the Creative Commons Attribution 4.0 License.
the Creative Commons Attribution 4.0 License.
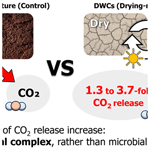
Comprehensive increase in CO2 release by drying–rewetting cycles among Japanese forests and pastureland soils and exploring predictors of increasing magnitude
Yuri Suzuki
Syuntaro Hiradate
Jun Koarashi
Mariko Atarashi-Andoh
Takumi Yomogida
Yuki Kanda
Hirohiko Nagano
It is still difficult to precisely quantify and predict the effects of drying–rewetting cycles (DWCs) on soil carbon dioxide (CO2) release due to the paucity of studies using constant moisture conditions equivalent to the mean water content during DWC incubation. The present study was performed to evaluate overall trends in the effects of DWCs on CO2 release and to explore environmental and soil predictors for variations in the effect size in 10 Japanese forests and pastureland soils variously affected by volcanic ash during their pedogenesis. Over an 84 d incubation period including three DWCs, CO2 release was 1.3- to 3.7-fold greater than under continuous constant moisture conditions (p<0.05) with the same mean water content as in the DWC incubations. Analysis of the relations between this increasing magnitude of CO2 release by DWCs (IF) and various environmental and soil properties revealed significant positive correlations between IF and soil organo-metal complex contents (p<0.05), especially pyrophosphate-extractable aluminum (Alp) content (r=0.74). Molar ratios of soil total carbon (C) and pyrophosphate-extractable C (Cp) to Alp contents and soil-carbon-content-specific CO2 release rate under continuous constant moisture conditions (qCO2_soc) were also correlated with IF (p<0.05). The covariations among Alp, total CpAlp, and CpAlp molar ratios and qCO2_soc suggested Alp to be the primary predictor of IF. Additionally, soil microbial biomass C and nitrogen (N) levels were significantly lower in DWCs than under continuous constant moisture conditions, whereas there was no significant relation between the microbial biomass decrease and IF. The present study showed a comprehensive increase in soil CO2 release by DWC in Japanese forests and pastureland soils, suggesting that Alp is a predictor of the effect size, likely due to vulnerability of organo-Al complexes to DWC.
- Article
(7088 KB) - Full-text XML
-
Supplement
(698 KB) - BibTeX
- EndNote
There is accumulating evidence of climate-change-induced alterations in global water cycling (IPCC, 2021; Allan et al., 2020; Dai, 2012; Donat et al., 2016; Pfleiderer et al., 2019). Of the consequent water regime changes, decreasing precipitation frequency (e.g., number of rainy days) and increasing intensity (e.g., number of heavy rainy days) are becoming more frequent (Dai, 2012; Donat et al., 2016), although decadal trends in annual precipitation levels are not significant over global scales (IPCC, 2021). For example, the annual number of precipitation days in Japan has decreased by 15 % during the past 120 years, whereas the annual number of heavy precipitation days (more than 100 mm in a day) has increased by 26 % (Ministry of Education, Science, and Technology (MEXT) and Japanese Meteorological Agency (JMA), 2020). There is a non-significant trend in annual precipitation level during the same period (MEXT and JAM, 2020). This changing pattern in precipitation is often observed in the temperate region of the Northern Hemisphere (IPCC, 2021) and is related to increased fluctuation of soil water environments, especially DWCs, and consequent alterations in ecosystem functions (Borken and Matzner, 2009; Jin et al., 2023; Zhang et al., 2020, 2023).
Carbon dioxide (CO2) release from soil is an ecosystem process that is sensitive to DWCs (Birch, 1958; Borken and Matzner, 2009; Lee et al., 2002; Nagano et al., 2019; Unger et al., 2010, 2012; Zhang et al., 2020, 2023) and has substantial feedback potential to the ongoing climate change due to its magnitude reaching as much as 7 times greater than anthropogenic CO2 emission on a global scale (Bond-Lamberty and Thomson, 2010; Friedlingstein et al., 2020). The effects of DWCs on soil CO2 release were first shown by Birch (1958) as the marked increase in soil organic matter (SOM) decomposition and CO2 release after the rapid rewetting of dried soil and has since been the subject of intensive investigation (Borken and Matzner, 2009; Kpemoua et al., 2023; Lee et al., 2002, 2004; Miller et al., 2005; Nagano et al., 2019; Unger et al., 2010, 2012; Xiang et al., 2008), including meta-analyses (Kim et al., 2012; Jin et al., 2023; Zhang et al., 2020). However, it is still difficult to precisely quantify and predict the effects of DWCs on soil CO2 release.
The significant uncertainties in the effects of DWCs on soil CO2 release include the inconsistent trends and sizes of effects likely due to the paucity of studies using constant moisture conditions equivalent to the mean water content during DWC incubation (Kpemoua et al., 2023; Zhang et al., 2020, 2023). According to a meta-analysis by Zhang et al. (2020) using 208 data from 34 sites in 29 reports, the effects of DWCs vary according to soil and water contents in continuous constant moisture conditions. In particular, changes in CO2 release rate associated with DWCs ranged from −4 % to +19 % with an average of +4 % in comparison with the medium level of constant moisture content, which should be the same as the mean water content during DWC incubation, whereas only 9 of 38 data representing CO2 release rates were measured for such a medium level of constant moisture conditions (Zhang et al., 2020). Another 29 data were calculated as the average of two CO2 release rates at the wettest and driest water contents of constant moisture conditions, which should be the same as the maximum and minimum water contents in DWC treatment, respectively (Zhang et al., 2020). In the experiment using three Alfisols from Chinese long-term experimental field studies, Zhang et al. (2023) showed similar or somewhat lower CO2 release in the DWC compared with the constant moisture conditions with the same mean water content for the DWC incubation. Using two Luvisols from French long-term field experiment sites, Kpemoua et al. (2023) also showed similar features of changes in CO2 release associated with DWCs, indicating the need for further comparison of CO2 release between DWCs and constant moisture conditions with the same mean water content. In contrast to these studies, Nagano et al. (2019) found a 49 % increase in CO2 release rate associated with DWCs in an Andisol collected from a Japanese forest. This increase was more than double that of another non-volcanic ash soil from the same forest. Thus, there are substantial variations in trends of effects of DWCs in comparison with constant moisture conditions having the same mean water content during incubation, leaving knowledge gaps about environmental and soil predictors for variations in effect sizes. There are roughly three proposed mechanisms for CO2 release increase by DWCs (Schimel, 2018; Barnard et al., 2020): (i) increase in available carbon source via the releases of cellular metabolites from microbial cells destroyed by rewetting after the strong drought, (ii) increase in available carbon source by the releases of carbon from macroaggregates destroyed by repeated DWC, and (iii) changes in the microbial communities in response to transient moisture conditions. Nevertheless, there are still substantial knowledge gaps for critical mechanisms or the relative importance of those mechanisms among multiple soils.
