the Creative Commons Attribution 4.0 License.
the Creative Commons Attribution 4.0 License.
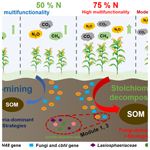
Moderate N fertilizer reduction with straw return modulates cropland functions and microbial traits in a meadow soil
Yan Duan
Minghui Cao
Wenling Zhong
Yuming Wang
Zheng Ni
Mengxia Zhang
Jiangye Li
Yumei Li
Xianghai Meng
Lifang Wu
Nitrogen (N) fertilization has received worldwide attention due to its benefits with regard to soil fertility and productivity, but excess N application also causes an array of ecosystem degenerations, such as greenhouse gas emissions. Generally, soil microorganisms are considered to be involved in upholding a variety of soil functions. However, the linkages between soil cropland properties and microbial traits under different N fertilizer application rates remain uncertain. To address this, a 4-year in situ field experiment was conducted in a meadow soil from the Northeast China Plain after straw return with the following treatments combined with regular phosphorus (P) and potassium (K) fertilization: (i) regular N fertilizer (N + PK), (ii) 25 % N fertilizer reduction (0.75N + PK), (iii) 50 % N fertilizer reduction (0.5N + PK), and (IV) no N fertilizer (PK). Cropland properties and microbial traits responded distinctly to the different N fertilizer rates. Treatment 0.75N + PK had overall positive effects on soil fertility, productivity, straw decomposition, and microbial abundance and functioning and alleviated greenhouse effects. Specifically, no significant difference was observed in soil organic carbon (SOC), total N, P content, straw C, N release amounts, microbial biomass C, N content, and cellulase and N-acetyl-D-glucosaminidase activities, which were all significantly higher than in 0.5N + PK and PK. Greenhouse gas emissions was reduced with the decreasing N input levels. Moreover, the highest straw biomass and yield were measured in 0.75N + PK, which were significantly higher than in 0.5N + PK and PK. Meanwhile, 0.75N + PK up-regulated aboveground biomass and soil C:N and thus increased the abundance of genes encoding cellulose-degrading enzymes, which may imply the potential ability of C and N turnover. In addition, most observed changes in cropland properties were strongly associated with microbial modules and keystone taxa. The Lasiosphaeriaceae within the module-1 community showed significant positive correlations with straw degradation rate and C and N release, while the Terrimonas within the module-3 community showed a significant positive correlation with production, which was conducive to soil multifunctionality. Therefore, our results suggest that straw return with 25 % chemical N fertilizer reduction is optimal for achieving soil functions. This study highlights the importance of abiotic and biotic factors in soil health and supports green agricultural development by optimizing N fertilizer rates in meadow soil after straw return.
- Article
(3229 KB) - Full-text XML
-
Supplement
(750 KB) - BibTeX
- EndNote
Multiple soil functions are indicators of soil health (Kihara et al., 2020; Lehmann et al., 2021), which refers to the ability of soil as a vital living system to sustainably increase crop productivity, improve environmental quality, tackle climate change, and promote plant and animal health (de Bello et al., 2010; Tang et al., 2019). Intensive agriculture has posed a wide range of threats to agroecosystem functions (Robertson et al., 2014; Allan et al., 2015). Irrational application of chemical fertilizers, especially nitrogen (N), is ubiquitous with regard to achieving high crop yields in response to population surges globally (Shi et al., 2019). In fact, N is considered to be the essential macronutrient for all biota, while excessive N fertilizer inputs not only reduce soil fertility and productivity but also lead to environmental burdens (Trost et al., 2016). Recent research indicated that the appropriate reduction of N fertilizer input can not only maintain crop yield by increasing N fertilizer use efficiency but also promote soil health by regulating the soil C:N ratio (Chen et al., 2014). However, excessive reduction of N fertilizer input can lead to an “N-mining” effect, resulting in the loss of soil organic matter, which reduces crop yields (Chen et al., 2014). Therefore, how to achieve agroecosystem functions by regulating N fertilizer application rates needs to be fully assessed.
Straw return has also been widely applied as a major measure to moderate cropland traits (Xu et al., 2021). Plant residues contain abundant N that further affects soil fertility and productivity (Pan et al., 2009; Liu et al., 2014). Thus, the straw-derived N released during degradation is an important source that may serve as a partial substitute for chemical N fertilizer application (Wang et al., 2017; Latifmanesh et al., 2020). However, crop fields suffering from superabundant exogenous materials may result in negative effects on the ecosystem. For example, excess organic materials usually have low reutilization efficiency (Hou et al., 2020); the majority of N in straw is released into the atmosphere as oxynitrides, such as nitrous oxide (N2O) (Wang et al., 2019; Sun et al., 2021). Subsequent literature highlighted that straw return significantly elevates greenhouse gas emissions so that less than 15 % of straw-derived N can be transformed into soil and become soil organic matter (SOM) (Yin et al., 2018; Wu et al., 2019). However, the potential for the partial substitution of straw for chemical N fertilizer application is still unclear.
Soil microorganisms are the drivers of soil functions (Handa et al., 2014; Wagg et al., 2014). Agronomic management for such multifunctionality has prompted research into the role that microbes play in providing the desired rates of multiple ecosystem processes (Gong et al., 2020). Fertilization-induced changes in microbial communities and functions are fundamental to the regulation of a variety of ecosystem multifunctionalities, including SOM formation, greenhouse gas emissions, litter decomposition, and crop production (Dominati et al., 2014). For example, Ning et al. (2020) found that long-term manure use increased the abundance of specific fungi, which was involved in yield improvement. Duan et al. (2021) indicated that adequate N input improved the cellulose-degrading ability of bacteria. To date, we still lack empirical evidence of the linkages among N fertilizers, specific microbial communities or functions, and multiple cropland traits, and the diverse soil functions driven by complex microbial traits under different N fertilizer rates are seldom clarified.
Microorganisms contribute to soil functions by modulating microbial traits (e.g., function, community composition, and succession) which are influenced by different N fertilizer input levels (Bradford et al., 2014; Chen et al., 2019a). Generally, bacteria and fungi are the main drivers of straw labile- and recalcitrant-component decomposition, respectively (Frey et al., 2013; Ge et al., 2017; Hogberg et al., 2007). In addition, microbial module communities and keystone taxa have been used to provide satisfactory explanations for soil functions. Chen et al. (2019b) found that particular microbial modules participated in N and phosphorus (P) turnover in a Cambisol. Actinobacteria have been extensively studied and can be considered to be the main degraders of straw by secreting cellulase (Bao et al., 2021). C, N, and P stoichiometry have profound impacts on microbial in vivo metabolism and ex vivo modification processes (Chen et al., 2016). Nevertheless, the knowledge of the microbial mechanisms that modulate cropland traits in response to N fertilizer input levels is still rudimentary.
As an important grain-producing region, the Northeast China Plain contributes to more than 20 % of the total grain yield in China (Li et al., 2017; Zhao et al., 2018). Here, a field experiment was conducted to reveal the influences of N input levels over soil ecosystem multifunctionality and associated microbial traits. In the present study, two hypotheses were tested: (i) soil cropland traits would show distinct responses to N fertilizer input levels, and (ii) the changes in cropland traits would be linked to specific microbial traits. The purpose of this study is to optimize the N fertilizer application rate to achieve soil ecosystem multifunctionality and to explore the potential microbial mechanism in Mollisol.
2.1 Site description and sampling
A field experiment conducted under contrasting inorganic N fertilizer input levels was established in 2018 in the town of Wenchuan (44°59′61′′ N, 129°59′18′′ E), Mudanjiang City, Heilongjiang Province, Northeast China Plain, which is an important grain-producing area. This region has a typical temperate continental monsoon climate with an average annual temperature of 4.3 °C and a mean annual precipitation of 579.7 mm. The soil is classified as a meadow soil according to the US Soil Taxonomy (USST). The cropping system was continuous maize (Zea mays L.) monoculture. Four treatments received different N fertilizer input levels after straw return to the field for 4 years as follows: (1) regular chemical fertilization, hereafter N + PK (300 kg urea (N 46 %) ha−1 yr−1, 250 kg calcium triple superphosphate (P2O5 46 %) ha−1 yr−1, 150 kg potassium chloride (K2O 50 %) ha−1 yr−1); (2) 25 % reduction in N fertilizer, hereafter 0.75N + PK (225 kg urea ha−1 yr−1, 250 kg calcium triple superphosphate ha−1 yr−1, 150 kg potassium chloride ha−1 yr−1); (3) 50 % reduction in N fertilizer, hereafter 0.50N + PK (150 kg urea ha−1 yr−1, 250 kg calcium triple superphosphate ha−1 yr−1, 150 kg potassium chloride ha−1 yr−1); and (4) no N fertilizer, hereafter PK (250 kg calcium triple superphosphate ha−1 yr−1, 150 kg potassium chloride ha−1 yr−1). Urea is the only nitrogen source in the soil. All straw and chemical fertilizers were applied with shallow tillage to 20 cm. Straw was cut into pieces of less than 5 cm and were input after the harvest in October, while the chemical fertilizers were applied during ploughing in May of the next year. All other normal management practices were consistent among treatments during the experiment. Before the experiment, the initial soil contained 18.74 g kg−1 SOC (soil organic carbon), 1.03 g kg−1 total N, and 0.54 g kg−1 total P, with a pH of 7.37 (H2O). The yield and some of the soil chemical properties under different bulk soil treatments during the experimental process are shown in Table S1 in the Supplement.
