the Creative Commons Attribution 4.0 License.
the Creative Commons Attribution 4.0 License.
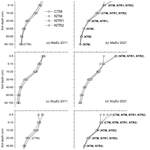
Diachronic assessment of soil organic C and N dynamics under long-term no-till cropping systems in the tropical upland of Cambodia
Rémi Cardinael
Florent Tivet
Vang Seng
Phearum Mark
Pascal Lienhard
Titouan Filloux
Johan Six
Lyda Hok
Stéphane Boulakia
Clever Briedis
João Carlos de Moraes Sá
Laurent Thuriès
No-till (NT) cropping systems have been proposed as a strategy to combat soil degradation by storing soil organic carbon (SOC) and total nitrogen (TN). We quantified the impacts of NT cropping systems on the changes in SOC and TN stocks and in particulate and mineral-associated organic matter fractions (POM and MAOM), to 100 cm depth, from three 13-year-old experiments in a tropical red Oxisol in Cambodia using diachronic and equivalent soil mass approaches. Established in 2009 and arranged in a randomized complete-block design with triplicates, the experiments included maize (MaiEx)-, soybean (SoyEx)-, and cassava (CasEx)-based cropping systems. Each experiment comprised three treatments: (1) mono-cropping of main crops (maize, soybean, and cassava) under conventional tillage (CTM); (2) mono-cropping of main crops under NT systems with the use of cover crops (NTM); and (3) bi-annual rotation of main crops under NT systems with the use of cover crops (NTR), with both crops being presented every year and represented by NTR1 and NTR2. Soil samples were collected in 2021, 10 years after the last sampling. All the NT systems significantly (p<0.05) increased SOC stock in the topsoil in SoyEx and MaiEx and down to 40 cm in CasEx. Considering the whole profile (0–100 cm), the SOC accumulation rates ranged from 0.86 to 1.47 and from 0.70 to 1.07 Mg C ha−1 yr−1 in MaiEx and CasEx, respectively. Although SOC stock significantly increased in CTM at 0–20 cm in MaiEx and CasEx, it remained stable at 0–100 cm in all the experiments. At 0–5 cm, NTR systems significantly increased TN stock in all the experiments, while, in NTM systems, it was only significant in MaiEx and SoyEx. At 0–100 cm, TN stock in all the experiments remained stable under NTR systems, whereas a significant decrease was observed under NTM systems in SoyEx and CasEx. Although C-POM stock significantly increased under all NT systems limited to 0–10 cm in MaiEx and SoyEx, all the NT systems significantly increased C-MAOM stock in the 0–10 cm layer in MaiEx and SoyEx and down to 40 cm in CasEx. All the NT systems significantly increased N-POM stock at 0–10 cm in MaiEx and SoyEx, while a significant decreased in N-MAOM stock was observed below 5 cm in CasEx and below 40 cm in MaiEx and SoyEx. Our findings showed that long-term NT systems with crop species diversification accumulated SOC not only on the surface but also in the whole profile by increasing SOC in both the POM and MAOM, even in the cassava-based system. This study highlights the potential of NT systems for storing SOC over time but raises questions about soil N dynamics.
- Article
(3056 KB) - Full-text XML
-
Supplement
(799 KB) - BibTeX
- EndNote
Land and soil degradation is a global challenge with consequences not only for food and nutrition security but also for livelihoods, environmental pollution, climate change, water scarcity, and biodiversity. The main processes that cause soil degradation are water and wind erosion, chemical depletion, physical deterioration, declines in soil organic carbon (SOC), losses in biodiversity, acidification, and salinity (Lal, 2015a; Stavi and Lal, 2015; Dragović and Vulević, 2020; Barbier and Di Falco, 2021). It was estimated that about 5.62×106 ha of land are degraded worldwide, with 5 to 10×106 ha of land lost each year as a result of severe degradation (Stavi and Lal, 2015; Nkonya et al., 2016). The major factors contributing to soil degradation are deforestation and land clearance, the overuse of agrochemicals, and intensive agricultural management practices (Dragović and Vulević, 2020). Tropical soils have the highest risks of degradation due to the combination of high rainfall intensity and the ongoing intensification of agriculture to meet the food demand of a fast-growing population, which is also constrained by the limited availability of land to be converted to cropland (Barbier and Hochard, 2018; Craswell and Lefroy, 2001; Barbier and Di Falco, 2021).
Cambodia, located in the tropical region of Southeast Asia, is one of the highest land degradation hotspots in the world, and about 55 % of the country's population resides in these hotspot areas (UNCCD, 2018). In the last 2 decades, human-induced activities including deforestation, land clearance for agriculture, climate change, and intensive farming practices have further worsened Cambodia's already poor soil fertility (UNCCD, 2018; Ken et al., 2020; ADB, 2021). Over the past 2 decades, 30 %, or about 4.24×106 ha, of forest areas were converted to croplands, putting pressure on natural resources and biodiversity and threatening the provision of several ecosystem services (World Bank Group, 2023). In the northwestern rainfed uplands, like in other parts of the country, studies on soil erosion at the field scale and modelling reported that the annual soil loss rate in conventional plough-based tillage (CT) ranged from 0.33 to more than 80 Mg soil ha−1 yr−1, depending on soil type and land slope (CARDI, 2017; Nut et al., 2021; Sourn et al., 2022). The amplitude of soil erosion increased by 41 % from an annual erosion rate of 2.92 Mg soil ha−1 yr−1 in 1998, at the beginning of the forest conversion to agriculture with extensive, more diversified farming practices, to 4.98 Mg soil ha−1 yr−1 in 2018 under CT maize- and cassava-based mono-cropping systems (Nut et al., 2021; Sourn et al., 2022, 2023). It was estimated that approximately 3–4 mm of topsoil is washed away annually for this northwestern region of Cambodia (Nut et al., 2021; Sourn et al., 2023). Erosion induces soil degradation and a loss of SOC for the eroded fields (Polyakov and Lal, 2004). It was estimated that, from 2000 to 2010, Cambodia lost approximately 1.98×106 Mg C in the top 0–30 cm depth as the consequence of forest conversion to other land uses (MAFF, 2018). Cambodian soils are seriously threatened by intensive agricultural systems. The returns on taking actions against land degradation through restoration and adoption of sustainable agricultural management practices are estimated at USD 3 for every dollar invested in restoring degraded land in Cambodia, highlighting the strong economic benefits (UNCCD, 2018).
SOC serves as the foundation of soil physical, chemical, and biological processes that sustain essential ecosystem functions, and it is the reservoir of plant nutrients and energy for biota (Lal, 2015a). Therefore, adopting sustainable management practices that lead to increases in SOC stock (Beillouin et al., 2023) is part of the key strategies to reverse the soil degradation trends and to minimize the economic and environmental impacts related to land degradation (Lal, 2015a; Obalum et al., 2017). Studies reported that agricultural practices, particularly those based on CT, weaken soil structure, accelerate soil erosion, and deplete SOC stock (Tivet et al., 2013; Sá et al., 2014; Briedis et al., 2018; Oliveira et al., 2020; Tiecher et al., 2020). By contrast, conservation agriculture (CA), defined by three key principles, namely (i) minimum or no soil disturbance, (ii) permanent soil cover with mulch or cover crops, and (iii) crop diversification through rotation or association, is a potential strategy to overcome soil degradation (Luo et al., 2010; Lal, 2015b; Powlson et al., 2016; Obalum et al., 2017). No-till (NT) cropping systems are part of the conservation agriculture practice approach and involve a range of practices with a reduction in or an absence of soil tillage and a high diversity of crop and cover crop species. The benefits of NT cropping systems for soil health improvement have been reported worldwide. Diversified NT cropping systems enhance both SOC stock (Hok et al., 2015) and pools (Sá et al., 2014; Briedis et al., 2018; Sithole et al., 2019; Cooper et al., 2021; Rodrigues et al., 2022), especially through an increase in the physical protection of particulate organic C (Sithole et al., 2019) and mineral-associated organic C (Rodrigues et al., 2022) inside soil aggregates. In addition, numerous studies have reported the co-benefits of NT cropping systems for soil health enhancement (Pheap et al., 2019; Koun et al., 2023), increased water infiltration, reduced soil erosion (TerAvest et al., 2015; Sithole et al., 2019), and enhanced microbial activities (Hok et al., 2018) and abundance (Lienhard et al., 2013). Yet, there are still arguments about the benefits of NT cropping systems and associated factors that determine SOC accumulation. Particulate organic matter (POM) and mineral-associated organic matter (MAOM) are the two main fractions of the SOC pools. They differ in terms of their physical and chemical characteristics, as well as in their turnover rates. POM is more sensitive to soil tillage and land use than MAOM and total SOC (Blanco-Moure et al., 2013; Kan et al., 2021). Therefore, documentation of SOC fractions is desirable for a better understanding of SOC dynamics and stabilization processes (Lavallee et al., 2020). In a meta-analysis, with the majority of the studies collecting samples between 0.15 and 0.3 m depth, Powlson et al. (2016) reported that SOC accumulation rates under CA systems ranged from 0.16 to 0.49 Mg C ha−1 yr−1 in tropical soils in the Indo-Gangetic Plain and from 0.28 to 0.96 Mg C ha−1 yr−1 in Sub-Saharan Africa. In a Ferralsol in Zimbabwe, Shumba et al. (2024) reported an SOC accumulation rate of 0.13 Mg C ha−1 yr−1 in the 0–5 cm layer under CA only but no change under NT only. However, in meta-analyses, Angers and Eriksen-Hamel (2008) and Luo et al. (2010) found that conversion from CT to NT only changed the SOC distribution in the soil profile but did not significantly increase SOC stock in the whole profile. Xiao et al. (2020) reported that NT significantly increased SOC stock only at the soil surface but not in the deeper layers. It is, therefore, crucial to quantify SOC change in subsoil when assessing the impact of practices, especially NT systems.
SOC storage is closely related to soil aggregate structure (Six et al., 2004; Liu et al., 2021). The complexity of cropping systems, characterized by crop species diversity through the use of cover crops, crop rotation, and intercropping, was reported to enhance soil aggregation stability and the proportion of soil macro-aggregates, along with an increase in SOC (Tiemann et al., 2015; Li et al., 2024). The diversity of crop species increased the quantity and chemical diversity of plant-derived litter inputs, which are the main sources of energy for soil microorganisms, and increased the microbial activity and abundance of fungal and bacterial communities (Tiemann et al., 2015; Zhang et al., 2023). The overall increases in fungal hyphae, plant roots, and aboveground biomass inputs under crop diversification are important organic binding agents that promote the formation of macro-aggregates and facilitate the soil aggregation process (Tiemann et al., 2015). Furthermore, the increased amount and diversity of plant-derived C inputs in the forms of crop residues and root exudates provided a suitable microenvironment for soil microorganisms, which promoted microbial growth and turnover (Morugán-Coronado, 2022). The faster microbial growth and turnover rates increased the amount of microbial biomass and necromass, thus increasing SOC (Liang et al., 2011; Prommer et al., 2019). The amount, quality, and frequency of the crop residues added to soil under a range of climate-driven decomposition rates, soil mineralogies, and profile characteristics are important factors to consider to increase SOC stocks (Paustian et al., 1997; Six et al., 2002; Bayer et al., 2006; Ogle et al., 2012; Virto et al., 2012). It has been suggested that the amount of biomass-C inputs was the main factor explaining the variability in SOC storage between sites under NT (Virto et al., 2012). In a synthesis from tropical soils, Fujisaki et al. (2018) reported that the amount of biomass-C inputs was the main factor driving SOC stock change. In a meta-analysis in Sub-Saharan Africa, Corbeels et al. (2019) found that no tillage alone does not lead to an increase in SOC stock, but CA systems combining the three principles could. It therefore seems that there is a hierarchy in CA principles when it comes to increasing SOC stock, the most important one being the permanent soil cover, followed by a reduction in soil tillage and improved rotations (Shumba et al., 2024). This has been confirmed in a recent second-order meta-analysis where crop residue retention and cover crops were the most efficient CA practices with regard to increasing SOC (Beillouin et al., 2023).
