lloyd, J.: Variations in soil chemical and physical properties explain basin-wide Amazon forest soil carbon concentrations, SOIL, 6, 53–88, https://doi.org/10.5194/soil-6-53-2020, 2020.
R Core Team: R: a language and environment for statistical computing, R Foundation for Statistical Computing, Vienna, Austria, https://www.R-project.org/ (last access: 2 November 2020), 2019.
Reeder, J. D. and Schuman, G. E.: Influence of livestock grazing on C sequestration in semi-arid mixed-grass and short-grass rangelands, Environ. Pollut., 116, 457–463, https://doi.org/10.1016/s0269-7491(01)00223-8, 2002.
Reinsch, T., Loges, R., Kluß, C., and Taube, F.: Effect of grassland ploughing and reseeding on CO2 emissions and soil carbon stocks, Agr. Ecosyst. Environ., 265, 374–383, https://doi.org/10.1016/j.agee.2018.06.020, 2018.
Ridley, A. M., Slattery, W. J., Helyar, K. R., and Cowling, A.: Acidification under grazed annual and perennial grass based pastures. Australian Journal of Experimental Agriculture, 30, 539–544, https://doi.org/10.1071/EA9900539, 1990.
Rosales, R. B.: Condensed tannins in tropical forage legumes: their characterization and study of their nutritional impact from the standpoint of structure-activity relationships, PhD Thesis, The University of Reading, 1999.
Sage, R. F. and Pearcy, R. W.: The nitrogen use efficiency of C3 and C4 plants, II. Leaf nitrogen effects on the gas exchange characteristics of Chenopodium album (L.) and Amaranthus retroflexus (L.), Plant Physiol., 84, 959–963, https://doi.org/10.1104/pp.84.3.959, 1987.
Saiz, G. and Albrecht, A.: Methods for smallholder quantification of soil carbon stocks and stock changes, in: Methods for measuring greenhouse gas balances and evaluating mitigation options in smallholder agriculture, edited by: Rosenstock, T., Rufino, M., Butterbach-Bahl, K., Wollenberg, L., and Richards, M., Springer, Cham, https://doi.org/10.1007/978-3-319-29794-1, 2016.
Saiz, G., Bird, M. I., Domingues, T., Schrodt, F., Schwarz, M., Feldpausch, T. R., Veenendaal, E., Djagbletey, G., Hien, F., Compaore, H., Diallo, A., and Lloyd, J.: Variation in soil carbon stocks and their determinants across a precipitation gradient in West Africa, Glob. Change Biol., 18, 1670–1683, https://doi.org/10.1111/j.1365-2486.2012.02657.x, 2012.
Schmeer, M., Loges, R., Dittert, K., Senbayram, M., Horn, R., and Taube, F.: Legume-based forage production systems reduce nitrous oxide emissions, Soil Till. Res., 143, 17–25, https://doi.org/10.1016/j.still.2014.05.001, 2014.
Schönbach, P., Wan, H., Gierus, M., Bai, Y., Müller, K., Lin, L., Susenbeth, A., and Taube, F.: Grassland responses to grazing: effects of grazing intensity and management system in an Inner Mongolian steppe ecosystem, Plant Soil, 340, 103–115, https://doi.org/10.1007/s11104-010-0366-6, 2011.
Semmartin, M., Di Bella, C., and de Salamone, I. G.: Grazing-induced changes in plant species composition affect plant and soil properties of grassland mesocosms, Plant Soil, 328, 471–481, https://doi.org/10.1007/s11104-009-0126-7, 2010.
Shanmugam, S., Dalal, R., Joosten, H., Raison, R., and Joo, G.: SOC stock changes and greenhouse gas emissions following tropical land use conversions to plantation crops on mineral soils, with a special focus on oil palm and rubber plantations, Agriculture, 8, 133, https://doi.org/10.3390/agriculture8090133, 2018.
Shrestha, G. and Stahl, P. D.: Carbon accumulation and storage in semi-arid sagebrush steppe: effects of long-term grazing exclusion, Agr. Ecosyst. Environ., 125, 173–181, https://doi.org/10.1016/j.agee.2007.12.007, 2008.
Six, J., Feller, C., Denef, K., Ogle, S., de Moraes Sa, J. C., and Albrecht, A.: Soil organic matter, biota and aggregation in temperate and tropical soils – Effects of no-tillage, Agronomie, 22, 755–775, https://doi.org/10.1051/agro:2002043, 2002.
Song, Z., Liu, C., Müller, K., Yang, X., Wu, Y., and Wang, H.: Silicon regulation of soil organic carbon stabilization and its potential to mitigate climate change, Earth-Sci. Rev., 185, 463–475, https://doi.org/10.1016/j.earscirev.2018.06.020, 2018.
Sottie, E., Marfo-Ahenkora, E., Domozoro, C., Wallace, P., and Iwaasa, A.: Nutritive value and condensed tannin concentration of some tropical legumes, in: Proceedings of the 10th international rangeland congress, edited by: Iwaasa, A., Lardner, H. A., Schellenberg, M., Willms, W., and Larson, K., Saskatoon, 16–22 July 2016, available at: http://2016canada.rangelandcongress.org (last access: 2 November 2020), 2016.
Stahl, C., Fontaine, S., Klumpp, K., Picon-Cochard, C., Grise, M. M., Dezécache, C., Ponchant, L., Freycon, V., Blanc, L., Bonal, D., Burban, B., Soussana, J.-F., and Blanfort, V.: Continuous soil carbon storage of old permanent pastures in Amazonia, Glob. Change Biol., 23, 3382–3392, https://doi.org/10.1111/gcb.13573, 2016.