We perform the present study to evaluate overall trends in the effect of DWCs on soil CO2 release and to explore the predictors of variations in its effect size among 10 Japanese forests and pastureland soils. These soils are variously affected by volcanic ash during their pedogenesis and, therefore, include several Andisols, which are known to have a high SOM storage capacity (Morisada et al., 2004), likely due to the protection of SOM from microbial decomposition by abundant reactive minerals and metals in these soils (Asano and Wagai, 2014; Imaya et al., 2007; Shirato et al., 2004). Reactive minerals and metals that contribute to the protection of SOM are iron (Fe) and aluminum (Al), constituting short-range-order minerals and organo-metal complexes (Asano and Wagai, 2014; Rasmussen et al., 2018; Shirato et al., 2004; Wagai et al., 2018). Although global coverage of Andisols is about 1 % (FAO/IIASA/ISRIC/ISS-CAS/JRC, 2009), determination of the responses of carbon cycling in Andisols to DWCs will help in understanding the responses of non-volcanic ash soils because reactive minerals and metals are also essential in high carbon stocks of those soils (Rasmussen et al., 2018; Hall and Thompson, 2022) and may be sensitive to climate and land use management, including water regime (Georgiou et al., 2022; Kramer and Chadwick, 2018).
2.1 Site description and soil sampling
We collected 10 soil samples from depths of 0–5 or 0–10 cm in six forests and a pastureland located in Niigata (six soils from four forests), Ibaraki (two soils from a forest) (Nagano et al., 2019), and Oita (two soils from a forest and a pastureland) (Wijesinghe et al., 2021) prefectures in Japan. Figure 1 and Table 1 present the locations and site characteristics, i.e., elevation, mean annual temperature (MAT), mean annual precipitation (MAP), potential evapotranspiration (PET), and net primary production (NPP). Briefly, all of the investigated sites have a humid temperate climate with MAT of 9.1–10.8 °C and MAP of 1474–2930 mm. All of the forests are dominated by beech (Fagus crenata and Fagus japonica) and oak (Quercus serrata), except for Oita forest which is a deciduous/evergreen mixed forest dominated by Siebold's maple (Acer sieboldianum), Japanese snowbell (Styrax japonicus), and Japanese holly (Ilex crenata). The pastureland in Oita is dominated by Japanese lawn grass (Zoysia japonica), dwarf fountain grass (Pennisetum alopecuroides), cranesbill (Geranium thunbergii), white clover (Trifolium repens), and Indian strawberry (Potentilla indica).
Table 1Environmental properties of Japanese forests and pastureland where investigated soils were collected*.
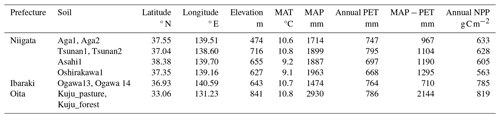
* MAT, MAP, and PET were obtained as averages for 1981–2020 in a global data set of climate and climatic water balance (i.e., TerraClimate) by Abatzoglou et al. (2018). NPP data were obtained as averages for 2001–2020 in the global distribution of NPP estimated from MODIS observation products (i.e., MOD17A3HGF) by Running and Zhao (2021). MAT, mean annual temperature; MAP, mean annual precipitation; PET, potential evapotranspiration; NPP, net primary production.
Soil sampling was conducted in the snow-free season (April to October) of 2021. We also collected soil samples from layers below the target depth (i.e., 0–5 or 0–10 cm depth) down to 50 cm as the maximum depth to examine whether the soil could be classified as Andisol. According to the USDA (United States Department of Agriculture) Soil Taxonomy criteria (USDA Soil Survey Staff, 2022), soils with 60 % or more of the thickness containing more than 20 mg of acid-oxalate-extractable Al (Alo) and Fe (Feo) per 1 g soil within a depth of 0–60 cm are classified as Andisols. According to these criteria, 4 of the 10 soils were Andisols (one each from Niigata and Ibaraki and two from Oita), with non-allophanic properties determined by a high ratio of pyrophosphate-extractable Al (Alp) content to Alo content (; USDA Soil Survey Staff (2022); Fig. S1 in the Supplement). Collected soil samples were transferred to the laboratory and stored at 4 °C before further analysis. Before analysis, soils were gently passed through a 4 mm sieve to remove gravel and plant tissue. Fine roots in the sieved samples were removed with tweezers. In our study, we considered that the soil water content at the soil sampling reflected the ability of soil to hold the water and thus the usual water contents in the field because the soil water content showed significantly positive correlation with water-holding capacity (WHC) (r=0.87, p<0.01). Therefore, CO2 release rate for constant moisture conditions in the present study should represent the release rate under the usual field moisture conditions of each soil.
2.2 Soil analysis
The soil properties analyzed were pH(H2O); electrical conductivity; water content; water-holding capacity (WHC); total carbon (C) and nitrogen (N) contents; particle size distributions as relative compositions of clay, silt, and sand-sized particles; and selectively dissolved Al and Fe contents (Tables 2–4). The pH(H2O) was measured in soil and water mixtures consisting of 1 g of soil and 2.5 mL of water. For measurement of electrical conductivity, 5 mL of water was added to 1 g of soil. Water content was measured by determining the difference in soil weight before and after drying at 105 °C for 24 h. WHC was measured as the difference in soil weight before and after water saturation referring to the Hilgard method (Mabuhay et al., 2003; Ahn et al., 2008). Here, water contents when soil is completely saturated in the Hilgard method should equal zero pF value (0 kPa) as soil water potential. Soil total C and N contents were measured for air-dried and well-ground soil samples using an elemental analyzer (vario PYRO cube; Elementar, Manchester, UK). Particle size distributions were determined using the sedimentation method based on Stokes' law (Miller et al., 1988; Nakai, 1997) using soil mineral particles after removing organic matter by hydrogen peroxide solution digestion. Selectively dissolved metals such as reactive Al and Fe were measured according to the procedure described previously in Nagano et al. (2023). Briefly, the contents of Al and Fe extractable with 2.0 M acid ammonium oxalate (i.e., Alo and Feo, respectively) were measured as contents of organo-metal complexes and short-range-order minerals, while Al and Fe extractable with 0.1 M sodium pyrophosphate solution (i.e., Alp and Fep, respectively) were measured as contents of organo-metal complexes (Asano and Wagai, 2014; Takahashi and Dahlgren, 2016; Wagai et al., 2018). The difference between acid-oxalate- and pyrophosphate-extractable metals (i.e., Alo-p and Feo-p) represented the contents of short-range-order minerals (Courchesne and Turmel, 2008). Contents of Al and Fe in the solution were measured with an inductively coupled plasma–optical emission spectrometer (ICP-OES) (5110; Agilent Technologies, Santa Clara, CA, USA). Carbon concentrations in pyrophosphate-extracted solution (Cp) were also measured with a total organic carbon (TOC) analyzer (TOC-L; Shimadzu, Kyoto, Japan). For soils from Kuju forest and grassland and two Ogawa forests, we also measured C concentration in free light density fraction (fLF) (Leuthold et al., 2023) obtained by the density fractionation method using sodium polytungstate solution at 2.0 g cm−3 (Koarashi et al., 2012), in order to evaluate possible effects of labile C abundance in fLF on CO2 release increase by DWCs.
Table 2Basic properties of 10 Japanese forests and pastureland soils collected from depths of 0–5 or 0–10 cm.
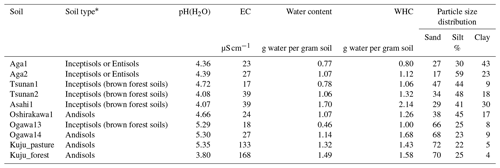
* Andisols were determined according to the USDA Soil Taxonomy criteria (USDA Soil Survey Staff, 2022) based on acid-oxalate-extractable Al and Fe contents (see text for details). All Andisols were non-allophanic. Other soil types were determined using a Japanese soil digital map, i.e., Japan Soil Inventory NARO (2023). EC: elemental carbon.