Two different soil-sampling approaches are commonly used for assessing SOC stock change, the diachronic and the synchronic approaches (Bernoux et al., 2005). The diachronic approach refers to collecting samples from the same field plots over time. The synchronic approach, also known as the space-for-time method, refers to sample collection at the same time from different (often adjacent) field plots under different land use or management systems (Bernoux et al., 2005; Neto et al., 2010). Neto et al. (2010) and Junior et al. (2013) revealed that the synchronic approach led to biased estimation of SOC accumulation from long-term experiments in Brazil due to spatial heterogeneity and initial land use history. They highlighted that diachronic soil sampling should be used for assessing SOC storage rates in relation to changes in land use or management patterns because it offers a more comprehensive view of how SOC and N levels change under long-term tillage and cropping systems over time in which non-identical initial soil conditions cannot practically be excluded, making it more accurate and realistic for the investigation of SOC and N dynamics despite the fact that they are costly and require significant time and resources to investigate (Bernoux et al., 2005; Neto et al., 2010; Junior et al., 2013). The synchronic approach, on the other hand, is simpler, lower-cost, and less time-consuming but comes with more uncertainty (Neto et al., 2010; Junior et al., 2013). A change in soil bulk density is often observed when comparing NT systems to CT due to differences in tillage but also due to the root systems of cover crops. It is, therefore, required that one estimate SOC change using an equivalent soil mass approach instead of a fixed-depth approach (Ellert and Bettany, 1995).
NT cropping systems have been promoted to smallholders in various agroecosystems in Cambodia since 2009. The early effects of NT cropping systems on soil health and SOC storage have been reported in several studies (Hok et al., 2015, 2018, 2021; Pheap et al., 2019; Suong et al., 2019; Sar, 2021; Koun et al., 2023); however, the information on the impact of long-term NT systems on the changes in SOC and TN stocks remains scarce in the country, as well as in Southeast Asia generally. There is a need to document the long-term changes in SOC and TN stocks under NT cropping systems to fill in the knowledge gaps, as well as to provide robust evidence to land use planners and policymakers. This could be profitable not only for Cambodia but also for other countries in the region.
Therefore, this study aimed to quantify the impacts of CT and different NT cropping systems on the changes in SOC and TN stocks and fractions over time (2011–2021) in Cambodia's tropical red Oxisol using diachronic and equivalent soil mass (ESM) approaches. We hypothesized that implementation of the three core technical principles of CA would significantly enhance the SOC stocks in both the POM and MAOM size fractions, including in the subsoils.
2.1 Study site description
The study was conducted at Bos Khnor Conservation Agriculture Research Station, the oldest CA research station in Southeast Asia, which belongs to the General Directorate of Agriculture (GDA), Department of Agricultural Land Resources Management (DALRM). It is located in the district of Chamkar Leu, Kampong Cham Province (12°12′31.0′′ N, 105°19′07.0′′ E; 118 m above sea level). The details of the study site were reported in Hok et al. (2015). Briefly, the site was the natural tropical rainforest, which was then converted into perennial cropland in 1937. The crops included cashew, coffee, mango, mulberry, avocado, and rubber, which were planted soon after forest clearance. Because of the civil war (Khmer Rouge) between 1970 and 1982, the area was abandoned and taken over by several tree species, such as Tetrameles nudiflora R Br., Nauclea officinalis L., Cassia siamea (Lam.) H. S. Irwin and Barneby, and Leucaena leucocephala (Lam.) de Wit, which grew naturally. The farming was resumed, and cotton (Gossypium hirsutum L.) and banana (Musa acuminata spp.) were planted from 1982 to 2000. From 2000 to 2009, successive annual crops per year of cotton, then mung bean (Vigna radiata (L.) R. Wilczek) and sesame (Sesamum indicum L.), and finally soybean (Glycine max L.) were rotated under conventional plough-based management before the establishment of the three experiments. Mineral fertilizers such as NPK (15–15–15), ammonium phosphate (16–20–0), and potassium chloride (0–0–60) were applied to the crops without lime application. The soil of the study site is classified as a red Oxisol (USDA, 1999) or a Ferralsol according to the World Reference Base (WRB) for Soil Resources (IUSS Working Group WRB, 2015), with 1 % sand, 29 % silt, and 69 % clay in the top 0–20 cm, gradually increasing with soil depth to 78 % clay at 20–100 cm. The clay fraction is mainly made of kaolinite (Hok et al., 2015). The land on the site is flat, and the land slope is < 1 %. Prior to the establishment of the three experiments in 2009, the average SOC and TN stocks in the 0–20 cm layer were 33.6 Mg C ha−1 and 3.33 Mg N ha−1, respectively. The research site's climate is defined as tropical monsoon, corresponding to the Am Köppen climate classification, with two main seasons: the wet season from May to October and the dry season from November to April. The mean annual temperature from 2009 to 2021 was 27.5 °C, while the average annual minimum and maximum temperatures were 22 and 35 °C, respectively. The annual rainfall from 2009 to 2021 ranged between 1650 and 2000 mm.
2.2 Experimental design, treatment description, and crop management
The detailed histories of the research site, experimental design, treatment description, and fertilizer application were reported in Hok et al. (2015) and Pheap et al. (2019). Our study covers three separate experiments, implemented in 2009, including (i) maize (Zea mays L.) (which was a former rice (Oryza sativa L.)-based trial from 2009 to 2019 and shifted to a maize-based trial in 2020), (ii) soybean (Glycine max L.), and (iii) cassava (Manihot esculenta Crantz) cropping system trials, hereafter called MaiEx, SoyEx, and CasEx, respectively. These represent the most important annual upland crops in Cambodia, as well as in some Southeast Asian countries. Each experiment is arranged in a randomized complete-block design with three replicates. The elementary plot dimensions are 8 m × 37.5 m, equivalent to 300 m2. Each experiment consists of three treatments, including (1) mono-cropping under conventional tillage (CTM), in which the main crops, i.e. maize (Mz), soybean (Sb), or cassava (Cs), were mono-cropped with land preparation done by disc ploughing (CTM-Mz, CTM-Sb, and CTM-Cs); (2) mono-cropping under NT systems with the use of cover crops (NTM), in which the main crops (maize, soybean, or cassava) were cropped in a 1-year frequency pattern with no soil tillage and with the addition of cover crops (NTM-Mz, NTM-Sb, and NTM-Cs); and (3) bi-annual rotation of the main crops under NT systems with the use of cover crops (NTR), where the main crops were presented every year in two separate elementary plots designated as NTR1 and NTR2. For treatment (3) of SoyEx and CasEx, represented by NTR1-Sb and NTR1-Cs, respectively, the main crops (i.e. soybean and cassava) were grown in a bi-annual rotation with maize, represented by NTR2-Sb and NTR2-Cs for SoyEx and CasEx, respectively. For the treatment (3) of MaiEx, the main crop (i.e. maize represented by NTR1-Mz) was grown in a bi-annual crop rotation with soybean, represented by NTR2-Mz (Table 1). Under all the NT systems, the species, sowing dates, and methods of cover crop establishment varied depending on the design of the treatments for each experiment, the types and cycles of the main crops, and the species and cycles of the cover crops (Table 1). For instance, stylo (Stylosanthes guianensis (Aublet) Sw.) and Brachiaria (Brachiaria ruziziensis R. Germ. and C. M. Evrard) were associated with rice and soybean, respectively, by manual broadcasting at the full flowering stage of rice before the end of September and with the first yellow leaves of soybean in the middle of October. Stylo was associated by line sowing with an NT planter on the same date of maize cultivation and 20 d after planting for cassava. In addition, if the development and/or density of the cover crop sown the previous year were considered to be insufficient, short-cycle cover crop species, i.e. pearl millet (Pennisetum typhoides (L.) Morrone) or sorghum (Sorghum bicolor (L.) Moench), were sown alone or mixed with cowpea (Vigna unguiculata (L.) Walp. and sunn hemp (Crotalaria juncea L.) at the beginning of the rainy season (in the first week of May). Cover crops were then grown for 60–75 d to increase the biomass inputs prior to the cultivation of the main cycle of rice, soybean, or maize (Table 1).
The establishment and harvest of the main crops varied depending on the species. For maize, upland rice, and soybean, with a life cycle of approximately 110–120 d, these crops were mainly seeded between the last week of June to mid-July and were harvested between mid-October and mid-November, whereas cassava was planted in early May and harvested at around 10 months old in mid-February of the following year. For main crop residue management in MaiEx and SoyEx, all crop residues were retained in the soil in all the tillage systems. In CasEx, under CTM-Cs, all the fallen leaves and branches of cassava were retained in the soil, while 100 % of the cassava main stems and original cuttings were completely removed from the plot after harvesting, representing standard farming practices. For all the NT-Cs systems, all the fallen leaves and branches of cassava were returned to the soil, while 50 % of the cassava main stems and 100 % of the original cuttings were retained in the soil and then crimped to speed up the decomposition process and to facilitate field operation implementations in the following cropping season. The residues of all the cover crops were left as mulch under all the NT systems in all the experiments.
There were a few adjustments to the cropping systems over the experimental period from 2009 to 2021, especially with the use of cover crops, crop varieties, and mineral fertilizer application types and rates. Details of the main crops and cover crop successions and the cumulative amount of the aboveground biomass-C and N inputs from the crop residues are presented in Table 1. The C inputs were estimated from the dry aboveground biomass inputs recorded prior to the termination of the cover crops and during grain and/or tuber harvests for the main crops. The C and N inputs from the root systems were neither recorded nor estimated based on the literature. In the case of missing data regarding aboveground biomass, the amount of biomass was estimated using the average of the recorded data over time as a reference in the case of cover crops and using the grain and/or tuber aboveground biomass ratio for the main crops (e.g. rice, maize, soybean, and cassava). In addition, the cumulative and annual N inputs were estimated from the amount of the cumulative and annual C inputs, respectively, by applying the available ratio values of each plant species that were obtained from the C and N concentration analysis by dry combustion.
For land preparation, the CTM plots were ploughed twice to 15–20 cm depth using a seven-disc plough after early rains at the beginning of the wet season and then before the main crop cultivation. If sufficient early rainfalls were received at the beginning of the wet season (in the third week of March), sesame and mung bean were sown manually under CTM treatment in SoyEx and MaiEx, respectively, as early-cycle cash crops (April to June) prior to the main crops, i.e. soybean or maize (from July to November). If that was not the case, the CTM plots remained fallow with the growth of natural grasses and broad leaves until the main-cycle crops. These cropping systems represent the standard farming practices. Under the NT systems (NTM, NTR1, and NTR2), a long-cycle cover crop, i.e. stylo, was used as a cover crop and was grown in association with the main crops. This cover crop was sown in the middle of the inter-row at 0, 15, and 35 d after the sowing of the main crops, i.e. maize, cassava, and rice, respectively, and by seed broadcasting at the first yellow leaves of soybean, approximately 4 weeks before harvest. In addition, if the development and/or density of the cover crop sown the previous year were considered to be insufficient, pearl millet or sorghum was sown alone for the treatments planted with soybean or mixed with sunn hemp and cowpea for the treatments planted with maize at the beginning of the rainy season as short-cycle cover crops. The cover crops were then grown for 60–75 d prior to the main cycle of rice, maize, or soybean. The main crops (rice, maize, and soybean) under both CTM and all the NT systems, as well as the cover crops (at the beginning of the rainy season), were sown by an NT planter (Fitarelli pulled by power tiller, Vence Tudo, or Semeato lifted or pulled by tractor). From 2009 to 2020, cassava was planted along the furrows drawn by chiselling at 0.8 m spacing to approximately 20 cm depth, and then it was planted by an NT cassava planter (Planti Center) in 2021. Under the NT systems, the cover crops were terminated by crimping followed by the application of a mix of non-selective herbicides, i.e. glyphosate (N-(phosphonomethyl) glycine) and 2,4-D (2,4-dichlorophenoxyacetic acid), at a rate of 960 and 720 g active ingredient (a.i) per hectare, respectively.