Soils were sampled after the maize harvest in October 2021. A randomized complete-block design consisting of four treatments with three replications was adopted in this study. Each field plot was 4.5 m × 15 m. We took nine soil cores (5 cm diameter) from the top 20 cm of bulk soil in each plot. Each soil sample consisted of a mixture of subsamples randomly collected from nine different positions in the same plot. In total, 12 soil samples were collected from four treatments. Each treatment contained three replicates. Soils were sieved through a 2 mm mesh, the mineral particles and plant roots were carefully removed, and then the soils were homogenized and stored in an incubator at 4 °C in a 40 % moisture environment. One part of the bulk soil sample was air-dried to measure basal soil properties, and the other part was used for microbial molecular analysis.
2.2 The field straw decomposition and carbon and nitrogen release experiments
The ditch-buried straw decomposition experiment was conducted using litter nylon bags. Maize straw materials were collected after maize harvesting in 2020 and were air-dried; 10 g of maize straw was cut to 2 cm in length and put into nylon litter bags, which were then sealed via heat sealing. The nylon bags were 6 cm × 10 cm in size and were made of 200 mesh nylon fabric, which permitted the free transfer of microorganisms between the nylon bags and soil. On 2 May 2021, litter bags containing straw were buried at 10 cm depth in a spatially random design to prevent bags associated with a given decomposition stage being placed together in space. The litter bags were collected after the harvest on 1 October 2021.
The straw decomposition ratio was calculated based on dry weight loss as (dry initial mass − dry final mass) dry initial mass. The straw C concentration was measured by titrimetry after oxidation with a mixture of H2SO4 and K2Cr2O7. Total N, P, and K were determined using the Kjeldahl, molybdenum blue colorimetry, and flame photometry methods, respectively. All methods have been described by Lu (2000). The initial and sampled maize straw material properties are shown in Table S2 in the Supplement. The amounts of total straw C and N released were calculated using the following equation:
the amounts of total straw C and N released = (initial C (or N) content × dry initial mass − final C (or N) content × dry final mass) × aboveground biomass.
2.3 Measurement of soil properties and assessment of cropland traits
Soil pH was measured at a soil : water ratio of 1:2.5 (weight weight). Air-dried soil and 25 mL of deionized water were shaken together for 1 min and left to settle for 30 min, and the soil pH was determined using an electrode. Soil organic carbon (SOC) was measured by titrimetry after oxidation with a mixture of H2SO4 and K2Cr2O7. Total N and P were determined using the Kjeldahl and molybdenum blue colorimetric methods, respectively. All of these methods have been described by Lu (2000).
Microbial biomass C (MBC) and microbial biomass N (MBN) were analyzed using the fumigation-extraction method; 10 g of fresh soil was fumigated with chloroform in the dark for 24 h, and then the fumigated and non-fumigated soils were extracted with 0.5 M K2SO4 and shaken at 200 rpm for 0.5 h. Soil extracts were filtered through a 0.45 µm Millipore filter, and the C and N in the extracts were determined using a multi C:N 3100 analyzer (Analytik Jena AG). The C and N contents in extracts of the non-fumigated soil were subtracted from the C and N extracted from the fumigated soil to give the C and N extracted from the soil microbial biomass. Values of 0.45 and 0.54 were used to calibrate the contents of MBC and MBN, respectively (Vance et al., 1987; Wu et al., 1990).
The activities of cellulose and N-acetyl-β-glucosaminidase (NAG) were measured using p-nitrophenyl-β-D-cellobioside and p-nitrophenyl-N-acetyl-β-D-glucosaminide as substrates, respectively. Fresh soil (1.0 g) was mixed with 2.5 mL of 0.2 M acetate buffer (pH 5.0) and 2.5 mL of 0.02 M substrates and then shaken at 200 rpm and 37 °C for 1 h. The reaction was stopped by adding 1 mL of 0.5 M CaCl2 and 4 mL of 0.1 M Tris buffer (pH 12.0). The mixture was suspended with a vortex, the supernatant was filtered, and the concentration of p-nitrophenol (PNP) was measured by colorimetry at 400 nm. The same procedure was followed for the controls, with the exception that the substrate was added after the incubation, and CaCl2 and Tris buffer were added (Dick, 2011; Geisseler and Horwath, 2009).
To estimate the greenhouse gas emission potential, we conducted a 60 d incubation experiment. Briefly, 20 g of fresh soil was placed in a 250 mL flask and then sealed with a gas-tight lid that had a rubber stopper in the middle. Gas samples (10 mL) were taken from the headspace of each flask at 1, 3, 7, 15, 30, and 60 d after sealing using a plastic syringe. The gas sample was immediately injected into a pre-evacuated 10 mL glass vial. Concentrations of methane (CH4), N2O, and carbon dioxide (CO2) were determined using a gas chromatograph (Agilent 7890) equipped with a flame ionization detector for CO2 and CH4 and a 63Ni electron capture detector for N2O. The gas standards were provided by the National Research Center for Certified Reference Materials, Beijing, China. The precision for greenhouse gas emission concentrations was ± 0.5 % based on repeated measurements of gas standards (Qiu et al., 2019). When the maize plants matured, all plants and grains were harvested from each plot, oven-dried at 60 °C for 48 h, and weighed. Straw aboveground biomass and crop yield were converted into weight per hectare.
We selected 15 soil properties to estimate cropland traits, i.e., the soil fertility index (SOC, total N, total P, MBC, and MBN), greenhouse gas emission amount (mainly CO2, N2O, and CH4), straw decomposition and C and N released, soil extracellular enzymes (cellulase and N-acetyl-D-glucosaminidase), and maize biomass (aboveground biomass and crop yield). Generally, SOC, total N, and total P are the major soil fertility factors and indicate the present nutrient status in croplands, which can be used to explain soil fertility conditions. Microbial biomass reflects ecosystem productivity. Greenhouse gas emissions are related to climate change, which can be regulated by fertilization regimes and soil microbial activities. Soil extracellular enzymes catalyze the decomposition of a range of organic polymers, resulting in C and N turnover. Maize biomass (such as aboveground biomass and crop yield) reflects soil productivity. As a whole, all of these variables contributed to the cropland functioning. To evaluate the functioning of the cropland ecosystem under different fertilization conditions, we calculated an integrative soil ecosystem multifunctionality index for further analysis. Notably, the opposite value was chosen for greenhouse gas emissions. Due to the lack of a specific definition of multifunctionality, we first calculated the Z scores of the 15 measured variables and obtained a multifunctionality value for each plot by averaging the Z scores of the 15 variables (Chen et al., 2019).
2.4 DNA extraction and quantification of general fungal ITS, bacterial 16S rRNA, and genes encoding cellulose-degrading enzymes
Total DNA was extracted from 0.5 g freeze-dried soil by using a FastDNA Spin Kit for Soil (MPbio, USA) according to the manufacturer's instructions and then dissolved in 50 µL of Tris-EDTA buffer. The quality of the DNA extraction was characterized by electrophoresis on 1 % (wt vol−1) agarose gels. The quantity and quality of DNA were checked using a NanoDrop spectrophotometer (NanoDrop, PEQLAB, Germany). The extracted DNA samples were stored at −80 °C before molecular analysis.
Bacterial and fungal abundances were determined to reveal the changes in microbial community compositions. The abundances of bacteria and bacteria fungi were measured according to modified procedures (Fierer and Jackson, 2005). We selected the primers 338F/518R (338F: CCTACGGGAGGCAGCAG; 518R: ATTACCGCGGCTGCTGG) and NSI1/58A2R (NSI1: GTAGTCATATGCTTGTCT; 58A2R: CATTCCCCGTTACCCGTT) for the qPCR assay. The thermal qPCR profiles for the bacteria and fungi were as follows: 95 °C, 2 min for DNA denaturation; 35 cycles (95 °C, 30 s; 60 °C, 30 s; 72 °C, 30 s; 80 °C 15 s) for DNA annealing; 81 °C, 10 s for DNA extension; 95 °C, 10 min for DNA denaturation; 40 cycles (95 °C, 15 s; 52 °C, 30 s; 72 °C, 30 s; 79 °C, 30 s) for DNA annealing; and 81 °C, 10 s for DNA extension, respectively. The initial concentrations of the two plasmids used as the standards for the bacterial and fungal abundance analyses were 1.22×1010 and 9.05×109, respectively.
The fungal cbhI gene and bacterial GH48 gene were selected as functional biomarkers of cellulolytic fungi and bacteria, respectively. The primers GH48 F8/GH48 R5 (GH48_F8: 5′-GCCADGHTBGGCG ACTACCT-3′; GH48_R5: 5′-CGCCCCABGMSWWGTACCA-3′) and cbhI F/cbhI R (cbhI F: ACCAAYTGCTAYACIRGYAA; cbhI R: GCYTCCCAIATRTCCATC) were used for the qPCR assay. The abundances of bacterial GH48 and fungal cbhI genes ere quantified according to modified procedures (Zhang et al., 2017). The thermal profiles of qPCR for the target genes of GH48 and cbhI were as follows: 95 °C, 5 min for DNA denaturation; 40 × (94 °C for 30 s, 60 °C for 45 s, and 72 °C for 90 s) for DNA annealing; 84 °C, 10 s for DNA extension; 94 °C, 4 min for DNA denaturation; 40 × (94 °C for 45 s, 50 °C for 30 s, and 72 °C for 60 s) for DNA annealing; and 81 °C, 10 s for DNA extension. The initial concentrations of the two plasmids as the standards for the bacterial GH48 and fungal cbhI gene abundance analysis corresponded to 1.85 × 1011 and 2.65 × 1010 copies g−1 dry soil, respectively; qPCR was performed in triplicate, and amplification efficiencies higher than 95 % were obtained with r2 values > 0.99.