For the soils after incubation (see Sect. 2.3), soil microbial biomass C and N were measured by the chloroform fumigation extraction method (Vance et al., 1987). Organic C and total N concentrations in 0.5 M potassium sulfate solution used for extraction of fumigated, and nonfumigated soils were measured using another TOC analyzer (TOC-L; Shimadzu) equipped with a total nitrogen (TN) unit (TNM-L, Shimadzu).
2.3 Incubation experiment and soil CO2 release rate measurement
Soils were incubated aerobically at 20 °C for 84 d including three DWCs (i.e., 28 d per cycle). Simultaneously, soils were incubated in the same manner but without DWCs, during which water content of the soils was maintained at a constant level equivalent to the mean water content for the DWC treatment (Fig. 2). A pre-incubation was conducted at the constant water content for 7 d prior to the 84 d incubation. A post-incubation was also conducted at the constant water content for 28 d after the 84 d incubation to evaluate the remaining effect of DWCs on soil CO2 release. We consider that the incubated soils have been aerobic even after the rewetting to increase the water content by twice the WHC because the CO2 concentrations in our experiment never surpassed 1 %; thus the oxygen concentrations in the incubation jar have likely never decreased below 19 % or lower. Also, a sufficiently large volume of our incubation jar (1.0 L) compared to contained soil amounts (i.e., 5.31–10.63 g) and added water contents in the rewetting (i.e., ca. 6 to 7 mL) support the state of aerobic condition during the incubation.
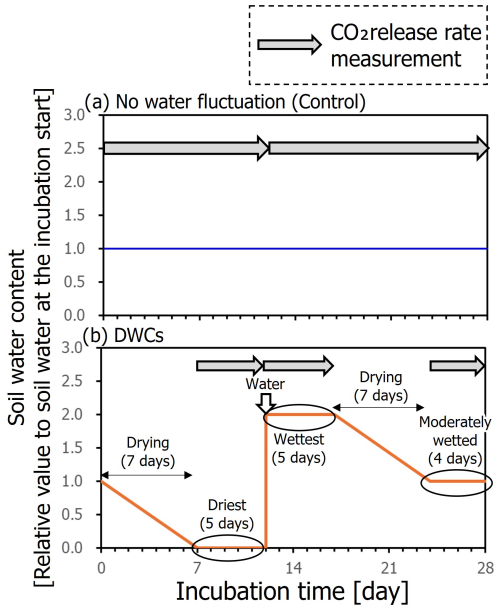
Figure 2Schematic time courses of soil moisture during incubation with constant moisture content (a) and DWCs (b) with measurement period of CO2 release rate.
Mason jars (1.0 L volume; Ball, Buffalo, NY, USA) with lids equipped with tube fitting systems for gas sample collection (Koarashi et al., 2012; Nagano et al., 2019) were used as incubation jars. Small vials (300 mL, SM sample glass vial; Sansyo, Tokyo, Japan) containing 5.31–10.63 g of soil sample depending on water content were placed in mason jars. Under the constant water treatment, we surrounded the small vial with 20 mL of water within the incubation jar to prevent the soil from drying. For each DWC, day 1 to day 7 and day 18 to day 24 were drying stages (Fig. 2), during which the soils were incubated with silica gel (20 g jar−1), which lowered the water content to <5 % WHC by day 7. Day 8 to day 12, day 13 to day 17, and day 25 to day 28 were the driest, wettest, and moderately wet stages, respectively, and CO2 release rates in these three stages were measured using a gas chromatograph equipped with a thermal conductivity detector (GC-14B; Shimadzu). The CO2 release rates were also measured in the pre- and post-incubation periods. At the beginning of the wettest stage, soils were rapidly rewetted with distilled water to double the soil water content from the initial status of DWC incubation. For CO2 release rate measurements, at the start of each stage, the headspace of the incubation jar was flushed with CO2-free air for 15 min at a rate of 0.5 L min−1, and the jar was closed. At the end of each stage, 15 mL of gas sample was collected from the jar using a 20 mL plastic syringe (Terumo, Kyoto, Japan) and stored in a pre-evacuated 5 mL glass vial (SVG-5; Nichiden Rika, Osaka, Japan). Then, the CO2 release rate was determined from the increase in CO2 concentration during this period. After gas sampling, the jars were flushed with CO2-free air and closed for the next incubation stage. For 84 d in the incubations with the constant moisture condition as controls, the CO2 release rates were measured for day 1 to day 12, day 13 to day 28, day 29 to day 40, day 41 to day 56, day 57 to day 68, and day 69 to day 84, in addition to the pre- and post-incubation periods. Soil water contents during the incubation were measured periodically and maintained by adding water to ensure the same mean water content between the two treatments. Even in the drying stage under the DWC treatment for day 1 to day 7 and day 18 to day 24, we conducted measurements of soil water content once or twice. The measurements were performed by weighing those soils. Based on these data, we confirmed that the mean soil water content during DWC incubation was equal to that during constant moisture incubation. All incubations were conducted with three replicates for each treatment and soil.
2.4 Data processing and statistical analysis
The CO2 release rates were compared between the DWC and constant water content treatments. For the DWC treatment, the CO2 release rates in the drying stages (i.e., day 1 to day 7 and day 18 to day 24) could not be measured and therefore had to be estimated to evaluate the mean CO2 release rates for the individual cycle and total of three cycles. The rates in the drying stages were estimated as the mean values of the CO2 release rates measured before and after the period of interest, in the same manner as described previously (Nagano et al., 2019). Then, the effect size of the DWCs on CO2 release (defined here as the increase factor, IF) was quantified as the ratio of CO2 release rate under the DWC condition to that under the constant water content condition (Nagano et al., 2019; Zhang et al., 2020). Here, doubling of CO2 release by DWC resulted in IF of 2, while halving resulted in IF of 0.5.
The pairwise t test was applied to examine the statistical significance of differences in CO2 release rates between the DWC and constant water content treatments with adjustment of site-by-site variations in the metrics. Differences in soil microbial biomass C and N between the two treatments were also evaluated with the pairwise t test. To explore predictors explaining the variation in IF among soils, relations between IF and environmental and soil properties were visualized on scatterplots and evaluated by linear correlation analysis. All statistical analyses were conducted with R 4.1.1 (R Core Team, Vienna, Austria), and p<0.05 was taken to indicate statistical significance.
3.1 Quantifying the effect of DWCs on soil CO2 release
The CO2 release rates under DWC conditions showed large fluctuations for all soils, along with fluctuations in soil water content (Fig. 3). The CO2 release rates in the driest stages (3.5 %–18.2 % of WHC depending on the soil) were 3.0–41.5 µg C per gram dry soil per day. In contrast, CO2 release rates in the wettest stages (90.8 %–201.1 % of WHC) reached 18.8–194.1 µg C per gram dry soil per day. In the moderately wet stages, CO2 release rates were 8.4–63.9 µg C per gram dry soil per day. For the constant water content treatment, CO2 release rates observed for each soil showed little fluctuation during incubation although the rates varied from 7.3 to 69.9 µg C per gram dry soil per day depending on the soil.