Since 2009, soil amendment has been done with thermophosphate (16 % P2O5, 31 % CaO, and 16 % MgO) at the end of then dry season (early April), following which basal fertilizers and top dressings are applied to the main crops with different rates of N, P, and K, depending on the types and phenological stages of each main crop, using diammonium phosphate (18 % N, 46 % P2O5), ammonium phosphate (16 % N, 20 % P2O5), potassium chloride (60 % K2O), and urea (46 % N). The application of the fertilizer inputs to each main crop is detailed in Table S1 in the Supplement.
Table 1Experiments, cropping systems and crop sequences, and associated cumulative and annual aboveground C and organic N inputs from crop residues during the experimental period (2009–2021).
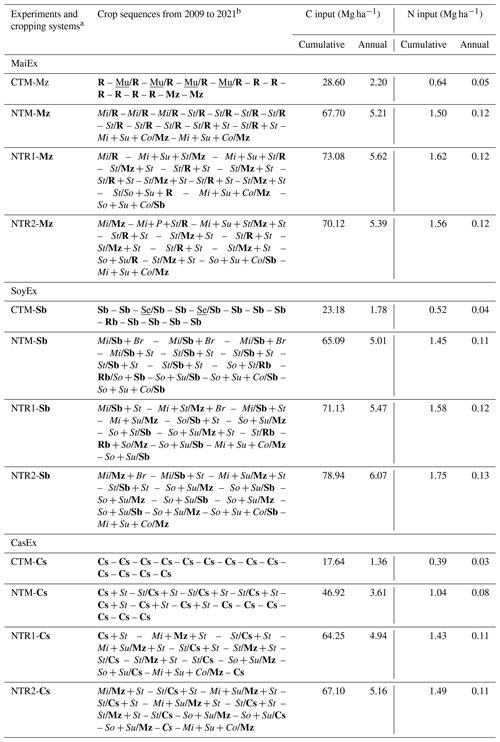
a MaiEx: maize-based cropping-system trial; SoyEx: soybean-based cropping-system trial; CasEx: cassava-based cropping-system trial; CTM: mono-cropping of the main crops under conventional tillage; NTM: mono-cropping of the main crops under no-till mulch-based cropping systems with the use of cover crops, and NTR1 and NTR2 refer to bi-annual rotation of the main crops under no-till mulch-based cropping systems with the use of cover crops. b Br: Brachiaria (Brachiaria ruziziensis R. Germ. and C. M. Evrard); Co: cowpea (Vigna unguiculata (L.) Walp.); Cs: cassava (Manihot esculenta Crantz); Mi: millet (Pennisetum glaucum (L.) R. Br.); Mu: mung bean (Vigna radiata (L.) R. Wilczek); Mz: maize (Zea mays L.); P: pigeon pea (Cajanus cajan L.); R: rice (Oryza sativa L.); Rb: rice bean (Vigna umbellata (Thunb.) Ohwi and H. Ohashi); Sb: soybean (Glycine max L.); Se: sesame (Sesame indicum L.); So: sorghum (Sorghum bicolor (L.) Moench); St: stylo (Stylosanthes guianensis (Aubl.) Sw.); Su: sunn hemp (Crotalaria juncea L.). The letters that are in bold, underlined, and italicized indicate the main crops, cash crops, and cover crops, respectively. Note that “–” indicates the period between the years, “/” indicates relay cropping with varying planting dates, and “+” indicates crops planted in association (same or staggered sowing dates). The C inputs were estimated from the amount of aboveground biomass of each crop; the belowground biomass was not included.
2.3 Soil sampling and processing
The study was a diachronic analysis from 2011 to 2021. In 2009, prior to the establishment of the experiments, soil and bulk density (ρb) samples were collected as the pre-experiment (PE) from three randomly selected sampling points per replicate of each experimental location at four depths: 0–5, 5–10, 10–20, and 20–30 cm. The individual soil samples from the same depth and replicate were composited, resulting in three composites per depth and per experiment. The composite samples were oven-dried at 40 °C and sieved through a 2 mm mesh for chemical property analysis. Bulk density samples were collected using core samplers of 5 cm in diameter and 5 cm in height and then were oven-dried at 105 °C for 48 h. The SOC and TN stocks of PE in 2009 in the top 0–20 cm were 33.3, 35.0, and 32.4 Mg C ha−1 and 3.34, 3.41, and 3.26 Mg N ha−1 in MaiEx, SoyEx, and CasEx, respectively (Fig. S1).
In November 2011, soil sampling was conducted to assess the early effects of tillage systems on soil organic C and N concentrations and stocks. The details of the sampling are described in Hok et al. (2015). Briefly, two pits (1 m × 1 m) were opened per elementary plot for soil and ρb sample collection. Individual samples for chemical analysis were collected from two undisturbed sides of each pit at 0–5, 5–10, 10–20, 20–40, 40–60, 60–80, and 80–100 cm. The individual samples from the same depth and the same pit were composited, and, as a result, two composite samples per layer were collected per elementary plot. The composites were oven-dried at 40 °C before being softly disrupted, sieved through a 2 mm sieve, and homogenized. Soil bulk density samples were taken from the same two undisturbed sides of each pit at the same soil depths as for SOC analysis using core samplers of 5 cm in diameter and 5 cm in height. The soil cores were oven-dried at 105 °C for 48 h.
In December 2021, we re-sampled the soil to assess the changes in ρb, SOC, and N concentrations and stocks 10 years after the study conducted by Hok et al. (2015). The samples were collected from the same seven layers. From each treatment and replicate, we collected four individual samples by means of an automatic soil column cylinder auger (a gasoline-powered percussion hammer Cobra TT with inner diameter of 85 mm, Eijkelkamp, the Netherlands) in a diagonal “X” shape from four points within each plot, avoiding overlapping with the pits opened in 2011. In addition, we dug a 1 m × 1 m pit in the middle of each plot for sample collection; three individual soil samples and three ρb cores were collected from three undisturbed sides of the pit at each depth. Soil samples were air-dried at room temperature, gently broken down, and sieved through a 2 mm mesh sieve. Finally, the seven individual samples from the same layer were mixed and homogenized to make a composite sample per elementary plot. The samples of ρb were oven-dried at 105 °C for 48 h.
2.4 Soil organic C and total N analyses
The concentrations of SOC and TN in the soil samples collected in 2009 and 2011 were determined by dry combustion using an elemental CN analyser (TruSpec CN, LECO, St. Joseph, USA). The details of the analysis were described in Hok et al. (2015). Sub-samples of the composite soils (n=3 per layer) collected in 2021 were finely ground (< 150 µm) before analysis for total C and N by dry combustion using the LECO® CHN628 analyser at the Sustainable Agroecosystems Lab, ETH Zurich University, Switzerland.
2.5 Soil organic C and total N stock calculation
In this Oxisol, there was no coarse fraction (i.e. gravels) left after sieving at 2 mm. Therefore, the bulk density of soil equals the bulk density of fine earth. To avoid inaccurate stock calculation due to differences in bulk density between treatments when using the fixed-depth method, the equivalent soil mass (ESM) approach was applied to compute SOC and TN stocks (Ellert and Bettany, 1995; Von Haden et al., 2020; Fowler et al., 2023). Since the ρb of the treatments differed between the two sampling years (2011 and 2021) at each sampling depth (Table S2), we defined the reference soil mass as the lowest soil mass observed at each sampling depth regardless of sampling years, cropping systems, or land use. For this reference, soil mass layers (480, 518, 1061, 1873, 1766, 1809, and 1779 Mg ha−1) corresponded to the depth layers (0–5, 5–10, 10–20, 20–40, 40–60, 60–80, and 80–100 cm, respectively). We applied these reference soil masses to compute the SOC and TN stocks in 2021 and recalculated the stocks of the PE and the treatments of the three experiments collected in 2009 and 2011.
To correct for differences in ρb, SOC and TN stocks were computed according to Eqs. (1) and (2).
In the above, M(soilmin,i) is the minimal soil mass per unit area in the ith layer (Mg ha−1) recorded over the treatments and used as a reference. ρb(i) is the bulk density of the ith layer (g cm−3). T(i) is the thickness of the ith layer (m). conc.(i) is the concentration of SOC in the ith layer. is the concentration of SOC in the layer i−1. M(soil,i) is the designated soil mass of each layer (i.e. the maximum soil mass). The numbers 1000 and 0.001 are unit conversion coefficients.
We defined the delta stock (Δ) of SOC and TN as the stock change within the same treatment and depth between the 2021 and 2011 sampling years (diachronic) and calculated it as follows:
where i represents the treatments.
To compare the synchronic and diachronic approaches for SOC stock change, the stock change estimated by the synchronic approach was computed as follows using the CTM treatment as the control treatment:
where NT(i) represents the NTM, NTR1, and NTR2 treatments.
The SOC and TN stock change (accumulation or loss) rates (Mg C or N ha−1 yr−1) of each treatment were calculated by dividing Δ SOC or TN stock by the number of years between the first and second samplings (10 years):
2.6 Particle size fractionation of soil organic matter
The soil organic C was physically fractionated using a sub-sample of the composite soil for all the treatments and seven depths. The particle size fractionation was implemented in accordance with the procedure described in Hok et al. (2015). Briefly, 40 g of each soil sample was dispersed in a solution of 1.25 g of sodium hexametaphosphate and 100 mL of deionized water and stored at 10 °C for 16 h. The sample was then horizontally shaken at 100 rpm for 8 h with three 10 mm diameter agate balls. The soil mixture was wet-sieved with deionized water through a 53 µm sieve to get the proportion of particulate organic matter (POM) sized between 53 and 2000 µm. The < 53 µm fraction was flocculated with 2 g CaCl2 in a 1 L glass cylinder and left overnight for sedimentation. The supernatant was syphoned after full sedimentation. This < 53 µm fraction is made up of mineral-associated organic matter (MAOM). The two fractions were oven-dried at 40 °C until reaching constant weight and were then finely ground for determining SOC and TN concentrations by dry combustion using the LECO® CHN628 analyser at the Sustainable Agroecosystems Lab, ETH Zurich University, Switzerland.
2.7 Statistical analysis
Statistical analysis was conducted using R software, version 4.3.1 (Core Team, 2023). Linear mixed models (lmerTest package) were fitted on all data: sampling years, soil depths, and treatments were defined as the fixed factors, while the replicates were defined as the random factors. Prior to the analysis, the normality of each variable was checked by means of Shapiro's test. Then we applied Levene's test to check the homoscedasticity of the data. A diachronic approach was used to assess the statistical significance between the 2 sampling years (i.e. 2021 vs. 2011) of the same treatment at the same soil depth by meas of analysis of variance with Fisher tests (degrees of freedom calculated by Satterthwaite method), computing of estimated marginal means (EMMs), and p-value adjustment using the Tukey method. The same approach was used to compare SOC stocks between treatments in the same sampling year, calculated at equivalent soil mass and using the synchronic approach. In addition, we applied the same statistical procedures to assess the statistical significance of cumulative SOC and TN stocks.
The effects of cropping systems on the concentrations, stocks of SOC and TN, and their fractions in the physical size classes between 2011 and 2021 varied among the three experiments and across the soil profile.
3.1 Impact of cropping systems on SOC concentration and stock
3.1.1 SOC concentration
The SOC concentration of all the treatments in 2011 and 2021 was highest in the topsoil (0–5 cm) and decreased with soil depth in all the experiments (Fig. 1, with Table S3 as duplication).
Over a 10-year period of historical cropping sequences from 2011 to 2021, all the NT cropping systems had significant effects (p<0.05) in relation to the increase in SOC concentration. On the other hand, the SOC concentration under CTM remained stable, with the exception of a few significant increases detected in the tilled layers in MaiEx and CasEx (Fig. 1).