2.5 Bacterial 16S rRNA genes and fungal ITS amplification and sequencing
High-throughput sequencing was performed with the Illumina MiSeq sequencing platform (Illumina Inc.). Both the forward and reverse primers were tagged with an adapter and linker sequence, and 8 bp barcode oligonucleotides were added to distinguish the amplicons from different soil samples.
The primers 515F (5′-GTGCCAGCMGCCGCGGTAA-3′) and 907R (5′-CCGTCAATTCMTTTRAGTTT-3′) were chosen to amplify the 16S rRNA genes in the V4–V5 hypervariable region. PCR was conducted in a 50 µL reaction mixture containing 27 µL of ddH2O, 2 µL (5 µM) of each forward or reverse primer, 2.5 µL (10 ng) of template DNA, 5 µL (2.5 mM) of deoxynucleoside triphosphates, 10 µL of 5× FastPfu buffer, 0.5 µL of bovine serum albumin, and 1 µL of TransStart FastPfu polymerase (TransGen, Beijing, China). The PCR conditions were 94 °C for 5 min; 30 cycles of 94 °C for 30 s, 52 °C for 30 s, and 72 °C for 30 s of extension; and then 72 °C for 10 min (Caporaso et al., 2010).
The fungal ITS1 region was amplified using the primer pair ITS1F (CTTGGTCATTTAGAGGAAGTAA)–ITS2 (GCTGCGTTCTTCATCGATGC) (Ghannoum et al., 2010). The 50 µL reaction mixture of each reaction mix consisted of 1 µL (30 ng) of DNA, 4 µL (1 µM) of each forward or reverse primer, 25 µL of PCR Master Mix, and 16 µL of ddH2O. PCR amplification was conducted at 98 °C for 3 min, followed by 30 cycles (98 °C for 45 s, 55 °C for 45 s, and 72 °C for 45 s), with a final extension at 72 °C for 7 min (Ghannoum et al., 2010). All amplicons were cleaned and pooled in equimolar concentrations in a single tube, after which they were subjected to library preparation, cluster generation, and 250 bp paired-end sequencing on an Illumina MiSeq platform (Illumina Inc., San Diego, CA, USA).
The raw sequence data were processed using the Qualitative Insights into Microbial Ecology (QIIME) pipeline (Caporaso et al., 2010). Sequences that fully matched the barcodes were selected and distributed into separate files for the bacterial 16S rRNA and fungal ITS genes. Poor-quality sequences with lengths less than 200 bp (for fungal ITS) and 500 bp (for bacterial 16S) and quality scores less than 20 were discarded, and the chimeras were removed using the UCHIME algorithm (Edgar et al., 2010). The remaining sequences were assigned to operational taxonomic units (OTUs) with a 97 % similarity threshold using UCLUST (Edgar et al., 2010). Alpha diversity and Bray–Curtis distances for principal coordinate analysis of the soil microbial community were calculated after rarefying all samples to the same sequencing depth.
2.6 Statistical analysis
The soil ecosystem multifunctionality index, crop yields, microbial traits, and other relevant soil variables among treatments were subjected to a chi-square test for independence of variance. Significant differences were determined by one-way analysis of variance (ANOVA) based on the post hoc Tukey test at the 5 % level. Prior to ANOVA, the normality and homogeneity of variances were tested by the Kolmogorov–Smirnov test and Levene's test, respectively. If normality was not met, log or square-root transformation was implemented. One-way ANOVA was performed using SPSS 21.0 (SPSS Inc., Chicago, IL, USA).
Nonmetric multidimensional scaling (NMDS) analysis was used to describe and evaluate the microbial community composition. The NMDS was performed in the Vegan package of R (4.0.2). Analysis of similarities (ANOSIM) was used to examine the significant differences in microbial community structure under different fertilizations. To describe the complex co-occurrence patterns in various organisms, we constructed co-occurrence networks. We focused on the abundant microbial phylotypes (with average relative abundance > 0.01 % for bacteria and fungi) for network construction. Nodes with Pearson correlations greater than 0.70 and P<0.05 were retained. Network visualization between microbial taxa and ecological clusters of microbial phylotypes was conducted and identified by Gephi software. To obtain the keystone species of each network, a Zi–Pi (ZP) plot series was constructed to determine the role of each OTU. According to Deng et al. (2012), the plot includes (a) peripheral nodes (Z≤0.25, P≤0.62), (b) module hubs (Z>0.25, P≤0.62), (c) connectors (Z≤0.25, P>0.62), and (d) network hubs (Z>0.25, P>0.62). From an ecological perspective, OTUs in module hubs, connectors, and network hubs may be regarded to be the microbial keystone taxa of the network systems (Deng et al., 2015).
To construct the relationship between fertilization, soil function, and microbial traits, two heat maps were constructed in this study (Origin 2022). The first heat map was constructed to reveal the associations between cropland properties with microbial module communities, and another heat map was constructed to reveal the associations between microbial traits and fertilizers, soil properties, greenhouse emissions, and ecosystem multifunctionality. The random forest algorithm was performed in the R package (4.0.2) randomForest to estimate the importance predictors of soil properties and microbial traits with regard to ecosystem multifunctionality.
3.1 Cropland properties
Data collection after a continuous 4-year in situ field experiment conducted under different N input levels revealed changes in cropland traits (Fig. 1). In terms of soil fertility, compared with the N limitation treatments (PK and 0.5N + PK), the SOC and total P contents were increased significantly by the N + PK and 0.75N + PK treatments (Fig. 1a, c) (P<0.05), while there were no significant changes in the total N content (Fig. 1b). After straw decomposition (Fig. 1d), the amounts of straw C (Fig. 1e) and N (Fig. 1f) released showed different responses to varying N fertilizer input levels. Generally, N-rich treatments (N + PK and 0.75N + PK) significantly increased the straw decomposition rate and achieved higher amounts of straw C and N release than the N limitation treatments (P<0.05). However, there was no significant difference between N + PK and 0.75N + PK. Microbial biomass C and N contents, as well as associated enzyme activity, were changed after different N fertilizer application rates (Fig. 1g, h, i, and j). The MBC (Fig. 1g) and MBN (Fig. 1h) contents were significantly higher in the N-rich treatments than in the other treatments. However, the highest cellulase activity was observed in the 0.75N + PK treatment, which was significantly higher than that in the other treatments (Fig. 1i) (P<0.05), and the N-acetyl-D-glucosaminidase activity decreased with the reduction in N application (Fig. 1j).
For greenhouse gas emissions, with the decrease in N fertilizer application levels, CO2 and N2O emissions gradually decreased (Fig. 1k, l). No significant difference was observed in CH4 emissions under the different fertilization treatments (Fig. 1m). In addition, the N fertilizer levels also had a strong influence on maize yields and aboveground biomass (Fig. 1n, o). The 0.75N + PK treatment achieved the highest multifunctionality index (0.61), followed by N + PK (0.32), 0.5N + PK (−0.34), and PK (−0.59) (Fig. 1p).
However, although the 0.75N + PK treatment increased the straw N release amount and may meet the requirements for plant growth, the total N input was still dominated by inorganic N input (Fig. S1). Therefore, the N released from the straw cannot offset the deficiency of the N fertilizer. Additionally, contrasting N fertilizer input levels significantly changed the stoichiometry of C, N, and P (Fig. S2). Notably, the 0.75N + PK treatment significantly increased the C:N ratio compared with the 0.5N + PK and PK treatments (P<0.05). The lowest C:N ratio was shown for the 0.5N + PK treatment (Fig. S2a). The N:P and C:P ratios showed no significant difference regardless of nutrient excess or limitation (Fig. S2b and c).
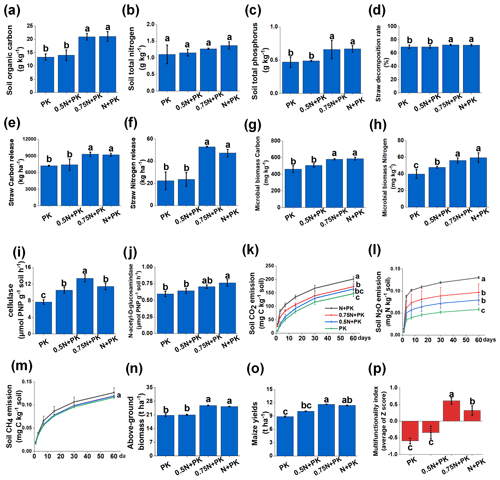
Figure 1The 15 cropland variables and multifunctionality index under different N input levels after straw return. Abbreviations: N + PK refers to the straw return plus regular inorganic N–P–K fertilizers, 0.75N + PK refers to the straw return plus regular inorganic P–K with 25 % N fertilizer reduction, 0.5N + PK refers to the straw return plus regular inorganic P–K with 50 % N fertilizer reduction, and PK refers to straw return plus regular inorganic P–K without N fertilizer.
3.2 Abundances of bacteria, fungi, and genes encoding cellulose-degrading enzymes
N fertilizer input levels had marked impacts on the abundances of fungi and bacteria (Table S3). The highest fungal abundance was observed in the 0.75N + PK treatment, which was significantly higher than that in the other treatments (P<0.05). The N + PK treatment significantly increased bacterial abundance compared with the PK treatment (P<0.05), while there were no obvious differences among the N + PK, 0.75N + PK, and PK treatments. The ratios of fungi to bacteria also showed contrasting responses to N fertilization (Table S3). The 0.75N + PK treatment significantly increased the ratio of fungi to bacteria compared with the other treatments (P<0.05), and the lowest ratio of fungi to bacteria was found in the PK treatment.