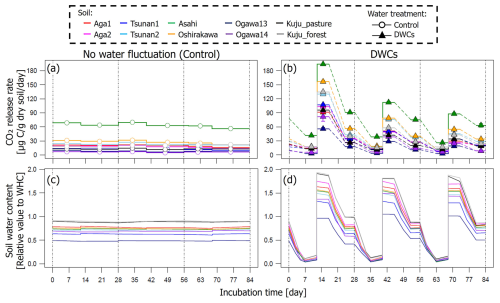
Figure 3Time courses of soil CO2 release rates (a, b) and water contents (c, d) during 84 d incubation under constant water content (a, c) and three DWC conditions (b, d).
For all soils, the observed large fluctuations in CO2 release under DWC treatment resulted in a greater CO2 release rate than under constant water content treatment, although the IF values varied among the soils (Fig. 4). At the wettest stage in the first DWC, the CO2 release rates increased by 47.2–127.7 µg C per gram dry soil per day compared with those under the constant water content conditions, resulting in IF values of 2.9–12.2. For the whole of the first cycle (i.e., the first 28 d), the CO2 release rates increased by 15.4–43.4 µg C per gram dry soil per day under the DWCs compared with the constant water content conditions, resulting in IF values of 1.6–5.2. For the whole incubation period (84 d) including three DWCs, IF values were 1.3–3.7, with an increase in CO2 release rate by 7.4–23.8 µg C per gram dry soil per day by DWCs. These increases in CO2 release by DWCs were observed in all cycles during the 84 d incubation period, whereas no increase was observed in the 28 d post-incubation period after the three DWCs (Fig. S2 in the Supplement). Considering these results, we focused on the IF variations obtained for the whole incubation period, including three DWCs.
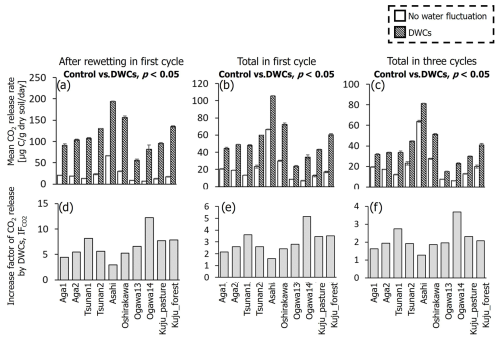
Figure 4Comparisons of mean CO2 release rates after rewetting in the first cycle, the whole of the first cycle, and for the total of three cycles between DWC and constant water content conditions (a–c), and the factor of increase in CO2 release by DWCs (IF) for individual periods (d–f). Statistically significant differences (p<0.05, pairwise t test) in CO2 release rate between the two treatments are presented.
3.2 Exploring predictors of the effect sizes on the increase in CO2 release
Among the environmental and soil physiochemical properties, reactive mineral and metal contents (especially, Alo + 0.5Feo, Alo, Feo, Alp + 0.5Fep, and Alp) in soils showed significant positive correlations with IF (p<0.05; Table 5). In particular, Alp content appeared to be a key predictor of the variation in IF among the soils, given that Alp accounted for most (73 %–99 %) of Alo (Table 4) and showed a higher correlation coefficient than others (Table 5). Scatterplots for Alp content and IF values are presented in Fig. 5. In addition, the molar ratios of soil total C and Cp contents to Alp contents showed significant negative correlations to IF (p<0.05; Table 5, Fig. 5). Here, it should be noted that IF had no significant relationship with soil water content at soil sampling and WHC, suggesting the variation in IF among soils resulting from other than such hydrogenic properties of soils. The SOM quality, such as ratios of total bulk soils and K2SO4-extractable fractions, also had no significant relationship with IF. The insignificant relations of SOM quality to IF were also supported by the fact that Kuju forest and grassland soils having more than doubled differences in fLF abundance showed only 12 % differences in IF, and two Ogawa forest soils having almost identical fLF abundances showed more than doubled differences in IF. The amounts of clay and sand-sized particles showed significant correlations with IF after rewetting in the first cycle (p<0.05, for clay and 0.71 for sand particles). However, those correlations between the particle contents and IF were insignificant for IF for a total of three cycles. Thus, in the present study, reactive mineral and metal content, especially Alp content in soils, was the significant predictor for IF variation among soils, rather than soil water environments, SOM quality, and particle size distributions.
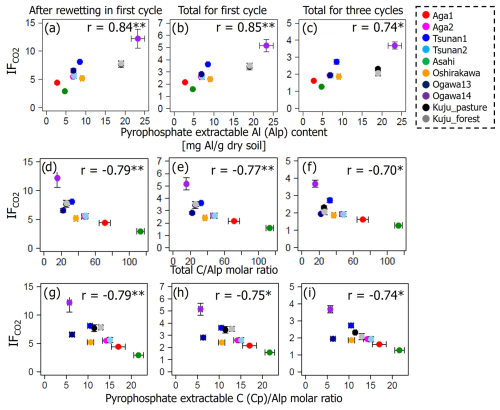
Figure 5Relations between IF and soil pyrophosphate-extractable Al (Alp) content (a–c), total CAlp molar ratio (d–f), and pyrophosphate-extractable C (Cp)Alp molar ratio (g–i). Significant correlation coefficients at p<0.01 and p<0.05 are indicated with single (*) and double asterisks (**), respectively.
Among the additional soil properties related to soil microbial activity and abundance, the soil-C-content-specific CO2 release rate under constant moisture conditions (defined as the CO2 release rate per unit of C in soil, qCO2_soc, in µg CO2-C per milligram of soil carbon per day) showed significant negative correlations with IF values in all incubation stages (p<0.01; Table 6, Fig. 6). Here qCO2_soc should be an index for microbial availability of carbon substrate normalized by total C contents in soils. Thus, using qCO2_soc, we can consider whether the microbially available carbon substrate in interested soil should be much more than in other soils. Microbial biomass N also showed significantly negative correlations with IF values after rewetting in the first cycle and in the whole of the first cycle (p<0.05) but not in the whole incubation period including three cycles (p=0.12).
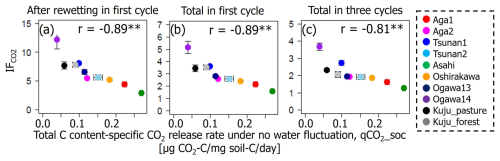
Figure 6Relations between IF and total-C-content-specific CO2 release rate under constant moisture content (qCO2_soc). Significant correlation coefficients at p<0.01 and p<0.05 are indicated with single (*) and double asterisks (**), respectively.
There were also considerable relations among Alp contents, total CAlp molar ratio, and qCO2_soc (Fig. 7). Soil Alp content and total CAlp molar ratio showed a negative correlation at p<0.05. The qCO2_soc showed a positive correlation with total CAlp molar ratio and negative correlation with Alp content at p<0.01. The CpAlp molar ratio also showed similar covariations with these variables, showing a strongly positive correlation with total CAlp molar ratio (r=0.90, p<0.01; Fig. S3 in the Supplement).
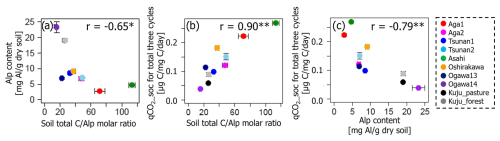
Figure 7Relations among soil Alp contents, total CAlp molar ratio, and qCO2_soc. Significant correlation coefficients at p<0.01 and p<0.05 are indicated with single (*) and double asterisks (**), respectively.