In 2021, the mono-cropping of main crops under NT systems in MaiEx (NTM-Mz) and CasEx (NTM-Cs) exhibited a similar trend of increasing the SOC concentration significantly (p<0.05) across the soil profile compared to in 2011 (Figs. 1b and f). The SOC concentration under NTM-Mz increased by 68 %, 21 %, 16 %, 17 %, 23 %, and 16 % at 0–5, 5–10, 20–40, 40–60, 60–80, and 80–100 cm, respectively (Fig. 1b). The significant increase in SOC concentration under NTM-Cs was detected from 0 to 80 cm, with a gain of 26 %, 20 %, 19 %, 22 %, 18 %, and 10 % at 0–5, 5–10, 10–20, 20–40, 40–60, and 60–80 cm, respectively (Fig. 1f).
When compared to 2011, the bi-annual rotation of main crops under NT systems in MaiEx (NTR1-Mz and NTR2-Mz) and CasEx (NTR1-Cs and NTR2-Cs) significantly (p<0.05) increased SOC concentration from the surface down to subsoil depth in 2021 (Fig. 1b and f). On average, NTR-Mz (average of NTR1-Mz and NTR2-Mz) significantly increased the SOC concentration by 50 %, 24 %, and 15 % at 0–5, 5–10, and 10–20 cm depth, respectively. A significant increase was still observed under NTR1-Mz at 20–40 cm depth (Fig. 1b). In 2021, among the two treatments of the NTR-Cs crop rotation systems (NTR1-Cs and NTR2-Cs), NTR2-Cs significantly increased the SOC concentration in the top 0 to 60 cm by 30 %, 12 %, 13 %, 23 %, and 15 % at 0–5, 5–10, 10–20, 20–40, and 40–60 cm, respectively, while a significant decrease of -13 % was recorded in the 80–100 cm depth (Fig. 1e and f). Under NTR1-Cs, significant increases in the SOC concentration were observed up to 40 cm depth, with a gain of 24 %, 10 %, and 23 % at 0–5, 5–10, and 20–40 cm, respectively, with a significant decrease by −12 % at 80–100 cm depth (Fig. 1e and f).
Unlike MaiEx and CasEx, the significant increase (p<0.05) in SOC concentration under all the NT cropping systems in SoyEx (NTM-Sb, NTR1-Sb, and NTR2-Sb) in 2021 was only observed in the top 0–5 cm, with a similar increase of ∼ 7.5 g C kg−1 soil (Fig. 1d).
Over a decade of mono-cropping of main crops under conventional tillage in all the experiments (CTM-Mz, CTM-Sb, and CTM-Cs), the SOC concentration remained stable overall, with the exception of a few significant increases detected in the tilled layers in MaiEx (CTM-Mz at 10–20 cm) and CasEx (CTM-Cs at 0–5 and 5–10 cm) (Fig. 1b, d, and f).
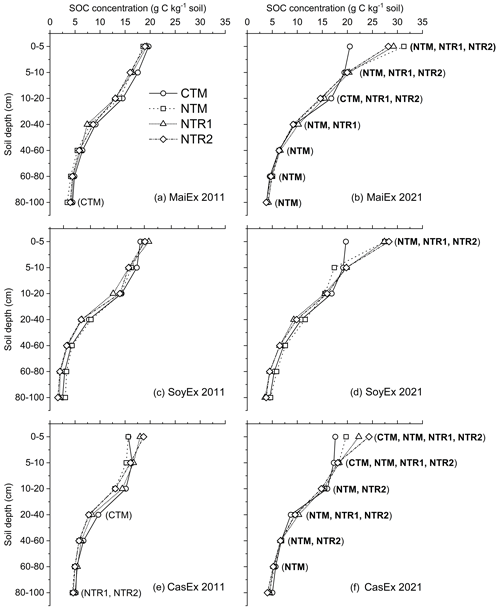
Figure 1SOC concentration distribution across the soil profile (0–100 cm) in relation to the treatments under different experiments in 2011 and 2021. CTM: mono-cropping of the main crops under conventional tillage; NTM: mono-cropping of the main crops under no-till mulch-based cropping systems with the use of cover crops, and NTR1 and NTR2 refer to bi-annual rotation of the main crops under no-till mulch-based cropping systems with the use of cover crops as described in Table 1. (a) MaiEx 2011: SOC concentration of the treatments in maize-based trial measured in 2011; (b) MaiEx 2021: SOC concentration of the treatments in maize-based trial measured in 2021; (c) SoyEx 2011: SOC concentration of the treatments in soybean-based trial measured in 2021; (d) SoyEx 2021: SOC concentration of the treatments in soybean-based trial measured in 2021; (e) CasEx 2011: SOC concentration of the treatments in cassava-based trial measured in 2011; and (f) CasEx 2021: SOC concentration of the treatments in cassava-based trial measured in 2021. Treatment(s) in bold within the brackets indicate the gain and significant (p<0.05) difference in concentrations between 2011 and 2021 for the same treatment at the same soil depth.
3.1.2 SOC stock
From 2011 to 2021, there were significant (p<0.05) increases in SOC stock, which varied depending on tillage, cropping systems, and the experiments (Tables 2 and S5). In 2021, in the case of MaiEx, the SOC stock under NTR-Mz crop rotation systems (average of NTR1-Mz and NTR2-Mz) significantly (p<0.05) increased by 4.6, 2.6, and 2.2 Mg C ha−1 at 0–5, 5–10, and 10–20 cm depth, respectively. NTR1-Mz showed a significant increase in SOC stock at a deeper profile at 20–40 cm, with a gain of 4.5 Mg C ha−1 (Tables 2 and S5). In the case of CasEx, the soils under NTR-Cs crop rotation systems (average of NTR1-Cs and NTR2-Cs) in 2021 significantly (p<0.05) increased SOC stock by an average of 2.4, 1.1, 1.4, and 2.9 Mg C ha−1 at 0–5, 5–10, 10–20, and 20–40 cm, respectively, but significantly decreased by an average of −0.9 Mg C ha−1 at 80–100 cm (Tables 2 and S5). For SoyEx, NTR-Sb crop rotation systems (average of NTR1-Sb and NTR2-Sb) significantly accumulated SOC stock by an average of 3.55 and 1.75 Mg C ha−1 at 0–5 and 5–10 cm, respectively, along with a positive trend from 10 to 80 cm depth (Tables 2 and S5).
Unlike SOC concentration, the significant effect of increasing SOC stock of the mono-cropping of main crops under NT systems varied across the three experiments (Table 2). NTM-Cs showed the significant (p<0.05) accumulation of SOC stock by 2.0, 1.6, 2.5, and 2.2 Mg C ha−1 in 0–5, 5–10, 10–20, and 20–40 cm, respectively (Tables 2 and S5). NTM-Mz significantly increased SOC stock in the surface layers (0–10 cm) by 6.1 and 2.2 Mg C ha−1 in the 0–5 and 5–10 cm, respectively, while the NTM-Sb significantly increased the stock by 3.6 Mg C ha−1 in the 0–5 cm (Tables 2 and S5).
In the case of the mono-cropping of main crops under conventional tillage, despite the fact that a few significant increases of 0.9 and 2.3 Mg C ha−1 in SOC stock were detected in the till layers in CTM-Mz (MaiEx) at 5–10 and 10–20 cm, respectively, the significant decline in SOC stock was observed below 60 cm, with a decrease of approximately −1.2 Mg C ha−1 at 60–80 and 80–100 cm depth (Table 2). For CasEx and SoyEx, despite the fact that a significant increase in SOC stock was observed in CTM-Cs from 0 to 20 cm, the accumulation rate was 2 times lower than in those NT systems, while no significant changes were recorded under CTM-Sb (Table 2).
Over the 10-year period from 2011 to 2021, considering a 100 cm layer as a single stratum, all the NT cropping systems significantly increased SOC stock, with accumulation rates ranging from 0.86 to 1.47 and 0.70 to 1.07 Mg C ha−1 yr−1 for NT-Mz and NT-Cs, respectively (Table 2). Although a non-significant difference was detected, all the NT-Sb systems increased SOC stock with annual accumulation rates ranging from 0.65 to 1.00 Mg C ha−1 yr−1 (Table 2). Despite the fact that a few significant increases in SOC stock were observed under CTM, the whole-profile SOC stock in all the CTM systems (CTM-Mz, CTM-Sb, and CTM-Cs) remained stable in 2021 (Table 2).
Table 2Mean SOC stocks in 2021 and SOC stock change rate between 2021 and 2011.
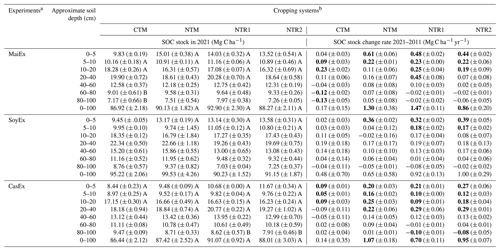
a MaiEx: maize-based experiment; SoyEx: soybean-based experiment; and CasEx: cassava-based experiment. b CTM: mono-cropping of the main crops under conventional tillage; NTM: mono-cropping of the main crops under no-till mulch-based cropping systems with the use of cover crops, and NTR1 and NTR2 refer to bi-annual rotation of the main crops under no-till mulch-based cropping systems with the use of cover crops as described in Table 1. Different uppercase letters accompanying the values indicate significant differences within the same treatment between 2011 and 2021 (diachronic) at the same soil depth at p<0.05 (Tukey's test). Values of SOC stock change rates in bold indicate that changes for a given treatment and depth between 2021 and 2011 are significantly different from 0 at p<0.05 (Tukey's test). Positive values of SOC stock change rate indicate an SOC accumulation; negative values indicate an SOC loss. Values in the parentheses indicate standard errors (n=3).
3.2 Impact of cropping systems on TN concentration and stock
3.2.1 Total N concentration
Over 10 years of cultivation from 2011 to 2021, surprisingly, the response of soil TN concentration to tillage and cropping systems differed from that of SOC (Fig. 2, with Table S4 as duplication). The positive (p<0.05) effect on TN concentration was mainly observed on the surface layer under NT systems. However, the significant (p<0.05) decrease in TN concentration varied across tillage, cropping systems, and experiments below 20 cm (Fig. 2, Table S4). In 2021, NTR systems (NTR1 and NTR2) significantly (p<0.05) increased the TN concentration in the top 5 cm of MaiEx (NTR-Mz) and SoyEx (NTR-Sb) by 32 % and 23 %, respectively (Fig. 2b and d), but decreased the TN concentration significantly (p<0.05) below 60 to 100 cm by −18 % to −21 % and −10 % to −25 % in MaiEx and SoyEx, respectively (Fig. 2a and b). Under CasEx in 2021, the soil TN concentration increased significantly (p<0.05) by 16 % in the top 0–5 cm under the NTR system (average of NTR1-Cs and NTR2-Cs), while the overall TN concentration remained stable below 5 cm, except for significant increases under NTR1-Cs by 10 % and 19 % at 5–10 and 20–40 cm, respectively (Fig. 2f).
From 2011 to 2021, in the case of mono-cropping under NT systems in MaiEx and SoyEx, there was a significant (p<0.05) increase in TN concentration by 44 % and 25 % under NTM-Mz and NTM-Sb, respectively (Fig. 2b and c). However, the TN concentration was significantly (p <0.05) decreased by −24 % at 40–60 cm under NTM-Mz and from −23 % to −29 % at 60–100 cm depth under NTM-Sb (Fig. 2a and b). After 10 years of cassava mono-cropping under the NT system (NTM-Cs), the TN concentration did not change in the top 0–10 cm, but the concentration decreased significantly (p<0.05) below 10–100 cm depth from −10 % to −25 % in 2021 (Fig. 2e).
In contrast to NT systems, after 10 years of conventional tillage-based mono-cropping of soybean (CTM-Sb) and cassava (CTM-Cs), the soil TN concentration in 2021 remained constant across the whole profile, except for a significant (p<0.05) decrease of −14 % in the 20–40 cm layer detected under CTM-Cs (Fig. 2d and e); on the other hand, in maize mono-cropping under CT (CTM-Mz), the soil TN concentration remained stable from 0 to 40 cm and then decreased significantly (p<0.05) by −12 % and −26 % at 40–60 and 80–100 cm depth, respectively (Fig. 2a and b).