Table 1The abundances of genes encoding cellulose-degrading enzymes across different N fertilizer level treatments after straw return.
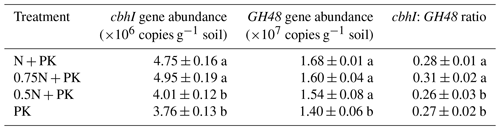
The results show means ± standard deviations (n=3). Different lowercase letters after values indicate significant differences between each treatment, P<0.05. N + PK refers to the straw return plus regular inorganic N–P–K fertilizers, 0.75N + PK refers to the straw return plus regular inorganic P–K with 25 % N fertilizer reduction, 0.5N + PK refers to the straw return plus regular inorganic P–K with 50 % N fertilizer reduction, and PK refers to the straw return plus regular inorganic P–K without N fertilizer.
N fertilizer input levels led to changes in the expression levels of genes encoding cellulose-degrading enzymes (Table 1). The N-rich treatments achieved higher fungal cbhI and bacterial GH48 gene abundance than the N limitation treatments. In contrast, the highest cbhI gene abundance was shown in the 0.75N + PK treatment, while the highest GH48 gene abundance was shown in the N + PK treatment. Compared with the PK treatment, the ratio of the fungal cbhI gene to the bacterial GH48 gene increased significantly under the 0.75N + PK treatment (P<0.05).
3.3 Co-occurrence network analysis of the microbial community
Regarding fungal alpha diversities, there were no significant differences in the Chao1 index across treatments. The N + PK treatment significantly increased fungal richness compared with the PK treatment (P<0.05) (Table S4). In addition, the PK treatment resulted in lower bacterial richness than the other treatments (P<0.05). No significant difference was observed in the bacterial Chao1 index across treatments (Table S4). NMDS plots showed that diverse N input levels significantly changed the fungal (Fig. S3a) and bacterial communities (Fig. S3b) (P<0.05).
We further conducted network analysis to identify co-occurrence patterns between specific microbial taxa (Fig. 2). The co-occurrence network was aggregated into smaller coherent modules that were examined to determine important module–trait relationships. The present network comprised 1963 nodes (composed of 1520 bacterial taxa and 443 fungal taxa) and 62 206 edges with 52.49 % positive associations (Fig. 2a). The results showed four dominant ecological modules (1–4) that strongly co-occurred within the multi-trophic network, which contributed 86.10 % of the whole network. Among the four modules, bacteria accounted for the highest proportion in each module, contributing more than 70 % of the total (Fig. 2b). The percentage of edges linking bacteria to bacteria (B–B) was higher than that of edges linking fungi to fungi (F–F) and bacteria to fungi (B–F). The highest proportion of B–B (80.32 %) was found in module 3, while the highest proportion of B–F (32.66 %) and F–F (6.00 %) was found in module 4 (Fig. 2c).
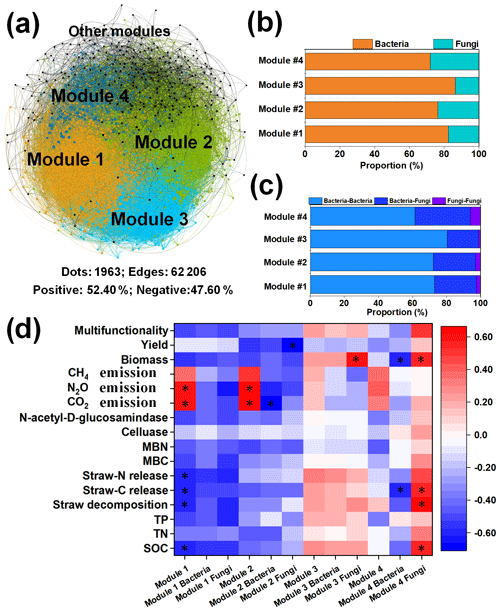
Figure 2The relationships of microbial module communities with cropland traits. Multi-trophic network including multiple ecological modules. The colors of the nodes represent different ecological modules (a). OTU number proportions of bacteria and fungi (b). The proportions of the edges linking bacteria to bacteria (B–B), bacteria to fungi (B–F), and fungi to fungi (F–F) in the major ecological modules (c). Links between the specific module communities with cropland traits (d). * Indicates significance at P<0.05. Abbreviations: SOC refers to soil organic carbon, C:N refers to the ratio of the SOC content to the total N content, and N:P refers to the ratio of the total N content to the total P content.
Individual nodes represented different roles in the microbial network based on the intra-module connectivity Zi and the intermodule connectivity Pi. ZP plots were constructed to identify the topological roles of each node in the network (Fig. 3a). As shown in Fig. 3b, 113 microbial taxa (81 bacterial species and 32 fungal species) were regarded to be connectors, and 43 microbial taxa (39 bacterial species and 4 fungal species) were regarded to be module hubs. Specifically, module 2 (54) contained the most keystone taxa, followed by module 1 (38) and then module 3 (32).
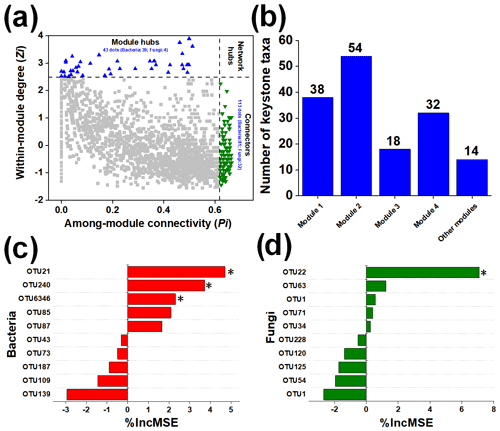
Figure 3The topological roles of microbial taxa and their effect on the soil multifunctionality index. The topological role of each OTU was determined according to the scatterplot of within-module connectivity (Z) and among-module connectivity (P) (a). The distribution of keystone taxa in each ecological module (b). Contribution of bacterial (c) and fungal OTUs (d) to the soil multifunctionality index. *, , and indicate significance at P<0.05, 0.01, and 0.001, respectively.
3.4 Linkage between microbial traits and soil ecosystem multifunctionality
The heat map showed the close correlations of N input (fertilizer and straw return) with soil stoichiometry and microbial traits (Fig. 4). Overall, the N input level, straw biomass, and C:N ratio up-regulated the abundance of genes encoding cellulose-degrading enzymes. In addition, N input was positively correlated with bacterial abundance, while a significant correlation was observed between straw biomass and the N input level. The random forest model was also used to identify abiotic and biotic attributes correlated with soil ecosystem multifunctionality (Fig. 5). The model explained 83.89 % of the variance in ecosystem multifunctionality. The results indicated that the N input level, straw biomass, and soil C:N ratio were the most prominent abiotic factors correlated to the ecosystem multifunctionality index, while some biotic factors, such as the abundance of genes encoding cellulose-degrading enzymes, were significantly correlated to the ecosystem multifunctionality index.
Moreover, to clarify the potential main specific drivers of cropland traits, correlations between the microbial physiological traits and soil properties were determined to illuminate the role of the microbial community in soil ecosystem multifunctionality (Fig. 2d). The results indicated that the particular microbial module community was significantly correlated with cropland traits. The communities of modules 1 and 2 and the fungal community in module 4 showed potential in cropland traits (Fig. 2d). Specifically, significant correlations were observed between the SOC content, straw decomposition, straw C:N release, CO2/N2O emissions, and the module-1 community; the module-2 community was positively correlated with greenhouse gas emissions (except for CH4), and the fungal community in module 4 was positively correlated with the SOC content, straw decomposition, straw C:N release, and straw biomass. Furthermore, the bacterial and fungal communities belonging to module 2 and the fungal community belonging to module 3 were significantly correlated with CO2 emission, maize yield, and straw biomass.
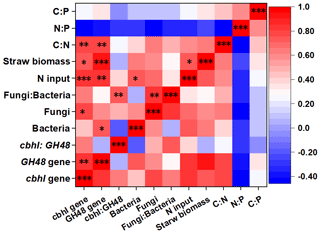
Figure 4Heat map revealing the correlation coefficients between microbial traits with fertilization and soil stoichiometry. *, , and indicate significance at P<0.05, 0.01, and 0.001, respectively. Abbreviations: C:N refers to the ratio of the SOC content to the total N content, and N:P refers to the ratio of the total N content to the total P content.
At the scale of microbial species, we selected the 20 keystone taxa (10 bacterial and 10 fungal taxa) with the highest relative abundance for further analysis. The random forest models indicated that the specific keystone taxa strongly influenced soil ecosystem multifunctionality (Fig. 3c and d). Bacterial Terrimonas (in module 1), Myxococcales (in module 2), and Terrimonas (in module 3) were highlighted as essential predictors of soil ecosystem multifunctionality, and fungal Lasiosphaeriaceae (module 3) was also found to be an important variable for predicting its changes. Subsequently, the relative abundances of selected keystone taxa were different across different N fertilizer level treatments after straw return (Table S5). The relative abundances of fungal OTU22 and bacterial OTU21 were higher in the N-rich treatments than in the N limitation treatments. Moreover, compared with the N + PK treatment, the 0.75N + PK treatment increased the relative abundances of fungal OTU22 by 38.20 % and of bacterial OTU21 by 40.63 %.