Sterling, S. and Ducharne, A.: Comprehensive dataset of global land-cover change for land surface model applications, Global Biogeochem. Cy., 22, GB3017, https://doi.org/10.1029/2007gb002959, 2008.
Stringer, L. C., Dougill, A. J., Thomas, A. D., Spracklen, D. V., Chesterman, S., Speranza, C. I., Rueff, H., Riddell, M., Williams, M., Beedy, T., Abson, D. J., Klintenberg, P., Syampungani, S., Powell, P., Palmer, A. R., Seely, M. K., Mkwambisi, D. D., Falcao, M., Sitoe, A., Ross, S., and Kopolo, G.: Challenges and opportunities in linking carbon sequestration, livelihoods and ecosystem service provision in drylands, Environ. Sci. Policy, 19–20, 121–135, https://doi.org/10.1016/j.envsci.2012.02.004, 2012.
Tamura, M. and Tharayil, N.: Plant litter chemistry and microbial priming regulate the accrual, composition and stability of soil carbon in invaded ecosystems, New Phytol., 203, 110–124, https://doi.org/10.1111/nph.12795, 2014.
Tan, Z., Tieszen, L. L., Tachie-Obeng, E., Liu, S., and Dieye, A. M.: Historical and simulated ecosystem carbon dynamics in Ghana: land use, management, and climate, Biogeosciences, 6, 45–58, https://doi.org/10.5194/bg-6-45-2009, 2009.
Taube, F., Gierus, M., Hermann, A., Loges, R., and Schönbach, P.: Grassland and globalization – challenges for north-west European grass and forage research, Grass Forage Sci., 69, 2–16, https://doi.org/10.1111/gfs.12043, 2014.
Taylor, S. H., Hulme, S. P., Rees, M., Ripley, B. S., Woodward, F. I., and Osborne, C. P.: Ecophysiological traits in C3 and C4 grasses: a phylogenetically controlled screening experiment, New Phytol., 185, 780–791, https://doi.org/10.1111/j.1469-8137.2009.03102.x, 2010.
Terrill, T. H., Rowan, T. H., Douglas, G. B., and Barry, T. N.: Determination of extractable and bound condensed tannin concentrations in forage plants, protein concentrate meals and cereal grains, J. Sci. Food Agr., 58, 321–329, https://doi.org/10.1002/jsfa.2740580306, 1992.
Timpong-Jones, E. C., Mutanga, O., Adogbla-Bessa, T., Attua, E. M., and Adiku, S. G. K.: Herbage yield and grazing capacity estimation in a tropical coastal Savannah rangeland using spatial statistics, West Afr. J. Appl. Ecol., 21, 37–45, 2013.
Waldrop, M. P., Zak, D. R., Sinsabaugh, R. L., Gallo, M., and Lauber, C.: Nitrogen deposition modifies soil carbon storage through changes in microbial enzymatic activity, Ecol. Appl., 14, 1172–1177, https://doi.org/10.1890/03-5120, 2004.
Wambugu, C., Place, F., and Franzel, S.: Research, development and scaling up the adoption of fodder shrub innovations in East Africa, Int. J. Agr. Sustain., 9, 100–109, https://doi.org/10.3763/ijas.2010.0562, 2011.
Wang, H., Yang, Y., Zhang, X., and Tian, G.: carbon footprint analysis for mechanization of maize production based on life cycle assessment: a case study in Jilin Province, China, Sustainability, 7, 15772–15784, https://doi.org/10.3390/su71115772, 2015.
Wiesmeier, M., Spörlein, P., Geuß, U., Hangen, E., Haug, S., Reischl, A., Schilling, B., von Lützow, M., and Kögel-Knabner, I.: Soil organic carbon stocks in southeast Germany (Bavaria) as affected by land use, soil type and sampling depth, Glob. Change Biol., 18, 2233–2245, https://doi.org/10.1111/j.1365-2486.2012.02699.x, 2012.
Xu, Z., Li, Z., Liu, H., Zhang, X., Hao, Q., Cui, Y., Yang, S., Liu, M., Wang, H., Gielen, G., and Song, Z.: Soil organic carbon in particle-size fractions under three grassland types in Inner Mongolia, China. J. Soil Sediment, 18, 1896–1905, https://doi.org/10.1007/s11368-018-1951-1, 2018.
Yan, G., Xing, Y., Xu, L., Wang, J., Meng, W., Wang, Q., Yu, J., Zhang, Z., Wang, Z., Jiang, S., Liu, B., and Han, S.: Nitrogen deposition may enhance soil carbon storage via change of soil respiration dynamic during a spring freeze-thaw cycle period, Sci. Rep., 6, 1–9, https://doi.org/10.1038/srep29134, 2016.
Yang, Y., Liang, Y., Han, X., Chiu, T. Y., Ghosh, A., Chen, H., and Tang, M. The roles of arbuscular mycorrhizal fungi (AMF) in phytoremediation and tree-herb interactions in Pb contaminated soil, Sci. Rep., 6, 1–14, https://doi.org/10.1038/srep20469, 2016.
Yang, Y., Tilman, D., Furey, G., and Lehman. C.: Soil carbon sequestration accelerated by restoration of grassland biodiversity, Nat. Commun., 10, 1–7, https://doi.org/10.1038/s41467-019-08636-w, 2019.
Zar, J. H.: Biostatistical Analysis, 5th Edn., Prentice-Hall/Pearson, Upper Saddle River, xiii, 944 pp., 2010.
Zhao, K., Zhang, W., Zhou, L., Liu, X., Xu, J., and Huang, P.: Modeling transfer of heavy metals in soil-rice system and their risk assessment in paddy fields, Environ. Earth Sci., 59, 519–527, https://doi.org/10.1007/s12665-009-0049-x, 2009.