It should be noted that both microbial biomass C and N were significantly lower in soils incubated under DWC conditions than constant water content conditions (p<0.05; Fig. 8). Microbial biomass C under DWC conditions was lower by ≥20.2 % than that under constant water content conditions. Microbial biomass N under DWC conditions was ≥12.6 % lower than under constant water content conditions. Nevertheless, there were no significant correlations between the differences in microbial biomass and the value of IF (p≥0.15; Fig. 9).
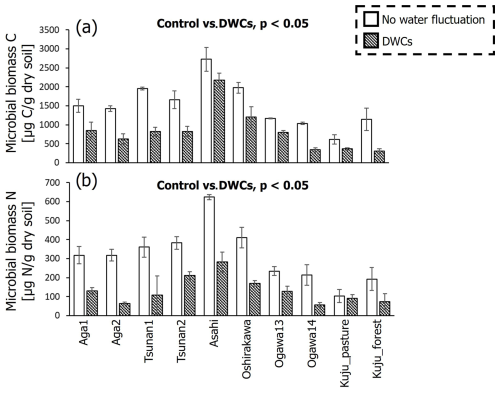
Figure 8Comparisons of microbial biomass C (a) and N (b) after incubation between DWC and constant water content conditions. Significant differences (p<0.05 by pairwise t test) in microbial biomass between two treatments are presented.
An increase in CO2 release due to DWCs was consistently observed across 10 forest and pastureland soils in Japan (Figs. 3 and 4). The comprehensive increases in CO2 release by DWCs were different from the findings of a recent meta-analysis of studies showing no significant increases in CO2 release under DWCs compared with constant water content with an equivalent mean water content during the period of interest (Zhang et al., 2020). Furthermore, our observations quantified the increase in CO2 release due to three DWCs as IF values of 1.3–3.7. The observed effect size of DWCs on the CO2 release from soils was large given that even a 20 % increase in CO2 release from the world's soils can exceed the annual CO2 emission from anthropogenic processes (Friedlingstein et al., 2020).
Furthermore, analyzing the relations between IF and fundamental soil properties showed a significantly positive correlation between IF and soil Alp content (Table 5, Fig. 5). Of the organo-metal complexes measured as pyrophosphate-extractable metals, Fep is known to be sensitive to DWCs, especially in seasonally flooded forests and wetlands, likely due to their vulnerability to redox potential changes caused by alterations to the water regime (Lacroix et al., 2019; Chen et al., 2017, 2018; Chen and Thompson, 2018). Less is known about the vulnerability of Alp to DWCs. However, soil Alp content may be affected by DWCs through changes in soil acidity. A previous field survey conducted in Japanese forest and arable soils by Takahashi et al. (2006) showed that liming of non-allophanic Andisols increased soil pH and decreased Alp content. Miyazawa et al. (2013) verified this behavior by a laboratory incubation experiment for Andisols. Although we did not monitor pH during incubation in the present study, increases in pH after DWCs have been widely observed in upland agricultural (Wang et al., 2020; Meng et al., 2020), seasonally submerged paddy, and wetland soils (Majumdar et al., 2023). Therefore, the acidity mitigation by DWCs would destroy the organo-Al complexes and increase microbially available C through the release of C protected by the organo-Al complexes or other soil elements, such as macro- and microaggregates, which are tightly bonded by organo-Al complexes and thus physically protect organic C from microbial decomposition (Asano and Wagai, 2014; Takahashi and Dahlgren, 2016; Wagai et al., 2018).
Covariations among soil Alp contents, total CAlp molar ratio, and qCO2_soc (Fig. 7) also support the state of soil Alp content as the primary predictor of variations in IF. Negative correlations of soil Alp contents with both total CAlp molar ratio and qCO2_soc suggested that more Alp to total C (i.e., low total CAlp values) strengthens binding between Alp and organic matter, resulting in resistance of organic matter to microbial decomposition under constant water conditions (i.e., low qCO2_soc values). These features among reactive Al and SOM dynamics would result in negative correlations of qCO2_soc and total CAlp molar ratio with IF (Table 6, Figs. 5 and 6). However, such an ability of Alp-rich soils to protect SOM from microbial decomposition likely does not persist under conditions of increased water fluctuations associated with DWCs, as suggested above. Given that amounts of pyrophosphate-extractable C (Cp) in investigated soils (>19 000 µg per gram dry soil; Table 4), which represented C associated with the organo-metal complex, were substantially greater than observed CO2 release increase by DWCs (620–1999 µg C per gram dry soil per 84 d), limited and specific C substrates in the organo-metal complex were likely associated with CO2 increase by DWCs.
We also found substantially lower microbial biomass in soils subjected to DWCs than constant water content conditions (Fig. 8), suggesting a decrease in microbial biomass through the destruction of microbial cells by DWCs (Kaiser et al., 2015; Marumoto et al., 1977, 1982; Marumoto, 1984; Nagano et al., 2023; Unger et al., 2010, 2012). The destruction of microbial cells is expected to release soluble organic matter available for microbes that have survived the DWC and to cause a marked increase in CO2 release after rewetting (Marumoto et al., 1977, 1982; Marumoto, 1984; Nagano et al., 2019; Unger et al., 2010, 2012). Nevertheless, the contribution of microbially derived substances to the increase in CO2 release remained unclear in the present study because of the lack of a significant correlation between IF and the decrease in microbial biomass (Fig. 9). Whereas there was also no significant correlation between the decrease in microbial biomass and the increase in CO2 release due to DWCs (Fig. S4 in the Supplement), the decrease in microbial biomass C (246–1134 µg C per gram dry soil) was within the amount of increase in CO2 release (620–1999 µg C per gram dry soil per 84 d). Therefore, the strict mechanisms of these carbon sources to CO2 release increase, including the persistence and timing of their contribution, still require further work (Schimel, 2018; Barnard et al., 2020), considering the significant contribution of more than two carbon pools to the CO2 release increase (Slessarev and Schimel, 2020; Warren and Manzoni, 2023), as well as carbon likely released by destructions of organo-metal complexes and microbial biomass by DWC, as suggested in the present study.
It should be noted that shortcomings from unmeasured CO2 release during the drying periods for DWCs treatments (i.e., day 1 to day 7 and day 18 to day 24 in each cycle) should be minor, while the linear changes in CO2 release rate during the drying also assumed in other studies (e.g., Nagano et al., 2019; Zhang et al., 2020; Jin et al., 2023). This is because we observed significant relationships between IF and the organo-Al complex, also in IF, after the rewetting in addition to the IF for the first cycle and three cycles in total (Table 5).
Through the present study, a comprehensive increase in CO2 release by DWCs (i.e., 1.3–3.7-fold greater than CO2 release under constant water conditions) was observed in Japanese forest and pastureland soils. These magnitudes of increase in CO2 release were strongly correlated with soil Alp content, total CAlp molar ratio, and total-C-content-specific CO2 release rate under constant water conditions (i.e., qCO2_soc), suggesting the possible vulnerability of SOM protection by organo-Al complexes against DWCs. A decrease in microbial biomass by DWCs was also suggested, whereas their relations with the increase in CO2 release remain to be determined in future studies.
The data that support the findings of this study are available from the corresponding author upon reasonable request.
The supplement related to this article is available online at: https://doi.org/10.5194/soil-11-35-2025-supplement.