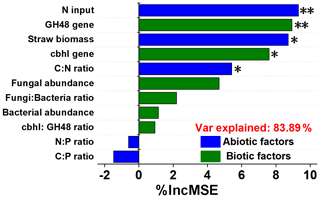
Figure 5Contribution of abiotic and biotic variables to the soil multifunctionality index. *, , and indicate significance at P<0.05, 0.01, and 0.001, respectively. Abbreviations: C:N refers to the ratio of the SOC content to the total N content, and N:P refers to the ratio of the total N content to the total P content.
4.1 Effect of N fertilizer reduction on cropland traits after straw return
Soil fertility, straw decomposition, C and N release amounts, and crop productivity were mostly higher under 0.75N + PK and N + PK than under other treatments, implying that better soil multifunctionality was achieved. Moreover, N + PK increased greenhouse gas emissions (Fig. 1). Higher microbial biomass C and N, as well as relevant enzyme activities, were also observed under N-rich treatments, indicating the strong positive impact of abundant N fertilizer application (Fig. 1g, h, i, j). It was reported that straw return with N fertilizer application can stimulate microbial activity and promote biomass accumulation (Treseder, 2008). The substantially increased straw decomposition and straw C and N release under N-rich treatments may be primarily attributed to the activation of microbial activity (Fig. 1d, e, f), which is consistent with previous research (Ramirez et al., 2012). Our results indicated that 0.75N + PK maintained soil fertility index net primary production compared to N + PK. This study demonstrates that 0.75N + PK has similar effects on cropland traits as N + PK. Therefore, it can be concluded that 0.75N + PK is a more efficient and effective option for improving soil functions. Moreover, 0.75N + PK may enhance N fertilizer use efficiency and stimulate microbial functioning by modulating the stoichiometry of C, N, and P in the soil, which promotes soil fertility and crop yield (Liu et al., 2010). Reducing the amount of N fertilizer by more than 50 % led to insufficient N inputs to meet the needs of both crops and microbes, resulting in a decline in soil health (Williams et al., 2013). Recent studies have also proven that rational N inputs can stimulate microbial ex vivo production of extracellular enzymes to accelerate straw decomposition and nutrient transformation (Chen et al., 2016). Moreover, it is well known that fungi have high nutrient utilization efficiency; thus, more straw-derived C and N would be stored in soil under N-rich treatments than under N-limited treatments (Hou et al., 2020). Rational N availability is also the premise of straw decomposition and SOM formation due to the microbial “stoichiometry decomposition” theory, while the “N-mining” theory in N limitation treatments reveals that oligotrophic species (such as K strategists) degrade native SOM because of the lack of N fertilizer inputs (Chen et al., 2014). In this study, a 25 % reduction in N fertilizer application may be the threshold value for the N mining and the stoichiometry decomposition. Finally, the increases in SOC, total N and P contents, and straw C and N release, as well as in the microbial biomass and functioning, are commonly attributed to high aboveground biomass and maize yields (Fig. 1n, o), which are favorable from the viewpoint of cropland traits.
However, the overuse of N inputs also causes more greenhouse gas emissions (Tang et al., 2019). In the present study, greenhouse gas emissions were quantified to evaluate the ecosystem degeneration under different N fertilizer input levels, which means the larger the value, the lower the soil ecosystem multifunctionality (Fig. 1k, l, m). Straw return with N fertilizer addition might be the crucial driver of CO2 and N2O emissions from agroecosystems and has been widely studied in previous literature (Gregorich et al., 2005). CO2 and N2O emissions increased significantly compared with those under the PK treatment, likely due to the stimulation of the activity of copiotrophs when sufficient C and N substrates were provided. For example, Dieleman et al. (2010) implied, through meta-analysis and field experiments, that there is an increase in CO2 and N2O, respectively, as the amount of N input increases. Qiu et al. (2019) indicated that the emission of CO2 enhanced root and mycorrhizal N uptake and increased N2O emissions, which was related to the changes in the soil denitrifier community composition in favor of N2O-producing taxa (nirK type or nirS type). In addition, there was no difference in CH4 emissions among treatments, although contradictory results have been widely reported in previous literature (Tang et al., 2019). Mapanda et al. (2011) and Liu et al. (2012) indicated that the emission of CH4 depended highly on the soil water content in maize crops, which is in line with our results. To summarize, previous research clearly demonstrated a positive correlation between CO2 and N2O emissions with N input. So, this study unequivocally showed that N + PK emits more greenhouse gases than 0.75N + PK.
In summary, compared with the N + PK treatment, the 0.75N + PK treatment supported multiple soil functions, including the promotion of soil fertility, straw nutrient release and microbial activity, and alleviating greenhouse gas emissions (Fig. 1p). Therefore, a reduction of 25 % in chemical N fertilizer input with straw return may be the appropriate regime to promote soil functions in meadow soils in the Northeast China Plain.
4.2 Responses of the microbial abundance and functioning to straw return with N fertilizer reduction
Fungal and bacterial abundances, as well as the ratio of fungi to bacteria, were sensitive to the changes in the N fertilizer input levels (Table S3 and Fig. 2). Straw addition with N fertilizer input supplied enough C and N for microbial metabolism, thus promoting microbial proliferation (Chen et al., 2016). Generally, bacterial abundance decreased with reduced N fertilizer input. This is mainly because bacteria are more sensitive to N availability than fungi, which is in line with a previous study (Ramirez et al., 2020). It is worth noting that a 25 % reduction in N fertilizer significantly increased fungal abundance compared with regular N inputs. This result might be attributed to the negative effect of excess N fertilizer (Wan et al., 2015). Moreover, Ning et al. (2020) demonstrated that the C:N ratio was the pivotal factor in fungal community compositions after performing seven long-term field experiments under different fertilization conditions across China and reported a significant positive correlation between them. It is well known that fungi have a strong C utilization efficiency compared to bacteria (Duan et al., 2021). Therefore up-regulation of fungal abundance and lowering the ratio of bacteria to fungi are crucial for straw degradation and SOC accumulation. Previous studies have shown that the ratio of soil microbial biomass was stable at (Cleveland et al., 2007) and that the fungal biomass C:N ratio was higher than this ratio (nearly 15.7:1) (Zhang et al., 2017). Generally, Bahram et al. (2018) concluded that a higher C:N ratio may promote fungal abundance and decrease the bacteria : fungi ratio. Excessive N fertilizer input may reduce the soil C:N ratio, while little N fertilizer input cannot meet the growth requirements of crops and microorganisms (Ning et al., 2020). Therefore, appropriate enhancement of the soil C:N ratio can increase the ratio of fungi to bacteria, stimulate fungal function, and promote straw degradation and SOC accumulation. Therefore, the 0.75N + PK treatment with a higher C:N ratio (16.47) may facilitate the proliferation of microorganisms and promote an increase in microbial abundance.
Subsequently, our results showed that N-rich treatments resulted in higher microbial cellulose-degrading gene abundances than the PK treatment (Table 1), which demonstrated the irreplaceable role of N inputs in straw degradation (Zhang et al., 2017). Additionally, compared with bacterial GH48 gene abundance, the increase in fungal cbhI gene abundance required adequate N fertilizer inputs and was regulated by the soil C:N ratio, which suggests that rational N fertilizer inputs could promote fungal functioning for further degradation of recalcitrant straw components (Hou et al., 2020). Therefore, the ratio of cbhI gene abundance to GH48 gene abundance was higher under 0.75N + PK than under the N limitation treatments since the increased expression of a fungal cellulose-degrading gene implies more straw C and N release.
Our results indicated that 75 %–100 % N fertilizer could up-regulate fungal and cbhI gene abundances, which may lead to straw decomposition and SOC accumulation. It is therefore necessary to further explore the potential associations between microbial traits and soil functions under diverse N fertilizer input levels.
4.3 Linkages of cropland properties with microbial traits
To clarify the effect of abiotic and biotic factors on soil cropland properties, we quantified the contributions of abiotic and biotic attributes to the ecosystem multifunctionality index across N input treatments (Figs. 4 and 5). Biotic factors, such as cbhI and GH48 gene abundances, as well as abiotic factors, including the C:N ratio, straw biomass, and N input level, are also pivotal regulators of ecosystem multifunctionality (Fig. 5). In general, promoting the rapid degradation of straw is an important way to convert straw C into SOM, thus improving soil fertility, aboveground biomass, and crop yield. In addition, fungi have a higher C utilization efficiency than bacteria; thus, a high fungal cbhI gene abundance may achieve better soil multifunctionality (Hou et al., 2020). For abiotic factors, the soil C:N ratio, straw biomass, and N fertilizer input are always regarded to be the main indicators of soil fertility and health, likely due to their providing various nutrient accessibilities and influencing the microbial community composition (Ning et al., 2020).
Numerous studies have shown that core microbiota play a vital role in maintaining the stability of soil microbial functioning and the complexity of microbial networks and then promoting soil nutrient cycling and other soil functions (Ghannoum et al., 2015), and keystone species may show great explanatory power in terms of specific network (or module) structures and functioning (Chen et al., 2019b). In the present study, Terrimonas (bacterial species in module 1) and Lasiosphaeriaceae (fungal species in module 3) were detected as the keystone taxa in influencing the soil multifunctionality of the co-occurrence network (Table S5). A previous study demonstrated that straw addition significantly increased the relative abundance of Lasiosphaeriaceae, which implied straw decomposition ability (Song et al., 2020). Afterwards, Lasiosphaeriaceae was proven to promote straw-derived C and N accumulation by secreting multiple extracellular enzymes (Guo et al., 2022). Meanwhile, Sun et al. (2023) revealed that Lasiosphaeriaceae abundance was regulated by the soil C:N ratio, especially changes in mineral N. Therefore, Lasiosphaeriaceae can effectively promote straw degradation and straw C and N release while driving the function and community of module 1, which is consistent with our results (Fig. 2d). However, relatively few studies have focused on the function of Terrimonas, and so this study focused on Chitinophagaceae. As reported in the previous literature, straw return was the main method to increase Chitinophagaceae abundance (Li et al., 2021). Furthermore, Chitinophagaceae was indicated to have a strong ability to accumulate soil C and N and degrade cellulose (Zhong et al., 2022), facilitating production improvement by regulating the module-3 community and functioning, which is in line with our results (Fig. 2d).