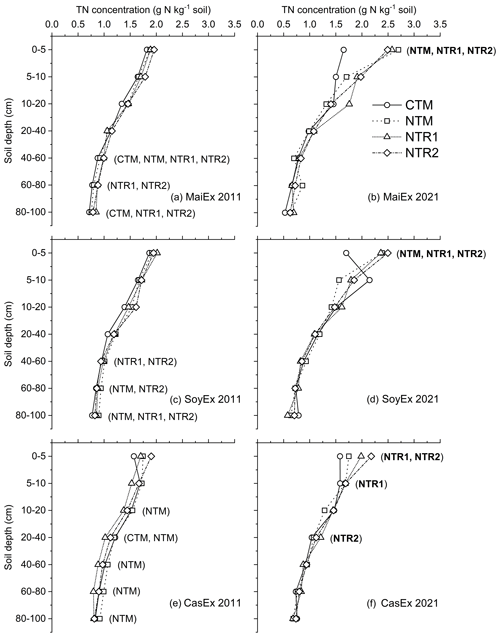
Figure 2TN concentration distribution across the soil profile (0–100 cm) in relation to the treatments under different experiments in 2011 and 2021. CTM: mono-cropping of the main crops under conventional tillage; NTM: mono-cropping of the main crops under no-till mulch-based cropping systems with the use of cover crops, and NTR1 and NTR2 refer to bi-annual rotation of the main crops under no-till mulch-based cropping systems with the use of cover crops as described in Table 1. (a) MaiEx 2011: TN concentration of the treatments in maize-based trial measured in 2011; (b) MaiEx 2021: TN concentration of the treatments in maize-based trial measured in 2021; (c) SoyEx 2011: TN concentration of the treatments in soybean-based trial measured in 2021; (d) SoyEx 2021: TN concentration of the treatments in soybean-based trial measured in 2021; (e) CasEx 2011: TN concentration of the treatments in cassava-based trial measured in 2011; and (f) CasEx 2021: TN concentration of the treatments in cassava-based trial measured in 2021. Treatment(s) in bold within the brackets indicate the gain and significant (p<0.05) difference in concentrations between 2011 and 2021 under the same treatment at the same soil depth.
3.2.2 Total N stock
Over the past decade, cultivating the main crops (maize, soybean, and cassava) under NTR systems (NTR1 and NTR2) significantly (p<0.05) increased TN stock in the soil surface in all the experiments in 2021. However, the response of TN stock below the surface layers to the NTR systems differed between the three experiments (Tables 3, S5). In the case of MaiEx (NTR1-Mz and NTR2-Mz), TN stock increased by 0.3 Mg N ha−1 at 0–5 cm; the stock remained stable at 5–40 cm but then decreased significantly (p<0.05) at 40–100 cm depth by between −0.25 and −0.40 Mg N ha−1 (Table 3). Similarly to MaiEx, the soil TN stock in the soils under NTR systems of SoyEx (NTR1-Sb and NTR2-Sb) significantly increased by 0.25 Mg N ha−1 in the 0–5 cm layer, remained constant in the 5–60 cm layer, and then significantly decreased in the 60–100 cm layer by a rough amount of −0.2 Mg N ha−1 (Table 3). In contrast to MaiEx and SoyEx, among the two NTR-Cs crop rotation systems, NTR2-Cs significantly (p<0.05) increased TN stock by 0.10 Mg N ha−1 at 0–5 cm, whereas the significant increase in TN stock was detected in the 0–5, 5–10, and 20–40 cm layers at 0.10, 0.10, and 0.3 Mg N ha−1, respectively, under NTR1-Cs. Unlike, MaiEx and SoyEx, there was no significant decrease in TN stock in the subsoil layers under the NTR-Cs (Table 3).
When compared to 2011, in 2021, the TN stock of MaiEx and SoyEx under the NTM system increased significantly (p<0.05) in the topsoil (0–5 cm) by 0.40 and 0.20 Mg N ha−1 under NTM-Mz and NTM-Sb, respectively. However, significant (p<0.05) decreases in TN stock were detected in the subsoils under NTM-Mz at −0.40 Mg N ha−1 in the 40–60 cm layer and at −0.30 to −0.40 Mg N ha−1 in the 60–100 cm layer under NTM-Sb (Table 3). In the case of CasEx, TN stock in the NTM-Cs soil remained constant in the top 0–10 cm and then significantly decreased from −0.3 to −0.5 Mg N ha−1 in the 10–100 cm layer (Table 3).
For the CTM of all the experiments, from 2011 to 2021, TN stock in the topsoil layers remained stable, whereas losses were observed in the layers below 20 cm. CTM-Sb significantly (p<0.05) increased TN stock by 0.20 Mg N ha−1 in the 10–20 cm layer and then remained constant below 20 cm, with a significant (p<0.05) reduction of −0.20 Mg N ha−1 being detected in the 60–80 cm layer (Table 3). In CTM-Mz, TN stock did not change in the 0–40 cm layer but significantly declined between −0.20 to −0.30 Mg N ha−1 from 40 to 100 cm (Table 3). In CTM-Cs soil, TN stock did not change in the top 0–20 cm but significantly decreased from −0.20 to −0.30 Mg N ha−1 in the 20–100 cm layer (Table 3).
Measured over the whole profile (0–100 cm), over the past decade, the TN stock under NTR systems in all the experiments remained stable (Table 3). Mono-cropping of soybean and cassava under NT systems (NTM-Sb and NTM-Cs) caused a significant (p<0.05) reduction in TN stock at annual depletion rates of −0.10 and −0.17 Mg N ha−1 yr−1, respectively, while nearly a decade of upland rice mono-cropping and then the recent shift to maize under the NTM system (NTM-Mz) did not change TN stock (Table 3). In the case of mono-cropping of main crops under CT, the TN stock under CTM-Cs decreased significantly (p<0.05) at a rate of −0.11 Mg N ha−1 yr−1. The TN stock of soil under CTM-Sb remained stable, while the CTM-Mz showed a depletion trend at a rate of −0.11 Mg N ha−1 yr−1, although this was non-significant (Table 3).
Table 3Mean TN stock in 2021 and TN stock change rate between 2021 and 2011.
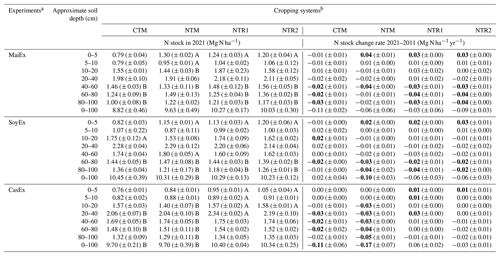
a MaiEx: maize-based experiment; SoyEx: soybean-based experiment; and CasEx: cassava-based experiment. b CTM: mono-cropping of the main crops under conventional tillage; NTM: mono-cropping of the main crops under no-till mulch-based cropping systems with the use of cover crops, and NTR1 and NTR2 refer to bi-annual rotation of the main crops under no-till mulch-based cropping systems with the use of cover crops as described in Table 1. Different uppercase letters accompanying the values indicate significant difference within the same treatment between 2011 and 2021 (diachronic) at the same soil depth at p<0.05 (Tukey's test). Values of TN stock change rates in bold indicate that changes for a given treatment and depth between 2021 and 2011 are significantly different from 0 at p<0.05 (Tukey's test). Positive values of TN stock change rate indicate N accumulation; negative values indicate N loss. Values in the parentheses indicate standard errors (n=3).
3.3 Impact of cropping systems on C and N stocks in size fractions
3.3.1 C stock in size fractions
In this diachronic study, over the 10-year period, the stocks of C-POM and C-MAOM were significantly (p<0.05) influenced by all the treatments. However, the effects varied across cropping systems and the experiments (Fig. 3, Tables S6 and S7).
The data showed that C-POM stock in 2021 increased significantly (p<0.05) in the surface layers (0–10 cm) under all the NT systems in MaiEx and SoyEx, but this was not the case in CasEx (Fig. 3b, d, and f). The annual accumulation rates of C-POM stock in MaiEx and SoyEx were similar, with a range of approximately 0.15 and 0.04 Mg C ha−1 yr−1 under the NTM system and 0.10 and 0.03 Mg C ha−1 yr−1 under the NTR systems (average of NTR1 and NTR2) in the 0–5 and 5–10 cm layers, respectively. This suggested the consequence of the annual biomass inputs that were left on the soil surface under all the NT systems over the experimental period (Table 1). Although the significant increase in C-POM stock was also detected under CTM in the tilled layers (5–20 cm) in MaiEx and SoyEx, at annual accumulation rates of only 0.02 Mg C ha−1 yr−1 across the two soil depths (5–10 and 10–20 cm), this is relatively low when compared with NT systems (Fig. 3b and d).
In a trend similar to that of C-POM, the C-MAOM stock increased significantly (p<0.05) in the topsoil depths under all the NT systems in MaiEx and SoyEx in 2021. In MaiEx, the annual accumulation rates were similar between NTM-Mz and NTR-Mz, with rates of 0.33 and 0.15 Mg C ha−1 yr−1 in the 0–5 and 5–10 cm layers, respectively (Fig. 3b). In SoyEx, the significant increases in C-MAOM stock under all the NT systems were observed down to 20 cm, with approximate annual accumulation rates of 0.20, 0.15, and 0.10 Mg C ha−1 yr−1 in the 0–5, 5–10, and 10–20 cm layers, respectively (Fig. 3d). In CasEx, despite the fact that the C-POM stock remained constant over the past decade, the C-MAOM stock increased significantly (p<0.05) down to 40 cm under all the NT systems in 2021, with similar accumulation rates from 0.09 to 0.26 Mg C ha−1 yr−1 at the 0–40 cm depths (Fig 3f).
Under CTM in 2021, an increase in C-MAOM stock was observed in the tilled layers across all experiments (Fig. 3b, d, and f). Specifically, in the MaiEx experiment, significant differences (p<0.05) in C-MAOM stock between 2011 and 2021 were found in the 5–10 cm and 10–20 cm layers, with annual accumulation rates of 0.10 and 0.23 Mg C ha−1 yr−1, respectively (Fig. 3b). In the case of SoyEx, a significant increase in C-MAOM stock was only detected in the 10–20 cm layer, with an annual accumulation rate of 0.11 Mg C ha−1 yr−1 (Fig. 3d). Meanwhile, in the CasEx experiment, the C-MAOM stock showed a significant annual increase at a rate of 0.05 Mg C ha−1 yr−1 across the topsoil at 0–20 cm (Fig. 3f).
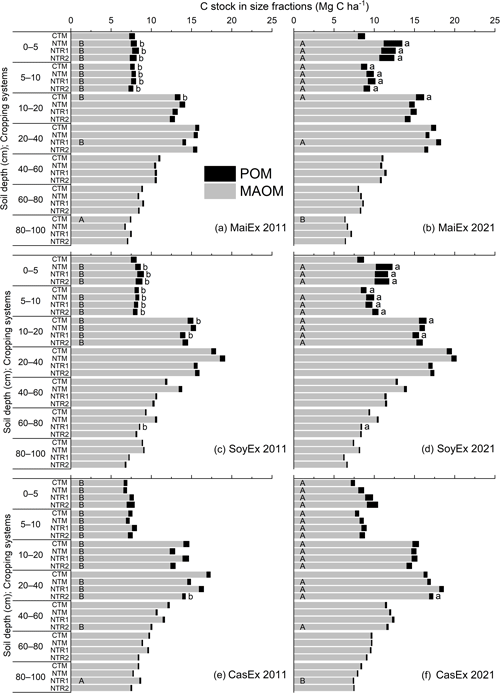
Figure 3Carbon stock in mineral-associated and particulate organic matter (MAOM and POM) fractions across the whole profile (0–100 cm) in 2011 and 2021 under different treatments and experiments. CTM: mono-cropping of the main crops under conventional tillage; NTM: mono-cropping of the main crops under no-till mulch-based cropping systems with the use of cover crops, and NTR1 and NTR2 refer to bi-annual rotation of the main crops under no-till mulch-based cropping systems with the use of cover crops as described in Table 1. The uppercase letters on the bars indicate a significant difference (Tukey's test; p<0.05) of the C stock in MAOM between 2011 and 2021 in the same treatment and at the same soil depth, while the lowercase letters in front of the bars indicate a significant difference of the C stock in POM between 2011 and 2021 in the same treatment and at the same soil depth.