YS and HN established the basic research design; conducted all data analyses, including software preparation, validation, and visualization; and wrote the original manuscript. SH, MAA, JK, and HN conducted soil sampling. YS, SH, MAA, JK, and HN contributed to the detailed research design, soil analysis, data validation, interpretation of the results, and editing of the manuscript. TY and YK provided essential support in analysis of soil properties and data. All authors contributed to editing the article and approved the submitted version.
The contact author has declared that none of the authors has any competing interests.
Publisher's note: Copernicus Publications remains neutral with regard to jurisdictional claims made in the text, published maps, institutional affiliations, or any other geographical representation in this paper. While Copernicus Publications makes every effort to include appropriate place names, the final responsibility lies with the authors.
The authors thank Kouki Hikosaka and Hirofumi Kajino of Tohoku University for providing the vegetation information on the Oita forest site. Ayako Tamaki and Masahiro Otaki at Niigata University supported sample preparation and analysis during the experiment and text editing in preparing the manuscript. Rei Shibata at Niigata University and Masami Tsukahara, Kosuke Ito, and Tatsuki Tanaka at Niigata Prefectural Forest Research Institute helped in site selection and soil sampling in Niigata Prefecture, Japan. Naoki Harada, Kazuki Suzuki, and Asiloglu Rasit of Niigata University and Hiroyuki Sase and Rieko Urakawa of the Asia Center for Air Pollution Research (ACAP) provided valuable preliminary discussion in writing the manuscript. The authors also thank Misuzu Kaminaga, Kikuko Yoshigaki, Makiko Ishihara, and Kazumi Matsumura at JAEA for support with laboratory work.
This research has been supported by the Japan Society for the Promotion of Science (JSPS) KAKENHI (grant nos. 21H02231, 21H05313, and 22H05717).
This paper was edited by Axel Don and reviewed by Haicheng Zhang and one anonymous referee.
Abatzoglou, J. T., Dobrowski, S. Z., Parks, S. A., and Hegewisch, K. C.: TerraClimate, a high-resolution global dataset of monthly climate and climatic water balance from 1958–2015, Scientific Data, 5, 170191, https://doi.org/10.1038/sdata.2017.191, 2018. a
Ahn, H. K., Richard, T. L., and Glanville, T. D.: Optimum moisture levels for biodegradation of mortality composting envelope materials, Waste Manage., 28, 1411–1416, https://doi.org/10.1016/J.WASMAN.2007.05.022, 2008. a
Allan, R. P., Barlow, M., Byrne, M. P., Cherchi, A., Douville, H., Fowler, H. J., Gan, T. Y., Pendergrass, A. G., Rosenfeld, D., Swann, A. L., Wilcox, L. J., and Zolina, O.: Advances in understanding large-scale responses of the water cycle to climate change, Ann. NY Acad. Sci., 1472, 49–75, https://doi.org/10.1111/NYAS.14337, 2020. a
Asano, M. and Wagai, R.: Evidence of aggregate hierarchy at micro- to submicron scales in an allophanic Andisol, Geoderma, 216, 62–74, https://doi.org/10.1016/j.geoderma.2013.10.005, 2014. a, b, c, d
Barnard, R. L., Blazewicz, S. J., and Firestone, M. K.: Rewetting of soil: Revisiting the origin of soil CO2 emissions, Soil Biol. Biochem., 147, 107819, https://doi.org/10.1016/j.soilbio.2020.107819, 2020. a, b
Birch, H. F.: The effect of soil drying on humus decomposition and nitrogen availability, Plant Soil, 10, 9–31, https://doi.org/10.1007/BF01343734, 1958. a, b
Bond-Lamberty, B. and Thomson, A.: Temperature-associated increases in the global soil respiration record, Nature, 464, 579–582, https://doi.org/10.1038/nature08930, 2010. a
Borken, W. and Matzner, E.: Reappraisal of drying and wetting effects on C and N mineralization and fluxes in soils, Global Change Biol., 15, 808–824, https://doi.org/10.1111/j.1365-2486.2008.01681.x, 2009. a, b, c
Chen, C. and Thompson, A.: Ferrous Iron Oxidation under Varying pO2 Levels: The Effect of Fe(III)/Al(III) Oxide Minerals and Organic Matter, Environ. Sci. Technol., 52, 597–606, https://doi.org/10.1021/acs.est.7b05102, 2018. a
Chen, C., Kukkadapu, R. K., Lazareva, O., and Sparks, D. L.: Solid-Phase Fe Speciation along the Vertical Redox Gradients in Floodplains using XAS and Mössbauer Spectroscopies, Environ. Sci. Technol., 51, 7903–7912, 2017. a
Chen, C., Meile, C., Wilmoth, J., Barcellos, D., and Thompson, A.: Influence of pO2 on Iron Redox Cycling and Anaerobic Organic Carbon Mineralization in a Humid Tropical Forest Soil, Environ. Sci. Technol., 52, 7709–7719, https://doi.org/10.1021/acs.est.8b01368, 2018. a
Courchesne, F. and Turmel, M.-C.: Extractable Al, Fe, Mn, and Si, in: Soil Sampling and Methods of Analysis, 2nd edn., Chap. 26, edited by: Carter, M. and Gregorich, E., CRC Press, 307–316, ISBN-13: 978-0-8493-3586-0, 2008. a
Dai, A.: Increasing drought under global warming in observations and models, Nat. Clim. Change, 3, 52–58, https://doi.org/10.1038/nclimate1633, 2012. a, b
Donat, M. G., Lowry, A. L., Alexander, L. V., O'Gorman, P. A., and Maher, N.: More extreme precipitation in the world's dry and wet regions, Nat. Clim. Change, 6, 508–513, https://doi.org/10.1038/nclimate2941, 2016. a, b
FAO/IIASA/ISRIC/ISS-CAS/JRC: Harmonized World Soil Database (version 1.1), FAO, Italy and IIASA, Laxenburg, Austria, 2009. a
Friedlingstein, P., O'Sullivan, M., Jones, M. W., Andrew, R. M., Hauck, J., Olsen, A., Peters, G. P., Peters, W., Pongratz, J., Sitch, S., Le Quéré, C., Canadell, J. G., Ciais, P., Jackson, R. B., Alin, S., Aragão, L. E. O. C., Arneth, A., Arora, V., Bates, N. R., Becker, M., Benoit-Cattin, A., Bittig, H. C., Bopp, L., Bultan, S., Chandra, N., Chevallier, F., Chini, L. P., Evans, W., Florentie, L., Forster, P. M., Gasser, T., Gehlen, M., Gilfillan, D., Gkritzalis, T., Gregor, L., Gruber, N., Harris, I., Hartung, K., Haverd, V., Houghton, R. A., Ilyina, T., Jain, A. K., Joetzjer, E., Kadono, K., Kato, E., Kitidis, V., Korsbakken, J. I., Landschützer, P., Lefèvre, N., Lenton, A., Lienert, S., Liu, Z., Lombardozzi, D., Marland, G., Metzl, N., Munro, D. R., Nabel, J. E. M. S., Nakaoka, S.-I., Niwa, Y., O'Brien, K., Ono, T., Palmer, P. I., Pierrot, D., Poulter, B., Resplandy, L., Robertson, E., Rödenbeck, C., Schwinger, J., Séférian, R., Skjelvan, I., Smith, A. J. P., Sutton, A. J., Tanhua, T., Tans, P. P., Tian, H., Tilbrook, B., van der Werf, G., Vuichard, N., Walker, A. P., Wanninkhof, R., Watson, A. J., Willis, D., Wiltshire, A. J., Yuan, W., Yue, X., and Zaehle, S.: Global Carbon Budget 2020, Earth Syst. Sci. Data, 12, 3269–3340, https://doi.org/10.5194/essd-12-3269-2020, 2020. a, b