Overall, straw return with sufficient N fertilizer application can increase the C:N ratio and stimulate microbial traits, which ultimately achieves soil ecosystem multifunctionality (Fig. 6). Straw return without enough N supply cannot support soil functions due to the decomposition of native SOM and the out-of-balance microbial community composition according to the N-mining theory (Chen et al., 2014); straw return with sufficient N application (N + PK and 0.75N + PK) can promote soil fertility, straw release, microbial activity, and crop productivity, which can be explained by the stoichiometry decomposition theory (Chen et al., 2014). Meanwhile, N + PK also caused more serious ecosystem degeneration, such as greenhouse gas emissions, than the 0.75N + PK treatment. Moreover, compared with the N + PK treatment, the 0.75N + PK treatment increased the soil C:N ratio and stimulated microbial module-1 and module-3 community functioning, cbhI gene abundances, and keystone taxa abundances, which were significantly positively correlated with soil ecosystem multifunctionality. On the other hand, Lasiosphaeriaceae-driven module-1 and Terrimonas-driven module-3 communities may be involved in maintaining soil ecosystem multifunctionality. Our study provides evidence that a 25 % reduction in chemical N fertilizer after straw return was the optimal agronomic measure for soil functions in meadow soil in the Northeast China Plain.
Straw return combined with different chemical N fertilizer application rates significantly changed cropland traits. Collectively, our work indicates that, compared with the N + PK treatment, straw return with a 25 % reduction in chemical N fertilizer has the potential to improve soil functions by maintaining soil fertility, productivity, microbial biomass, and functioning, promoting straw decomposition and C and N release and alleviating greenhouse gas emissions. The 0.75N + PK treatment achieved higher soil ecosystem multifunctionality than all other treatments. In addition, the N input level, straw biomass, and soil C:N ratio can up-regulate the abundances of the cbhI and GH48 genes, which may together achieve soil ecosystem multifunctionality.
Furthermore, the changes in multiple cropland traits were strongly associated with microbial module communities and keystone taxa. The relationships between cropland properties and microbial traits were examined here to confirm that the Lasiosphaeriaceae driving the functioning and structure of the module-1 community leads to the promotion of straw degradation and straw C and N release, while Terrimonas driving the functioning and structure of the module-3 community probably contributes to production improvement under the 0.75N + PK treatment. Therefore, a 25 % reduction in chemical N fertilizer with straw return might be a win–win strategy that not only produces considerable ecological benefits for the pedosphere and atmosphere but also reduces fertilizer expenditures in meadow soil in the Northeast China Plain.
Some or all of the data, models, or code generated or used during the study are available from the corresponding author upon request.
The supplement related to this article is available online at: https://doi.org/10.5194/soil-10-779-2024-supplement.
YD, LW, and XM designed the experiment; YD, MC, ZN, WZ, and YW performed the measurements; YD, YW, MZ, and JL analyzed the data; YD and MC wrote the paper draft; YL, JL, and LW reviewed and edited the paper.
The contact author has declared that none of the authors has any competing interests.
Publisher's note: Copernicus Publications remains neutral with regard to jurisdictional claims made in the text, published maps, institutional affiliations, or any other geographical representation in this paper. While Copernicus Publications makes every effort to include appropriate place names, the final responsibility lies with the authors.
We thank all our lab colleagues for their assistance with the soil sampling and analyses.
This research has been supported by the National Key Research and Development Program of China (grant no. 2023YFD1901005), the Anhui Post- doctoral Science Foundation (grant no. 2022B638), the Special Project of Zhongke Bengbu Technology Transfer Center (grant no. ZKBB202103), the China Postdoctoral Science Foundation (grant no. 2023M733542), the Special Research Assistant Project of the Chinese Academy of Sciences (grant no. 2023000140), and the Chinese Academy of Sciences (CASHIPS) Director's Fund (grant no. YZJJ2023QN37).
This paper was edited by Kate Buckeridge and reviewed by Yong Li, Guilherme Lucio Martins, and one anonymous referee.
Allan, E., Manning, P., Alt, F., Binkenstein, J., Blaser, S., Bluethgen, N., Bohm, S., Grassein, F., Holzel, N., Klaus, V. H., Kleinebecker, T., Morris, E. K., Oelmann, Y., Prati, D., Renner, S. C., Rillig, M. C., Schaefer, M., Schloter, M., Schmitt, B., Schoning, I., Schrumpf, M., Solly, E., Sorkau, E., Steckel, J., Steffen-Dewenter, I., Stempfhuber, B., Tschapka, M., Weiner, C. N., Weisser, W. W., Werner, M., Westphal, C., Wilcke, W., and Fischer, M.: Land use intensification alters ecosystem multifunctionality via loss of biodiversity and changes to functional composition, Ecol. Lett., 18, 834–843, https://doi.org/10.1111/ele.12469, 2015.
Bahram, M., Hildebrand, F., Forslund, S.K., Jennifer L. A., Nadejda, A. S., Nadejda, A. S., Johan, B., Sten, A., Luis, P. C., Helery. H., Jaime. H., Marnix, H. M., Mia, R. M., Sunil, M., Pål, A. O., Mari, P., Sergei, P., Shinichi, S., Martin, R., Leho, T., and Peer, B.: Structure and function of the global topsoil microbiome, Nature, 560, 233–237, https://doi.org/10.1038/s41586-018-0386-6, 2018.
Bao, Y. Y., Dolfing, J., Guo, Z. Y., Chen, R. R., Wu, M., Li, Z. P., Lin, X. G., and Feng, Y. Z.: Important ecophysiological roles of non-dominant Actinobacteria in plant residue decomposition, especially in less fertile soils, Microbiome, 9, 84, https://doi.org/10.1186/s40168-021-01032-x, 2021.
Bradford, M. A., Wood, S. A., Bardgett, R. D., Black, H. I. J., Bonkowski, M., Eggers, T., Grayston, S. J., Kandeler, E., Manning, P., Setala, H., and Jones, T. H.: Discontinuity in the responses of ecosystem processes and multifunctionality to altered soil community composition, P. Natl. Acad. Sci. USA, 111, 14478–14483, https://doi.org/10.1073/pnas.1413707111, 2014.
Caporaso, J. G., Lauber, C. L., Walters, W. A., Berg-Lyons, D., Huntley, J., Fierer, N., Owens, S. M., Betley, J., Fraser, L., Bauer, M., Gormley, N., Gilbert, J. A., Smith, G., and Knight, R.: Ultra-high-throughput microbial community analysis on the Illumina HiSeq and MiSeq platforms, Isme J., 6, 1621–1624, https://doi.org/10.1038/ismej.2012.8, 2012.
Chen, L. J., Jiang, Y. J., Liang, C., Luo, Y., Xu, Q. S., Han, C., Zhao, Q. G., and Sun, B.: Competitive interaction with keystone taxa induced negative priming under biochar amendments, Microbiome, 7, 77, https://doi.org/10.1186/s40168-019-0693-7, 2019a.
Chen, L., Redmile-Gordon, M., Li, J. W., Zhang, J. B., Xin, X. L., Zhang, C. Z., Ma, D. H., and Zhou, Y. F.: Linking cropland ecosystem services to microbiome taxonomic composition and functional composition in a sandy loam soil with 28-year organic and inorganic fertilizer regimes, Appl. Soil Ecol., 139, 1–9, https://doi.org/10.1016/j.apsoil.2019.03.011, 2019b.
Chen, R. R., Senbayram, M., Blagodatsky, S., Myachina, O., Dittert, K., and Lin, X. G., Blagodatskaya, E., and Kuzyakov, Y.: Soil C and N availability determine the priming effect: microbial N mining and stoichiometric decomposition theories, Glob. Change Biol., 20, 2356–2367, https://doi.org/10.1111/gcb.12475, 2014.
Chen, Y. L., Chen, L. Y., Peng, Y. F., Ding, J. Z., Li, F., Yang, G. B., Kou, D., Liu, L., Fang, K., Zhang, B. B., Wang, J., and Yang, Y. H.: Linking microbial stoichiometry to microbial community and abiotic factors along a 3500-km grassland transect on the Tibetan Plateau, Global Ecol. Biogeogr., 25, 1416–1427, https://doi.org/10.1111/geb.12500, 2016.
Cleveland, C. C. and Liptzin, D.: stoichiometry in soil: is there a “Redfield ratio” for the microbial biomass?, Biogeochemistry, 85, 235–252, https://doi.org/10.1007/s10533-007-9132-0, 2007.
de Bello, F., Lavorel, S., Diaz, S., Harrington, R., Cornelissen, J. H. C., Bardgett, R. D., Berg, M. P., Cipriotti, P., Feld, C. K., Hering, D., da Silva, P. M., Potts, S. G., Sandin, L., Sousa, J. P., Storkey, J., Wardle, D. A., and Harrison, P. A.: Towards an assessment of multiple ecosystem processes and services via functional traits, Biodivers. Conserv., 19, 2873–2893, https://doi.org/10.1007/s10531-010-9850-9, 2010.