3.3.2 N stock in size fractions
Over the past decade (2011–2021), cropping systems had varying effects on the stocks of N-POM and N-MAOM across soil depths and experiments (Fig. 4, Tables S8 and S9). In 2021, N-POM stock increased significantly (p<0.05) in the topsoil (0–10 cm) under all the NT systems in MaiEx and SoyEx, with similar amounts of 0.10 and 0.01 Mg N ha−1 in the 0–5 and 5–10 cm layers, respectively (Fig. 4b and d). Below 10 cm, N-POM stock remained constant under all the NT systems in both experiments, except for the depletion trend found under an NTR system, particularly under NTR2-Mz below 40 cm and NTR2-Sb below 60 cm (Fig. 4b and d). In contrast to MaiEx and SoyEx, in CasEx, none of NT systems increased N-POM stock in the topsoils, but NTM-Cs and NTR-Cs systems depleted it significantly (p<0.05) below 20 cm (Fig. 4f).
In 2021, mono-cropping of soybean under conventional tillage (CTM-Sb) significantly accumulated N-POM stock in the tilled layers (5–10 and 10–20 cm) by an amount of 0.01 Mg N ha−1 across the two layers, but the significant depletion (p<0.05) at a similar amount was observed below 40 cm (Fig. 4d). Mono-cropping of upland rice over a decade and the recent shift to maize under conventional tillage (CTM-Mz) did not change the N-POM stock across the soil profile (Fig. 4b), whereas the N-POM stock under CTM-Cs soil remained stable in the top 20 cm but declined significantly by −0.01 Mg N ha−1 from 20 to 60 cm (Fig. 4e and f).
Surprisingly, from 2011 to 2021, none of the tillage or cropping systems increased N-MAOM stock but rather decreased it under varying soil depths and experiments (Fig. 4). In MaiEx and SoyEx, N-MAOM stock remained unchanging under the NTM system (i.e. NTM-Mz and NTM-Sb) from 0 to 40 cm but declined significantly (p<0.05) below 40 cm, with the rate ranging from −0.036 to −0.063 Mg N ha−1 yr−1 (Fig. 4a and c). Under NTR-Mz and NTR-Sb, the significant decrease in N-MAOM stock was detected below 5 cm to subsoil layers, but this was inconsistent between the two NTR systems (NTR1 and NTR2) and soil depths, with depletion rates ranging from −0.023 Mg N ha−1 yr−1 in the soil near-surface to −0.140 Mg N ha−1 yr−1 at the bottom of the soil profile (Fig. 4a and c). In CasEx, the N-MAOM stock in the surface layer (0–5 cm) did not change under all the NT systems (NTM-Cs, NTR1-Cs, and NTR2-Cs) but decreased significantly (p<0.05) below 5 cm, with the annual depletion rates ranging from −0.009 to Mg N ha−1 yr−1 in the 5 cm layer to −0.111 Mg N ha−1 yr−1 in the subsoil profile (Fig. 4e and f).
In 2021, the N-MAOM stock of the CTM-Mz soil remained steady in the 0–40 cm layer, whereas depletion was detected from 40 to 100 cm at rates ranging from −0.032 to −0.058 Mg N ha−1 yr−1 (Fig. 4a and b). CTM-Sb did not preserve N-MAOM stock even in tilled layers over the past 10 years but depleted it significantly below 5 cm to subsoil depths at rates of −0.016 to −0.073 Mg N ha−1 yr−1 (Fig. 4c and d), while a significant decrease in N-MAOM stock was observed throughout the soil profile (0–100 cm), with a depletion of −0.013 to −0.081 Mg N ha−1 yr−1 (Fig. 4e and f).
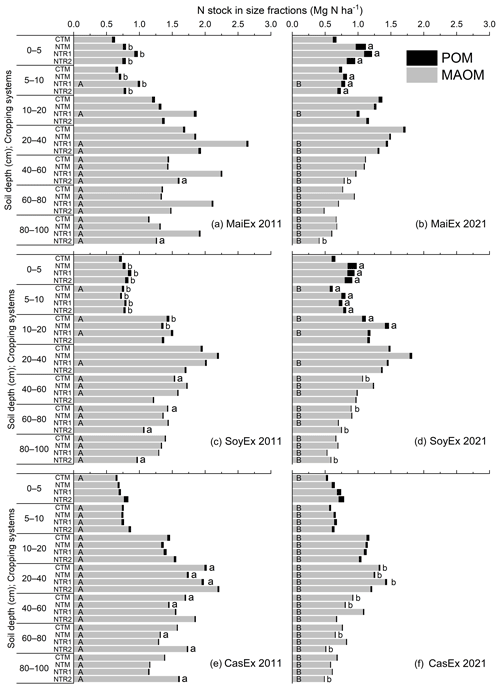
Figure 4TN stock in mineral-associated and particulate organic matter (MAOM and POM) fractions across the whole profile (0–100 cm) in 2011 and 2021 under different treatments and experiments. CTM: mono-cropping of the main crops under conventional tillage; NTM: mono-cropping of the main crops under no-till mulch-based cropping systems with the use of cover crops, and NTR1 and NTR2 refer to bi-annual rotation of the main crops under no-till mulch-based cropping systems with the use of cover crops as described in Table 1. The uppercase letters on the bars indicate a significant difference (Tukey's test; p<0.05) of TN stock in MAOM between 2011 and 2021 in the same treatment and at the same soil depth, while the lowercase letters in front of the bars indicate a significant difference of TN stock in POM between 2011 and 2021 in the same treatment and at the same soil depth.
4.1 Change in SOC stock
Despite the contrasting effects among the NT systems and the experiments, our study showed that adopting NT systems with the use of cover crops in the long term significantly increased SOC stock (Table 2). Several studies reported that long-term NT adoption accumulated SOC stock on the surface soils only, but the stock did not differ from that of CT when considering the whole soil profile (Blanco-Canqui and Lal, 2008; Luo et al., 2010; Blanco-Canqui et al., 2011; Du et al., 2017; Xiao et al., 2020). For example, in a recent meta-analysis from 86 studies covering a range of crop productions across the world, Xiao et al. (2020) found that NT systems significantly accumulated the SOC stock only in the top 0–5 cm, and no significant change was found below 5 cm. Across climatic conditions, soil types, and various cropping systems in China, based on 95 comparisons between NT and CT, adopting NT led to an increase in SOC stock by 3.8 % in the upper-20 cm layer but led to a decrease in SOC in the 30–40 cm layer (Du et al., 2017). Similarly, from a systematic review of global data from 69 paired experiments, Luo et al. (2010) reported that long-term NT adoption only significantly affected SOC stock in the top 0–10 cm but not down to 40 cm depth. The authors also reported that increasing crop species diversity resulted in a lower SOC accumulation in the surface and a greater SOC loss in deeper layers.
SOC stock changes reported under NT systems may differ according to climate, soil type, and cropping systems (Paustian et al., 1997; Six et al., 2002; Bayer et al., 2006; Ogle et al., 2012; Virto et al., 2012). Soils in a tropical climate require diversified and large amounts of C inputs for NT viability due to fast residue decomposition and difficulty in maintaining soil cover (Séguy et al., 2006; Castro et al., 2015).
In the same experiments as in our study, Hok et al. (2015) reported that NT systems with diverse crop species significantly accumulated SOC at the surface (0–5 cm) after 4 years of NT adoption. Our study revealed that NT systems significantly increased SOC stock, although there was variability among the NT systems and across the three experiments in terms of the accumulation rates in the subsoil layers (Table 2). Considering the cumulative SOC stock, all the NT systems significantly (p<0.05) increased cumulative SOC stock across the whole soil profile in MaiEx and CasEx. In SoyEx, a significant increase in cumulative SOC stock was limited to the top 0–20 cm under NTM-Sb, whereas NTR-Sb had significantly accumulating SOC stock from 0 to 80 cm depths (Table S10).
Consistently with our findings, with the intensive NT systems and high C inputs retained in the soils, other studies reported that long-term NT with the use of cover crops increased SOC stock beyond the surface and the whole soil profile (Diekow et al., 2005; Boddey et al., 2010; Olson et al., 2014). From three long-term experiments (15–26 years) on Ferralsols in southern Brazil, no tillage with intensive cropping systems of maize and soybean production increased SOC, with annual accumulation rates between 0.04 and 0.88 Mg ha−1 at 0–30 cm and from 0.48 to 1.53 Mg ha−1 yr−1 at 0–100 cm (Boddey et al., 2010). After 12 years of NT adoption with the use of cover crops for soybean and maize rotation in a humid continental sloping land in Illinois, USA, SOC stock recovered from its initial SOC loss under CT before the experiment implementation, with accumulation rates of 0.42, 0.78, and 1.21 Mg C ha−1 yr−1 at 0–15, 15–75, and 0–75 cm, respectively (Olson et al., 2014).
SOC storage and stabilization could be explained by several processes: (i) continuous supplies of large quantities and diverse qualities of plant biomass-C inputs into the soil (Sá et al., 2014); (ii) the transformation of this biomass-C by microbial communities into various organic C forms (Frasier et al., 2016; Schmidt et al., 2019); (iii) the stabilization of newly derived C by physical protection, binding with organo-mineral particles, and biochemical stabilization through the formation of recalcitrant soil organic matter (Six et al., 2002); and (iv) distribution of SOC over the soil profile through biological processes from root systems (Lorenz and Lal, 2005) and soil fauna (Lavelle et al., 2016).
In NT systems, multiple crop species were sown in the same unit area of land through the rotation of cash crops and the use of cover crops by intercropping or during the fallow period, producing a large quantity and diverse quality of C inputs aboveground but also belowground that were retained in the soils. In our experiments, the annual biomass-C inputs retained in the NT soils ranged from 3.61 to 6.07 Mg ha−1 yr−1 versus 1.36 to 2.20 Mg ha−1 yr−1 under CTM (Table 1). In a clayey Oxisol of Brazil, a 16-year-old experiment revealed that NT was more effective than CT at converting biomass-C inputs into SOC, with a C conversion ratio at 0–40 cm depth of 0.35 compared to 0.07 under NT and CT, respectively (Sá et al., 2014). In addition, integration of cover crops into the crop production system led to a significant increase in SOC. From the observation of 139 plots at 37 sites in the tropics and temperate zones with diverse soil types, Poeplau and Don (2015) reported that the use of cover crops led to an average SOC accumulation rate of 0.32 Mg C ha−1 yr−1 at 22 cm depth. The association of tropical legume cover crops in maize production led to increased SOC stock in the surface, as well as throughout the whole soil profile. Diekow et al. (2005) found that the SOC accumulation rate in the legume-based cropping systems was 0.83 and 1.42 Mg C ha−1 yr−1 in the 0–17.5 and 0–107.5 cm layers, respectively, after 17 years of NT adoption in a Brazilian Acrisol. From a 30-year-old experiment in a Brazilian Acrisol, legume cover crops were twice as effective at storing C as mineral N fertilization, with 1 kg of residue C input being transformed into 0.15 kg of SOC (Veloso et al., 2018).