Georgiou, K., Jackson, R. B., Vindušková, O., Abramoff, R. Z., Ahlström, A., Feng, W., Harden, J. W., Pellegrini, A. F., Polley, H. W., Soong, J. L., Riley, W. J., and Torn, M. S.: Global stocks and capacity of mineral-associated soil organic carbon, Nat. Commun., 13, 1–12, https://doi.org/10.1038/s41467-022-31540-9, 2022. a
Hall, S. J. and Thompson, A.: What do relationships between extractable metals and soil organic carbon concentrations mean?, Soil Sci. Soc. Am. J., 86, 195–208, https://doi.org/10.1002/saj2.20343, 2022. a
Imaya, A., Inagaki, Y., Tanaka, N., and Ohta, S.: Free oxides and short-range ordered mineral properties of brown forest soils developed from different parent materials in the submontane zone of the Kanto and Chubu districts, Japan, Soil Sci. Plant Nutr., 53, 621–633, https://doi.org/10.1111/j.1747-0765.2007.00175.x, 2007. a
IPCC: Climate Change 2021: The Physical Science Basis. Working Group I Contribution to the IPCC Sixth Assessment Report, Cambridge University Press, United Kingdom and New York, https://doi.org/10.1017/9781009157896, 2021. a, b, c
Jin, X., Wu, F., Wu, Q., Heděnec, P., Peng, Y., Wang, Z., and Yue, K.: Effects of drying-rewetting cycles on the fluxes of soil greenhouse gases, Heliyon, 9, e12984, https://doi.org/10.1016/j.heliyon.2023.e12984, 2023. a, b, c
Kaiser, M., Kleber, M., and Berhe, A. A.: How air-drying and rewetting modify soil organic matter characteristics: An assessment to improve data interpretation and inference, Soil Biol. Biochem., 80, 324–340, https://doi.org/10.1016/j.soilbio.2014.10.018, 2015. a
Kim, D.-G., Vargas, R., Bond-Lamberty, B., and Turetsky, M. R.: Effects of soil rewetting and thawing on soil gas fluxes: a review of current literature and suggestions for future research, Biogeosciences, 9, 2459–2483, https://doi.org/10.5194/bg-9-2459-2012, 2012. a
Koarashi, J., Hockaday, W. C., Masiello, C. A., and Trumbore, S. E.: Dynamics of decadally cycling carbon in subsurface soils, J. Geophys. Res.-Biogeo., 117, 1–13, https://doi.org/10.1029/2012JG002034, 2012. a, b
Kpemoua, T. P., Barré, P., Houot, S., and Claire, C.: Accurate evaluation of the Birch effect requires continuous CO2 measurements and relevant controls, Soil Biol. Biochem., 180, 109007, https://doi.org/10.1016/J.SOILBIO.2023.109007, 2023. a, b, c
Kramer, M. G. and Chadwick, O. A.: Climate-driven thresholds in reactive mineral retention of soil carbon at the global scale, Nat. Clim. Change, 8, 1104–1108, https://doi.org/10.1038/s41558-018-0341-4, 2018. a
LaCroix, R. E., Tfaily, M. M., McCreight, M., Jones, M. E., Spokas, L., and Keiluweit, M.: Shifting mineral and redox controls on carbon cycling in seasonally flooded mineral soils, Biogeosciences, 16, 2573–2589, https://doi.org/10.5194/bg-16-2573-2019, 2019. a
Lee, M.-S., Nakane, K., Nakatsubo, T., Mo, W.-H., and Koizumi, H.: Effects of rainfall events on soil CO2 flux in a cool temperate deciduous broad-leaved forest, Ecol. Res., 17, 401–409, https://doi.org/10.1046/j.1440-1703.2002.00498.x, 2002. a, b
Lee, X., Wu, H. J., Sigler, J., Oishi, C., and Siccama, T.: Rapid and transient response of soil respiration to rain, Global Change Biol., 10, 1017–1026, https://doi.org/10.1111/j.1529-8817.2003.00787.x, 2004. a
Leuthold, S. J., Haddix, M. L., Lavallee, J., and Cotrufo, M. F.: Physical fractionation techniques, Encyclopedia of Soils in the Environment, 2nd edn., 68–80, https://doi.org/10.1016/B978-0-12-822974-3.00067-7, 2023. a
Mabuhay, J. A., Nakagoshi, N., and Horikoshi, T.: Microbial biomass and abundance after forest fire in pine forests in Japan, Ecol. Res., 18, 431–441, https://doi.org/10.1046/j.1440-1703.2003.00567.x, 2003. a
Majumdar, A., Dubey, P. K., Giri, B., Moulick, D., Srivastava, A. K., Roychowdhury, T., Bose, S., and Jaiswal, M. K.: Combined effects of dry-wet irrigation, redox changes and microbial diversity on soil nutrient bioavailability in the rice field, Soil Till. Res., 232, 105752, https://doi.org/10.1016/J.STILL.2023.105752, 2023. a
Marumoto, T.: Mineralization of C and N from microbial biomass in paddy soil, Plant Soil, 76, 165–173, https://doi.org/10.1007/BF02205577, 1984. a, b
Marumoto, T., Kai, H., Yoshida, T., and Harada, T.: Drying effect on mineralizations of microbial cells and their cell walls in soil and contribution of microbial cell walls as a source of decomposable soil organic matter due to drying, Soil Sci. Plant Nutr., 23, 9–19, https://doi.org/10.1080/00380768.1977.10433017, 1977. a, b
Marumoto, T., Anderson, J. P., and Domsch, K. H.: Decomposition of 14C- and 15N-labelled microbial cells in soil, Soil Biol. Biochem., 14, 461–467, https://doi.org/10.1016/0038-0717(82)90105-5, 1982. a, b
Meng, Z., Huang, S., Xu, T., Deng, Y., Lin, Z., and Wang, X.: Transport and transformation of Cd between biochar and soil under combined dry-wet and freeze-thaw aging, Environ. Pollut., 263, 114449, https://doi.org/10.1016/J.ENVPOL.2020.114449, 2020. a
MEXT and JAM: Climate Change in Japan: Report on Assessment of Observed/Projected Climate Changes Relating to the Atmosphere, Land and Oceans., Tech. rep., Ministry of Education, Culture, Sports, Science and Technology, Japan Meteorological Agency, https://www.data.jma.go.jp/cpdinfo/ccj/index.html (last access: 9 January 2025), 2020. a
Miller, A. E., Schimel, J. P., Meixner, T., Sickman, J. O., and Melack, J. M.: Episodic rewetting enhances carbon and nitrogen release from chaparral soils, Soil Biol. Biochem., 37, 2195–2204, https://doi.org/10.1016/j.soilbio.2005.03.021, 2005. a
Miller, W. P., Radcliffe, D. E., and Miller, D. M.: An historical perspective on the theory and practice of soil mechanical analysis, Journal of Agronomic Education, 17, 24–28, 1988. a