Deng, Y., Jiang, Y.-H., Yang, Y., He, Z., Luo, F., and Zhou, J.: Molecular ecological network analyses, Bmc Bioinformatics, 13, 113, https://doi.org/10.1186/1471-2105-13-113, 2015.
Dieleman, W. I. J., Luyssaert, S., Rey, A., De Angelis, P., Barton, C. V. M., Broadmeadow, M. S. J., Broadmeadow, S. B., Chigwerewe, K. S., Crookshanks, M., Dufrene, E., Jarvis, P. G., Kasurinen, A., Kellomaki, S., Le Dantec, V., Liberloo, M., Marek, M., Medlyn, B., Pokorny, R., Scarascia-Mugnozza, G., Temperton, V. M., Tingey, D., Urban, O., Ceulemans, R., and Janssens, I. A.: Soil N modulates soil C cycling in CO2-fumigated tree stands: a meta-analysis, Plant Cell Environ., 33, 2001–2011, https://doi.org/10.1111/j.1365-3040.2010.02201.x, 2010.
Dick, R. P.: Methods of Soil Enzymology, Soil Science Society of America, Madison, 163–168, https://doi.org/10.2136/sssabookser9, 2011.
Dominati, E., Patterson, M., and Mackay, A.: A framework for classifying and quantifying the natural capital and ecosystem services of soils, Ecol. Econ., 69, 1858–1868, https://doi.org/10.1016/j.ecolecon.2010.05.002, 2010.
Duan, Y., Chen, L., Li, Y. M., Wang, Q. Y., Zhang, C. Z., Ma, D. H., Li, J. Y., and Zhang, J. B.: N, P and straw return influence the accrual of organic carbon fractions and microbial traits in a Mollisol, Geoderma, 403, 115373, https://doi.org/10.1016/j.geoderma.2021.115373, 2021.
Edgar, R. C.: Search and clustering orders of magnitude faster than BLAST, Bioinformatics, 26, 2460–2461, https://doi.org/10.1093/bioinformatics/btq461, 2010.
Fierer, N., Jackson, J. A., Vilgalys, R., and Jackson, R. B.: Assessment of soil microbial community structure by use of taxon-specific quantitative PCR assays, Appl. Environ. Microb., 71, 4117–4120, https://doi.org/10.1128/AEM.71.7.4117-4120.2005, 2005.
Frey, S. D., Lee, J., Melillo, J. M., and Six, J.: The temperature response of soil microbial efficiency and its feedback to climate, Nat. Clim. Change, 3, 395–398, https://doi.org/10.1038/NCLIMATE1796, 2013.
Ge, T., Li, B. Z., Zhu, Z. K., Hu, Y. J., Yuan, H. Z., Dorodnikov, M., Jones, D. L., Wu, J. S., and Kuzyakov, Y.: Rice rhizodeposition and its utilization by microbial groups depends on N fertilization, Biol. Fert. Soils., 53, 37–48, https://doi.org/10.1007/s00374-016-1155-z, 2017.
Geisseler, D. and Horwath, W. R.: Relationship between carbon and nitrogen availability and extracellular enzyme activities in soil, Pedobiologia, 53, 87–98, https://doi.org/10.1016/j.pedobi.2009.06.002, 2009.
Ghannoum, M. A., Jurevic, R. J., Mukherjee, P. K., Cui, F., Sikaroodi, M., Naqvi, A., and Gillevet, P. M.: Characterization of the Oral Fungal Microbiome (Mycobiome) in Healthy Individuals, Plos Pathog., 6, e1000713, https://doi.org/10.1371/journal.ppat.1000713, 2010.
Gong, H. R., Li, J., Sun, M. X., Xu, X. B., and Ouyang, Z.: Lowering carbon footprint of wheat-maize cropping system in North China Plain: Through microbial fertilizer application with adaptive tillage, J. Clean. Prod., 268, 122255, https://doi.org/10.1016/j.jclepro.2020.122255, 2020.
Gregorich, E. G., Rochette, P., VandenBygaart, A. J., and Angers, D. A.: Greenhouse gas contributions of agricultural soils and potential mitigation practices in Eastern Canada, Soil Till. Res., 83, 53–72, https://doi.org/10.1016/j.still.2005.02.009, 2005.
Guo, T. F., Zhang, Q., Song, D. L., Ai, C., Zhang, S. Q., Yue, K., Huang, S. M., and Zhou, W.: Varying microbial utilization of straw-derived carbon with different long-term fertilization regimes explored by DNA stable-isotope probing, Eur. J. Soil Biol., 108, 103379, https://doi.org/10.1016/j.ejsobi.2021.103379, 2022.
Handa, I. T., Aerts, R., Berendse, F., Berg, M. P., Bruder, A., Butenschoen, O., Chauvet, E., Gessner, M. O., Jabiol, J., Makkonen, M., McKie, B. G., Malmqvist, B., Peeters, E. T. H. M., Scheu, S., Schmid, B., van Ruijven, J., Vos, V. C. A., and Hattenschwiler, S.: Consequences of biodiversity loss for litter decomposition across biomes, Nature, 509, 218–221, https://doi.org/10.1038/nature13247, 2014.
Hogberg, M. N., Chen, Y., and Hogberg, P.: Gross nitrogen mineralisation and fungi-to-bacteria ratios are negatively correlated in boreal forests, Biol. Fert. Soils, 44, 363–366, https://doi.org/10.1007/s00374-007-0215-9, 2007.
Hou, R. J., Li, T. X., Fu, Q., Liu, D., Li, M., Zhou, Z. Q., Li, Q. L., Zhao, H., Yu, P. F., and Yan, J. W.: The effect on soil nitrogen mineralization resulting from biochar and straw regulation in seasonally frozen agricultural ecosystem, J. Clean. Prod., 255, 120302, https://doi.org/10.1016/j.jclepro.2020.120302, 2020.
Kihara, J., Bolo, P., Kinyua, M., Nyawira, S. S., and Sommer, R.: Soil health and ecosystem services: Lessons from sub-Sahara Africa (SSA), Geoderma., 370, https://doi.org/10.1016/j.geoderma.2020.114342, 2020.
Latifmanesh, H., Deng, A. X., Li, L., Chen, Z. J., Zheng, Y. T., Bao, X. T., Zheng, C. Y., and Zhang, W. J.: How incorporation depth of corn straw affects straw decomposition rate and release in the wheat-corn cropping system, Agr. Ecosyst. Environ., 300, 107000, https://doi.org/10.1016/j.agee.2020.107000, 2020.
Lehmann, J., Bossio, D. A., Kogel-Knabner, I., and Rillig, M. C.: The concept and future prospects of soil health, Nat. Rev. Earth Environ., 1, 544–553, https://doi.org/10.1038/s43017-020-0080-8, 2020.
Li, H., Feng, W. T., He, X. H., Zhu, P., Gao, H. J., Sun, N., and Xu, M. G.: Chemical fertilizers could be completely replaced by manure to maintain high maize yield and soil organic carbon (SOC) when SOC reaches a threshold in the Northeast China Plain, J. Integr. Agr., 16, 937–946, https://doi.org/10.1016/S2095-3119(16)61559-9, 2017.
Li, J. Q., Ye, X. H., Zhang, Y. L., Chen, J., Yu, N., and Zou, H. T.: Maize Straw Deep-Burying Promotes Soil Bacteria Community Abundance and Improves Soil Fertility, J. Soil Sci. Plant. Nut., 21, 1397–1407, https://doi.org/10.1007/s42729-021-00448-6, 2021.
Liu, C., Wang, K., and Zheng, X.: Responses of N2O and CH4 fluxes to fertilizer nitrogen addition rates in an irrigated wheat-maize cropping system in northern China, Biogeosciences, 9, 839–850, https://doi.org/10.5194/bg-9-839-2012, 2012.
Liu, C., Lu, M., Cui, J., Li, B., and Fang, C. M.: Effects of straw carbon input on carbon dynamics in agricultural soils: a meta-analysis, Glob. Change Biol., 20, 1366–1381, https://doi.org/10.1111/gcb.12517, 2014.
Liu, E. K., Yan, C. R., Mei, X. R., He, W. Q., Bing, S. H., Ding, L. P., Liu, Q., Liu, S. A., and Fan, T. L.: Long-term effect of chemical fertilizer, straw, and manure on soil chemical and biological properties in northwest China, Geoderma., 158, 173–180, https://doi.org/10.1016/j.geoderma.2010.04.029, 2010.
Lu, R. K.: The Analysis Method of Soil Agricultural Chemistry, Chinese Agricultural Sciences and Technology Press, 2000 (in Chinese).
Mapanda, F., Wuta, M., Nyamangara, J., and Rees, R. M.: Effects of organic and mineral fertilizer nitrogen on greenhouse gas emissions and plant-captured carbon under maize cropping in Zimbabwe, Plant Soil., 343, 67–81, https://doi.org/10.1007/s11104-011-0753-7, 2011.
Ning, Q., Chen, L., Jia, Z. J., Zhang, C. Z., Ma, D. H., Li, F., Zhang, J. B., Li, D. M., Han, X. R., Cai, Z. J., Huang, S. M., Liu, W. Z., Zhu, B., and Li, Y.: Multiple long-term observations reveal a strategy for soil pH-dependent fertilization and fungal communities in support of agricultural production, Agr. Ecosyst. Environ., 293, 106837, https://doi.org/10.1016/j.agee.2020.106837, 2020.