Considering the challenges faced by smallholder farmers in Cambodia with low financial resources and/or a high level of indebtedness, the main strategy should focus on enhancing nutrient cycling through continuous biomass-C inputs under no-till cropping systems together with a combination of actions to reduce nutrient removal from cassava fields through the non-removal of leaves and of a proportion of stalks, which may also help to reduce the impact of nutrient deficiencies. In addition, the tolerance of cassava in relation to acidic soil, its ability to grow in depleted and degraded soils as a result of the occurrence and synergistic effects of arbuscular mycorrhizal fungi (Howeler et al., 1982; Howeler and Sieverding, 1983) and plant-growth-promoting rhizobacteria (Balota et al., 1999), and its nutrient-recycling ability by means of leaf litter and when the stalks are not used as planting materials and are kept in the field could be used to improve soil and cropping-system sustainability (Fermont et al., 2008). This possible use by farmers of cassava cropping systems as a strategy for regenerating soil fertility was also emphasized by Saïdou et al. (2004) and Adjei-Nsiah et al. (2007) in Benin and Ghana, respectively.
Long-term NT adoption has been shown to significantly improve soil structure, soil porosity, and pore connectivity (Cooper et al., 2021), contributing to the improvement of water infiltration, gas exchanges and microbial activities, and root development to deeper soil profiles (Rosolem et al., 2016). In addition, the aerobic conditions of soil aggregates would enhance SOC stability in unsaturated soils (Zhang et al., 2021). Sisti et al. (2004) showed that increased C accumulation in NT soil below 30 cm depth could be explained by greater root density when compared with CT. Another possibility is that organic residues from upper layers were transported downward by soil meso- and macro-fauna organisms, which could have been favoured by better environmental conditions provided by the continuous C flow and soil structure enhancement under NT systems (Lavelle et al., 2016).
In our study, the SOC stock in the whole soil profile (0–100 cm) under CTM and for the three experiments remained stable, which could be attributed to the fully retained crop residues (i.e. mung bean, rice, and maize) in MaiEx and (i.e. sesame and soybean) in SoyEx and to the partially retained cassava's fallen leaves and stalks in CasEx (Table 1), indicating that it reached a new SOC equilibrium. The high clay content also contributed to the stabilization of the MAOC that, in 2021, accounted for 97.2 % of the carbon stock along the soil profile.
Under a synchronic approach, considering CTM as the reference, the SOC stock change rates in 2021 under NT systems ranged from 0.13 to 0.60, −0.50 to 0.43, and 0.10 to 0.46 Mg C ha−1 yr−1 in MaiEx, SoyEx, and CasEx, respectively (Fig. 5). When compared with the diachronic approach, this corresponds to an underestimation of 146 % to 536 %, 51 % to 347 %, and 51 % to 997 % in MaiEx, SoyEx, and CasEx, respectively (Table 2). In Brazil, from on-farm assessments of the SOC dynamics under long-term NT systems in tropical heavy clayey soils, Neto et al. (2010) and Junior et al. (2013) reported that a synchronic approach led to biased annual SOC accumulation rates under NT systems when compared with a diachronic approach. The main factors associated with the errors could be the underlying heterogeneities of the soil conditions prior to the conversion to NT systems that are hard to capture despite all the steps of the methodologically precautious measurements being implemented properly (Neto et al., 2010; Junior et al., 2013). Our findings clearly emphasize the importance of the diachronic approach in accurately estimating the effects of long-term NT systems on SOC storage.
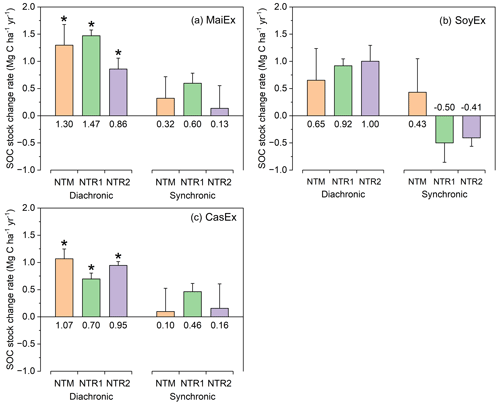
Figure 5Comparison between the diachronic and synchronic approaches used to estimate SOC stock change rate (0–100 cm) from 2011 to 2021 under NT systems in the tropical red Oxisol of Cambodia (n=3; error bars = SE). (a) MaiEx (maize-based trial), (b) SoyEx (soybean-based trial), and (c) CasEx (cassava-based experiments). NTM: mono-cropping of the main crops under no-till mulch-based cropping systems with the use of cover crops; NTR1 and NTR2 refer to bi-annual rotation of the main crops under no-till mulch-based cropping systems with the use of cover crops as described in Table 1. The stock change rates using a diachronic approach were calculated by subtracting the stock of the same treatment in 2021 from the stock in 2011 and dividing by the number of years between the first and second samplings (10 years), while the stock change rates of NT systems in 2021 using a synchronic approach were calculated by subtracting the stock of each NT treatment from the stock of CTM in 2021, considering the control, and dividing by the number of years between the first and second samplings (10 years). Note that (*) indicates a significant difference (Tukey's test; p<0.05) in SOC stock between 2011 and 2021. Positive values indicate SOC stock accumulation; negative values indicate SOC loss.
4.2 Change in N stock
In addition to increasing SOC stock in the surface and throughout the whole soil profile, Diekow et al. (2005) found that soil TN stock was significantly increased by an average of 27 % at the surface (0–17.5 cm) and by 6 % throughout the whole profile (0–107.5 cm) after 17 years of NT maize and tropical legume intercropping and N fertilization compared with its original state under the native grassland of Brazilian Acrisol. Sá et al. (2014) reported a significant correlation (R2=0.89, p<0.0002) between soil N and SOC stock accumulation. Each unit of N stock accumulation contributed to the sequestration of 10.2 Mg C ha−1 in the top 0–10 cm under long-term (16-year) continuous NT maize-based production of Brazilian Oxisol.
However, the diachronic assessment in our study showed that TN stock under NT systems significantly increased in the topsoil (0–5 cm) only in MaiEx and SoyEx, while the stock remained stable in CasEx (Table 3). The significant decline in TN stock under NT systems, although with variability across the NT systems and the experiments, was detected below 20 cm. When considering the whole profile (0–100 cm), significant depletion of N stock was observed under the NT mono-cropping systems, with a loss rate at −0.10 and −0.17 Mg N ha−1 yr−1 in SoyEx (NTM-Sb) and CasEx (NTM-Cs), respectively. Under NT crop rotation systems, despite being non-significant, TN stock tended to decrease across the three experiments, with a depletion rate ranging from −0.03 to −0.09 Mg N ha−1 yr−1 (Table 3).
The depletion of TN stock under NT was reported from short-term (Wuaden et al., 2020) to longer-term NT adoption (Delgado, 2023). From a short-term (5-year) conversion of native grassland to cropland under NT adoption with double cropping, with maize as a cash crop followed by black oat as a cover crop in Brazil's Rhodic , NT soils had significant losses of soil total N in comparison with the original stocks under grassland conditions throughout the soil profile, with the exception of the 0–5 and 10–20 cm soil layers. Considering the whole profile (0–60 cm), soil total N was depleted by −1.7 Mg N ha−1, which is equivalent to an annual loss rate of −0.34 Mg N ha−1 yr−1 after 5 years of grassland conversion to NT (Wuaden et al., 2020). Results from a 12-year experiment in the US (0–120 cm depth) in an irrigated NT continuous maize rotation where mineral N was applied at different rates indicated that even NT could potentially have significant net N loss, with an average loss of -15 kg N ha−1 yr−1 in the top 30 cm of soil regardless of N application rate (Delgado, 2023).
In our study, it is a rather surprising finding to observe an increase in SOC and a simultaneous soil TN depletion. Associating legume cover crops in the cropping system did not enhance soil N through biological N fixation (Rosolem et al., 2016). In general, N is an important factor contributing to SOC storage (De Vries, 2014; Kirkby et al., 2014). Nutrient reserves are among other factors that determine soil C storage capacity (Lal, 2018). Therefore, more studies on nutrient availability and their stoichiometric relationship, including in deeper layers (> 100 cm), as well as on the N use efficiency and N cycling, are needed to understand the driving mechanisms of the N dynamics under these NT systems.
Nitrogen uptake and/or N priming effects from the cover crops, among other factors, could possibly have resulted in N loss. Priming effects are short-term changes in the turnover of soil N caused by the addition of organic or mineral fertilizer, the mechanical treatment of soil, its drying and rewetting (Kuzyakov et al., 2000), and the exudation of organic substances in the rhizosphere by living plants (Kuzyakov, 2002). These effects can occur immediately or very shortly after the addition of a specific substance to the soil and are larger in soils rich in C and N than in poor soils (Kuzyakov et al., 2000). In our experiments, under NT systems, the soils are protected year-round by the cover crops established through association with or succession following the main crops (maize, soybean, and cassava) and that continue to grow after the main crop harvest. Several species of drought-tolerant and fast-growing cover crops (stylo, Brachiaria, cowpea, sorghum, pearl millet, and sunn hemp), which are commonly used in our experiments singly or as a mixture (Table 1), are good examples of crops that remain green throughout the dry season with root exudates that may have enhanced the priming effect. In addition, the symbiotic relationship between the cover crops and rhizobia during the dry season could also be low due to low soil moisture content, therefore resulting in high N uptake from the soil by those cover crops. Their drought-tolerant characteristics allow these species to cross the dry season, even with little or no rain for more than 4 months in the dry season. Their fast-growing characteristics, along with the species diversity, produced a large amount of biomass annually and were retained in the soil at the termination of the cultivation of the main crops (Table 1), which may create conditions for the effects of N uptake or N priming to happen. Measurement of N content and the estimation of biological nitrogen fixation by the legume cover crops using the 15N isotopic technique should be conducted to better understand N dynamics in the different systems.
To date, few studies on the impact of NT systems on N gains or N losses, N use efficiency, and changes in soil N have been conducted, and the results vary depending on the period of NT adoption and sampling depths (Congreves et al., 2017; Delgado, 2023). Further research is needed to understand the driving mechanism of the N dynamics under NT systems by considering deeper layers (> 100 cm) to make informed decisions regarding sustainable soil fertility management and crop production systems. Positive accumulation rates of SOC stock, recorded under NT systems, could not be sustained in the long term as the depletion of the TN stock may lead to nutrient scarcity of other nutrients (P, S, Ca2+, and Mg2+), which constitutes the driving force limiting SOC accumulation. Further analysis is needed to assess the coming changes in SOC and N stocks along with the content of nutrients and its potential impact on the rate of SOC accumulation or depletion (Kirkby et al., 2013).
4.3 Carbon and N in size fractions and stabilization processes in NT systems
Particulate organic matter (POM) and mineral-associated organic matter (MAOM) are the two main fractions of the SOC pools. They differ in terms of their physical and chemical characteristics, as well as in their turnover rates. POM is more sensitive to soil tillage and land use than MAOM and total SOC (Blanco-Moure et al., 2013; Kan et al., 2021). In all the experiments, NT systems significantly increased C in both POM and MAOM fractions in the topsoil layer (Fig. 3). These increases could be attributed to the continuous supply of large amounts of and the diversity in biomass-C inputs into the soil surface, as well as to the diversity of the root systems along with the low level of soil disturbance under NT systems (Sá et al., 2014; Briedis et al., 2018).
During the decomposition process, microbial communities use the rapidly decomposable materials as energy sources, while the recalcitrant and other labile compound materials act as the glue to bind soil mineral particles together (Witzgall et al., 2021). This process is a pathway for the formation of soil micro-aggregates (Bot and Benites, 2005). The continuous supply of biomass-C inputs to the soil, associated with microbial decomposition without soil mechanical disturbance, creates a favourable environment for the emergence of soil macro-aggregates (Crews and Rumsey, 2017). Organic carbon inside soil aggregates is physically protected from microbial oxidation, as well as being strongly associated with the organo-minerals, leading to SOC stabilization over time (Powlson et al., 1987; Lützow et al., 2006). In the same experiments as in our study but after 3 years of NT adoption, Hok et al. (2021) reported that soil aggregation was one of the main stabilization mechanisms, providing physical protection to the newly derived C in the soil micro-aggregates protected by macro-aggregates. From our knowledge of the literature, the high SOC accumulation rate recorded under cassava-based NT cropping systems is relatively unique, and, in addition to the residues of cover crops and maize under the bi-annual crop rotation system, the nature of the cassava residues that were retained in the field with high cellulose and lignin contents may explain this result (Veiga et al., 2016).