Miyazawa, M., Takahashi, T., Sato, T., Kanno, H., and Nanzyo, M.: Factors controlling accumulation and decomposition of organic carbon in humus horizons of Andosols, Biol. Fert. Soils, 49, 929–938, https://doi.org/10.1007/s00374-013-0792-8, 2013. a
Morisada, K., Ono, K., and Kanomata, H.: Organic carbon stock in forest soils in Japan, Geoderma, 119, 21–32, https://doi.org/10.1016/S0016-7061(03)00220-9, 2004. a
Nagano, H., Atarashi-Andoh, M., and Koarashi, J.: Effect of dry-wet cycles on carbon dioxide release from two different volcanic ash soils in a Japanese temperate forest, Soil Sci. Plant Nutr., 65, 525–533, https://doi.org/10.1080/00380768.2019.1649976, 2019. a, b, c, d, e, f, g, h, i
Nagano, H., Atarashi-Andoh, M., Tanaka, S., Yomogida, T., Kozai, N., and Koarashi, J.: Stable C and N isotope abundances in water-extractable organic matter from air-dried soils as potential indices of microbially utilized organic matter, Frontiers in Forests and Global Change, 6, 1228053, https://doi.org/10.3389/ffgc.2023.1228053, 2023. a, b
Nakai, M.: Soil particle distribution [The pipette method], in: Dojo-Kankyo-Bunseki-Ho (Analytical Methods for Soil Environment), edited by: Editorial Committee for Analytical Methods for Soil Environment, Hakuyusha, Tokyo, 24–29, ISBN-13: 978-4-8268-0168-3, 1997. a
NARO: Japan Soil Inventory, https://soil-inventory.rad.naro.go.jp/index.html (last access: 9 January 2025), 2023. a
Pfleiderer, P., Schleussner, C.-F., Kornhuber, K., and Coumou, D.: Summer weather becomes more persistent in a 2 °C world, Nat. Clim. Change, 9, 666–671, https://doi.org/10.1038/s41558-019-0555-0, 2019. a
Rasmussen, C., Heckman, K., Wieder, W. R., Keiluweit, M., Lawrence, C. R., Berhe, A. A., Blankinship, J. C., Crow, S. E., Druhan, J. L., Hicks Pries, C. E., Marin-Spiotta, E., Plante, A. F., Schädel, C., Schimel, J. P., Sierra, C. A., Thompson, A., and Wagai, R.: Beyond clay: towards an improved set of variables for predicting soil organic matter content, Biogeochemistry, 137, 297–306, https://doi.org/10.1007/s10533-018-0424-3, 2018. a, b
Running, S. and Zhao, M.: MODIS/Terra Net Primary Production Gap-Filled Yearly L4 Global 500 m SIN Grid V061, https://doi.org/10.5067/MODIS/MOD17A3HGF.061, 2021. a
Schimel, J. P.: Life in Dry Soils: Effects of Drought on Soil Microbial Communities and Processes, Annu. Rev. Ecol. Evol. S., 49, 409–432, https://doi.org/10.1146/annurev-ecolsys-110617-062614, 2018. a, b
Shirato, Y., Hakamata, T., and Taniyama, I.: Modified rothamsted carbon model for andosols and its validation: changing humus decomposition rate constant with pyrophosphate-extractable Al, Soil Sci. Plant Nutr., 50, 149–158, https://doi.org/10.1080/00380768.2004.10408463, 2004. a, b
Slessarev, E. W. and Schimel, J. P.: Partitioning sources of CO2 emission after soil wetting using high-resolution observations and minimal models, Soil Biol. Biochem., 143, 107753, https://doi.org/10.1016/j.soilbio.2020.107753, 2020. a
Takahashi, T. and Dahlgren, R. A.: Nature, properties and function of aluminum-humus complexes in volcanic soils, Geoderma, 263, 110–121, https://doi.org/10.1016/j.geoderma.2015.08.032, 2016. a, b
Takahashi, T., Ikeda, Y., Fujita, K., and Nanzyo, M.: Effect of liming on organically complexed aluminum of nonallophanic Andosols from northeastern Japan, Geoderma, 130, 26–34, 2006. a
Unger, S., Máguas, C., Pereira, J. S., David, T. S., and Werner, C.: The influence of precipitation pulses on soil respiration – Assessing the “Birch effect” by stable carbon isotopes, Soil Biol. Biochem., 42, 1800–1810, https://doi.org/10.1016/j.soilbio.2010.06.019, 2010. a, b, c, d
Unger, S., Máguas, C., Pereira, J. S., David, T. S., and Werner, C.: Interpreting post-drought rewetting effects on soil and ecosystem carbon dynamics in a Mediterranean oak savannah, Agr. Forest Meteorol., 154–155, 9–18, https://doi.org/10.1016/j.agrformet.2011.10.007, 2012. a, b, c, d
USDA Soil Survey Staff: Keys to Soil Taxonomy, USDA-Natural Resources Conservation Service, 13th edn., https://www.nrcs.usda.gov/resources/guides-and-instructions/keys-to-soil-taxonomy#keys (last access: 9 January 2025), 2022. a, b, c
Vance, E. D., Brookes, P. C., and Jenkinson, D. S.: An extraction method for measuring soil microbial biomass C, Soil Biol. Biochem., 19, 703–707, https://doi.org/10.1016/0038-0717(87)90052-6, 1987. a
Wagai, R., Kajiura, M., Uchida, M., and Asano, M.: Distinctive Roles of Two Aggregate Binding Agents in Allophanic Andisols: Young Carbon and Poorly-Crystalline Metal Phases with Old Carbon, Soil Systems, 2, 29, https://doi.org/10.3390/soilsystems2020029, 2018. a, b, c
Wang, D., Felice, M. L., and Scow, K. M.: Impacts and interactions of biochar and biosolids on agricultural soil microbial communities during dry and wet-dry cycles, Appl. Soil Ecol., 152, 103570, https://doi.org/10.1016/J.APSOIL.2020.103570, 2020. a
Warren, C. R. and Manzoni, S.: When dry soil is re-wet, trehalose is respired instead of supporting microbial growth, Soil Biol. Biochem., 184, 109121, https://doi.org/10.1016/J.SOILBIO.2023.109121, 2023. a
Wijesinghe, J. N., Asaoka, H., Mori, Y., and Hiradate, S.: Depth profile of plant nutrients and acidity in a non-allophanic Andosol as affected by a half-century of fertilization, Soil Sci. Plant Nutr., 67, 211–221, https://doi.org/10.1080/00380768.2021.1887711, 2021. a
Xiang, S. R., Doyle, A., Holden, P. A., and Schimel, J. P.: Drying and rewetting effects on C and N mineralization and microbial activity in surface and subsurface California grassland soils, Soil Biol. Biochem., 40, 2281–2289, https://doi.org/10.1016/j.soilbio.2008.05.004, 2008. a
Zhang, S., Yu, Z., Lin, J., and Zhu, B.: Responses of soil carbon decomposition to drying-rewetting cycles: A meta-analysis, Geoderma, 361, 114069, https://doi.org/10.1016/j.geoderma.2019.114069, 2020. a, b, c, d, e, f, g, h, i, j
Zhang, S., Lin, J., Wang, P., and Zhu, B.: The direct and legacy effects of drying-rewetting cycles on active and relatively resistant soil carbon decomposition, Land Degrad. Dev., 34, 2124–2135, https://doi.org/10.1002/ldr.4594, 2023. a, b, c, d