Pan, G. X., Zhou, P., Li, Z. P., Smith, P., Li, L. Q., Qiu, D. S., Zhang, X. H., Xu, X. B., Shen, S. Y., and Chen, X. M.: Combined inorganic/organic fertilization enhances N efficiency and increases rice productivity through organic carbon accumulation in a rice paddy from the Tai Lake region, China, Agr. Ecosyst. Environ., 131, 274–280, https://doi.org/10.1016/j.agee.2009.01.020, 2009.
Qiu, Y. P., Jiang, Y., Guo, L. J., Zhang, L., Burkey, K. O., Zobel, R. W., Reberg-Horton, S. C., Shew, H. D., and Hui, S. J.: Shifts in the Composition and Activities of Denitrifiers Dominate CO2 Stimulation of N2O Emissions, Environ. Sci. Technol., 53, 11204–11213, https://doi.org/10.1021/acs.est.9b02983, 2019.
Ramirez, K. S., Craine, J. M., and Fierer, N.: Consistent effects of nitrogen amendments on soil microbial communities and processes across biomes, Glob. Change Biol., 18, 1918–1927, https://doi.org/10.1111/j.1365-2486.2012.02639.x, 2012.
Ramirez, P. B., Fuentes-Alburquenque, S., Diez, B., Vargas, I., and Bonilla, C. A.: Soil microbial community responses to labile organic carbon fractions in relation to soil type and land use along a climate gradient, Soil Biol. Biochem., 141, 107692, https://doi.org/10.1016/j.soilbio.2019.107692, 2020.
Robertson, G. P., Gross, K. L., Hamilton, S. K., Landis, D. A., Schmidt, T. M., Snapp, S. S., and Swinton, S. M.: Farming for Ecosystem Services: An Ecological Approach to Production Agriculture, Bioscience, 64, 404–415, https://doi.org/10.1016/j.soilbio.2016.04.018, 2014.
Shi, Y. J., Wang, J. F., Le Roux, X., Mu, C. S., Ao, Y. N., Gao, S., Zhang, J. W., and Knops, J. M. H.: Trade-offs and synergies between seed yield, forage yield, and N-related disservices for a semi-arid perennial grassland under different nitrogen fertilization strategies, Biol. Fert. Soil., 55, 497–509, https://doi.org/10.1007/s00374-019-01367-6, 2019.
Song, K., Sun, Y. F., Qin, Q., Sun, L. J., Zheng, X. Q., Terzaghi, W., Lv, W. G., and Xue, Y.: The Effects of Earthworms on Fungal Diversity and Community Structure in Farmland Soil With Returned Straw, Front. Microbiol., 11, 594265, https://doi.org/10.3389/fmicb.2020.594265, 2020.
Sun, Y., Zhu, M. J., Mi, W. H., and Wu, L. H.: Long-term impacts of nitrogen fertilization and straw incorporation on rice production and nitrogen recovery efficiency under plastic film mulching cultivation, J. Plant Nutr., 44, 213–227, https://doi.org/10.1080/01904167.2020.1806303, 2021.
Sun, Y., Xu, Y. H., Zhang, J. N., Bello, A., Li, X., Liu, W. Y., Egbeagu, U. U., Zhao, L. Y., Han, Y., Cheng, L. J., Zhang, W. H., Meng, Q. X., Bi, R. X., Zhao, M. M., Liu, X. D., Sun, L., Gai, Z. X., Shi, S., Jong, C., and Xu, X. H.: Investigation of underlying links between nitrogen transformation and microorganisms' network modularity in the novel static aerobic composting of dairy manure by “stepwise verification interaction analysis”, Sci. Total Environ., 883, 163674, https://doi.org/10.1016/j.scitotenv.2023.163674, 2023.
Tang, Q., Ti, C. P., Xia, L. L., Xia, Y. Q., Wei, Z. J., and Yan, X. Y.: Ecosystem services of partial organic substitution for chemical fertilizer in a peri-urban zone in China, J. Clean. Prod., 224, 779–788, https://doi.org/10.1016/j.jclepro.2019.03.201, 2019.
Treseder, K. K.: Nitrogen additions and microbial biomass: a meta-analysis of ecosystem studies, Ecol. Lett., 11, 1111–1120, https://doi.org/10.1111/j.1461-0248.2008.01230.x, 2008.
Trost, B., Prochnow, A., Meyer-Aurich, A., Drastig, K., Baumecker, M., and Ellmer, F.: Effects of irrigation and nitrogen fertilization on the greenhouse gas emissions of a cropping system on a sandy soil in northeast Germany, Eur. J. Agron., 81, 117–128, https://doi.org/10.1016/j.eja.2016.09.008, 2016.
Vance, E. D., Brookes, P. C., and Jenkinson, D. S.: An Extraction Method for Measuring Soil Microbial Biomass-C, Soil Biol. Biochem., 19, 703–707, https://doi.org/10.1016/0038-0717(87)90052-6, 1987.
Wagg, C., Bender, S. F., Widmer, F., and van der Heijden, M. G. A.: Soil biodiversity and soil community composition determine ecosystem multifunctionality, P. Natl. Acad. Sci. USA, 111, 5266–5270, https://doi.org/10.1073/pnas.1320054111, 2014.
Wan, X. H., Huang, Z. Q., He, Z. M., Yu, Z. P., Wang, M. H., Davis, M. R., and Yang, Y. S.: Soil C: N ratio is the major determinant of soil microbial community structure in subtropical coniferous and broadleaf forest plantations, Plant Soil., 387, 103–116, https://doi.org/10.1007/s11104-014-2277-4, 2015.
Wang, D. D., Zhu, Z. K., Shahbaz, M., Chen, L., Liu, S. L., Inubushi, K., Wu, J. S., and Ge, T. D.: Split N and P addition decreases straw mineralization and the priming effect of a paddy soil: a 100-day incubation experiment, Biol. Fert. Soils, 55, 701–712, https://doi.org/10.1007/s00374-019-01383-6, 2019.
Wang, W. Q., Sardans, J., Wang, C., Pan, T., Zeng, C. S., Lai, D. Y. F., Bartrons, M., and Penuelas, J.: Straw Application Strategy to Optimize Nutrient Release in a Southeastern China Rice Cropland, Agronomy, 7, 84, https://doi.org/10.3390/agronomy7040084, 2017.
Williams, A., Borjesson, G., and Hedlund, K.: The effects of 55 years of different inorganic fertiliser regimes on soil properties and microbial community composition, Soil Biol. Biochem., 67, 41–46, https://doi.org/10.1016/j.soilbio.2013.08.008, 2013.
Wu, J., Joergensen, R. G., Pommerening, B., Chaussod, R., and Brookes, P. C.: Measurement Of Soil Microbial Biomass C by Fumigation Extraction – an Automated Procedure, Soil Biol. Biochem., 22, 1167–1169, https://doi.org/10.1016/0038-0717(90)90046-3, 1990.
Wu, L., Zhang, W. J., Wei, W. J., He, Z. L., Kuzyakov, Y., Bol, R., and Hu, R. G.: Soil organic matter priming and carbon balance after straw addition is regulated by long-term fertilization, Soil Biol. Biochem., 135, 383–391, https://doi.org/10.1016/j.soilbio.2019.06.003, 2019.
Xu, X. B., Liu, J. P., Tan, Y., and Yang, G. S.: Quantifying and optimizing agroecosystem services in China's Taihu Lake Basin, J. Environ. Manag., 277, 111440, https://doi.org/10.1016/j.jenvman.2020.111440, 2021.
Yin, H. J., Zhao, W. Q., Li, T., Cheng, X. Y., and Liu, Q.: Balancing straw returning and chemical fertilizers in China: Role of straw nutrient resources, Renew. Sust. Energ. Rev., 81, 2695–2702, https://doi.org/10.1016/j.rser.2017.06.076, 2018.
Zhang, Q., Liang, G. Q., Guo, T. F., He, P., Wang, X. B., and Zhou, W.: Evident variations of fungal and actinobacterial cellulolytic communities associated with different humified particle-size fractions in a long-term fertilizer experiment, Soil Biol. Biochem., 113, 1–13, https://doi.org/10.1016/j.soilbio.2017.05.022, 2017.
Zhang, J. and Elser, J. J.: Carbon:Nitrogen:Phosphorus Stoichiometry in Fungi: A Meta-Analysis, Front. Microbiol., 8, 1281, https://doi.org/10.3389/fmicb.2017.01281, 2017.
Zhao, Y. C., Wang, M. Y., Hu, S. J., Zhang, X. D., Ouyang, Z., Zhang, G. L., Huang, B. A., Zhao, S. W., Wu, J. S., Xie, D. T., Zhu, B., Yu, D. S., Pan, X. Z., Xu, S. X., and Shi, X. Z.: Economics- and policy-driven organic carbon input enhancement dominates soil organic carbon accumulation in Chinese croplands, P. Natl. Acad. Sci. USA, 115, 4045–4050, https://doi.org/10.1073/pnas.1700292114, 2018.
Zhong, L., Wu, T., Ding, J., Xu, W., Yuan, F., Liu, B.F., Zhao, L., Li, Y., Ren, N. Q., and Yang, S. S.: Co-composting of faecal sludge and carbon-rich wastes in the earthworm's synergistic cooperation system: Performance, global warming potential andkey microbiome, Sci. Total Environ., 857, 159311, https://doi.org/10.1016/j.scitotenv.2022.159311, 2022.