From an incubation of labelled litter, Witzgall et al. (2021) found that the occlusion of organic matter into aggregates and the formation of organo-mineral associations occur concurrently on fresh litter surfaces regardless of soil structure. In addition, the increase in C-MAOM is attributed to C transfer from POM and other labile C pools. Over time, these compounds are transferred to more stable pools, creating associations with mineral colloids, with MAOM being more stabilized (Briedis et al., 2018). Rosolem et al. (2016) conducted 3-year successive experiments to assess the aboveground and belowground effects of a wide range of tropical grasses and legume cover crops, which were the same species that were used under the NT systems in our experiments, in combination with no-till soybean-based cropping systems in Brazilian tropical clayey Rhodic Ferralsol, on total organic C and N stocks and on POM fraction. They reported that the presence of C4 deep-root grass cover crops during the fallow period significantly increased total organic C and POM. Furthermore, legume cover crops contributed to maintaining the C N ratio in the topsoil layers, which could keep increasing C over time. Besides the aboveground biomass, the root systems of cover crops are also important C inputs for SOC accumulation due to their capacity to grow deeper in the soil profile, some during the dry season, thereby exploring large volumes of soil (Rosolem et al., 2016; Sokol et al., 2019), releasing large quantities of root exudates, and recycling nutrients (Rosolem et al., 2005). The increase in C stock in POM and MAOM shows that combining NT systems with the use of cover crops is a key strategy to promote both SOC storage and long-term SOC stabilization.
In contrast to C-POM and C-MAOM, although the significant increase in N-POM stock in the top 0–10 cm was observed under all the NT systems in MaiEx and SoyEx, the depletion of N-MAOM stock was observed below 5 cm in CasEx and below 40 cm in MaiEx and SoyEx. This raises questions about the N dynamics and N supply through the use of mineral fertilizers, as well as N fixation through the use of legume crops in the NT cropping systems. Therefore, there is a need to conduct further research on N use efficiency, N cycles, and nutrient availability and their stoichiometric relationship by considering deeper layers (> 100 cm) to understand the mechanism driving N loss under NT systems in these long-term experiments.
The present study showed that, over 10 years, the effects of NT systems on SOC and TN stocks and fractions varied across the three NT systems and the experiments. All the NT cropping systems significantly increased SOC stock in the surface layers in SoyEx and in deeper soil layers under MaiEx and CasEx. When considering the whole profile (0–100 cm), the annual SOC accumulation rates in NT systems ranged from 0.86 to 1.47 and from 0.70 to 1.07 Mg C ha−1 yr−1 in MaiEx and CasEx, respectively. Similarly, under all NT systems, increases in C-POM and C-MAOM stocks were observed in the topsoil layers in MaiEx and SoyEx, and increases were observed in C-MAOM stock in the 0–40 cm layer in CasEx. However, under all the NT systems, N-POM stock only increased in the surface (0–10 cm) layer, but N-MAOM stock decreased below 5 cm in CasEx and below 40 cm in MaiEx and SoyEx.
Overall, our findings reveal that diachronic sampling is crucial for proper measurements of the impacts of NT systems on SOC dynamics with time. Long-term adoption of NT cropping systems accompanied by diversified crop and cover crop species significantly increased SOC stock and fractions in the tropical red Oxisol of Cambodia. The study highlights the potential of NT cropping systems for SOC accumulation and stabilization over time, even for cassava, which is known to induce soil degradation, but raises questions about soil N dynamics. Further research on the N dynamics is needed to understand the mechanism driving N loss in NT systems in order to make informed decisions regarding sustainable soil fertility management and crop production systems.
All data are freely available on the CIRAD data repository under https://doi.org/10.18167/DVN1/NNBBAQ (Leng et al., 2024).
The supplement related to this article is available online at: https://doi.org/10.5194/soil-10-699-2024-supplement.
VL co-established and managed the experiments, carried out the fieldwork, managed all sample collection campaigns and laboratory works, and wrote the first draft of the paper. FT co-managed the experiments, provided support to the team for the last 8 years, and raised funds along with GDA/DALRM to sustain these long-term experiments. LT, RC, and FT conceptualized the research; supervised the field operations, analytical procedures, and data computation; and reviewed the paper. VS, PL, LH, JCdMS, and CB gave advice regarding the analytical procedures, data calculation, and improvement of the paper. PM significantly contributed to the implementation of the field operations, sample collection, and lab work. TF performed the statistical analysis. SB raised funds; designed, established, and managed the experiments during the first years; and contributed to the improvement of the paper.
At least one of the (co-)authors is a member of the editorial board of SOIL. The peer-review process was guided by an independent editor, and the authors also have no other competing interests to declare.
Publisher’s note: Copernicus Publications remains neutral with regard to jurisdictional claims made in the text, published maps, institutional affiliations, or any other geographical representation in this paper. While Copernicus Publications makes every effort to include appropriate place names, the final responsibility lies with the authors.
We thank the DALRM/GDA/MAFF for providing access to the experiments and supporting the paperwork authorization for sample shipments to Switzerland. We further acknowledge CIRAD, France, for its strong collaboration with GDA and for continuing to provide the technical support for maintaining these long-term experiments. Additional thanks are given to CARDEC's team, who manages the field operations. Special appreciation to Britta Jahn-Humphrey of the Sustainable Agroecosystem, ETH Zurich, for processing the paperwork and overseeing the measurement of all the SOC and TN.
This research was supported by the Agroecology and Safe Food System Transitions in Southeast Asia (ASSET) project. The project is co-funded by the Agence Française de Développement (grant no. CZZ2453 01B); the European Commission, Directorate-General for the Environment (grant no. CZZ2453 02C); and the Fonds Français pour l’Environnement Mondial (grant no. CZZ2868 01M).
This paper was edited by Ping He and reviewed by two anonymous referees.
ADB: Cambodia Agriculture, Natural Resources, and Rural Development Sector Assessment, Strategy, and Road Map, Asian Development Bank, Manila, Philippines, No. TCS210256-2, https://doi.org/10.22617/TCS210256-2, 2021.
Adjei-Nsiah, S., Kuyper, T. W., Leeuwis, C., Abekoe, M. K., and Giller, K. E.: Evaluating sustainable and profitable cropping sequences with cassava and four legume crops: Effects on soil fertility and maize yields in the forest/savannah transitional agro-ecological zone of Ghana, Field Crops Res., 103, 87–97, https://doi.org/10.1016/j.fcr.2007.05.001, 2007.
Angers, D. A. and Eriksen-Hamel, N. S.: Full-Inversion Tillage and Organic Carbon Distribution in Soil Profiles: A Meta-Analysis, Soil Sci. Soc. Am. J., 72, 1370–1374, https://doi.org/10.2136/sssaj2007.0342, 2008.
Balota, E. L., Lopes, E. S., Hungria, M., and Döbereiner, J.: Occurence of diazotrophic and arbuscular mycorhizal fungi on the cassava crop, Pesq. Agropec. Bras., 34, 1265–1276, https://doi.org/10.1590/S0100-204X1999000700020, 1999.
Barbier, E. B. and Di Falco, S.: Rural Populations, Land Degradation, and Living Standards in Developing Countries, Rev. Env. Econ. Policy, 15, 115–133, https://doi.org/10.1086/713152, 2021.
Barbier, E. B. and Hochard, J. P.: Land degradation and poverty, Nat. Sustain., 1, 623–631, https://doi.org/10.1038/s41893-018-0155-4, 2018.
Bayer, C., Martin-Neto, L., Mielniczuk, J., Pavinato, A., and Dieckow, J.: Carbon sequestration in two Brazilian Cerrado soils under no-till, Soil Till. Res., 86, 237–245, https://doi.org/10.1016/j.still.2005.02.023, 2006.
Beillouin, D., Corbeels, M., Demenois, J., Berre, D., Boyer, A., Fallot, A., Feder, F., and Cardinael, R.: A global meta-analysis of soil organic carbon in the Anthropocene, Nat. Commun., 14, 3700, https://doi.org/10.1038/s41467-023-39338-z, 2023.
Bernoux, M., Feller, C., Cerri, C. C., Eschenbrenner, V., and Cerri, C. E. P.: Soil Carbon Sequestration, in: Soil Erosion And Carbon Dynamics, CRC Press, Boca Raton, 13–22, ISBN-13: 978-1-56670-688-9, 2005.
Blanco-Canqui, H. and Lal, R.: No-Tillage and Soil-Profile Carbon Sequestration: An On-Farm Assessment, Soil Sci. Soc. Am. J., 72, 693–701, https://doi.org/10.2136/sssaj2007.0233, 2008.
Blanco-Canqui, H., Schlegel, A. J., and Heer, W. F.: Soil-profile distribution of carbon and associated properties in no-till along a precipitation gradient in the central Great Plain, Agr. Ecosyst. Environ., 144, 107–116, https://doi.org/10.1016/j.agee.2011.07.004, 2011.
Blanco-Moure, N., Gracia, R., Bielsa, C., and Lopez, M. V.: Long-term no-tillage effects on particulate and mineral-associated soil organic matter under rainfed Mediterranean conditions, Soil Use Manag., 29, 250–259, https://doi.org/10.1111/sum.12039, 2013.
Boddey, R. M., Jantalia, C. P., Conceição, P. C., Zanatta, J. A., Bayer, C., Mielniczuk, J., Dieckow, J., Dos Santos, H. P., Denardin, J. E., Aita, C., Giacomini, S. J., Alves, B. J. R., and Urquiaga, S.: Carbon accumulation at depth in Ferralsols under zero-till subtropical agriculture, Glob. Change Biol., 16, 784–795, https://doi.org/10.1111/j.1365-2486.2009.02020.x, 2010.
Bot, A. and Benites, J.: The importance of soil organic matter: key to drought-resistant soil and sustained food and production, FAO – Food and Agriculture Organization of the United Nations, Rome, 78 pp., ISBN: 92-5-105366-9, 2005.
Briedis, C., De Moraes Sá, J. C., Lal, R., Tivet, F., Franchini, J. C., De Oliveira Ferreira, A., Da Cruz Hartman, D., Schimiguel, R., Bressan, P. T., Inagaki, T. M., Romaniw, J., and Gonçalves, D. R. P.: How does no-till deliver carbon stabilization and saturation in highly weathered soils?, Catena, 163, 13–23, https://doi.org/10.1016/j.catena.2017.12.003, 2018.
CARDI: Annual report 2016, Cambodian Agricultural Research and Development Institue (CARDI), Phnom Penh, Cambodia, 2017.
Castro, G. S. A., Crusciol, C. A. C., Calonego, J. C., and Rosolem, C. A.: Management Impacts on Soil Organic Matter of Tropical Soils, Vadose Zone J., 14, 1–8, https://doi.org/10.2136/vzj2014.07.0093, 2015.
Congreves, K. A., Hooker, D. C., Hayes, A., Verhallen, E. A., and Van Eerd, L. L.: Interaction of long-term nitrogen fertilizer application, crop rotation, and tillage system on soil carbon and nitrogen dynamics, Plant Soil, 410, 113–127, https://doi.org/10.1007/s11104-016-2986-y, 2017.
Cooper, H. V., Sjögersten, S., Lark, R. M., Girkin, N. T., Vane, C. H., Calonego, J. C., Rosolem, C., and Mooney, S. J.: Long-term zero-tillage enhances the protection of soil carbon in tropical agriculture, Europ. J. Soil Sci., 72, 2477–2492, https://doi.org/10.1111/ejss.13111, 2021.
Corbeels, M., Cardinael, R., Naudin, K., Guibert, H., and Torquebiau, E.: The 4 per 1000 goal and soil carbon storage under agroforestry and conservation agriculture systems in sub-Saharan Africa, Soil Till. Res., 188, 16–26, https://doi.org/10.1016/j.still.2018.02.015, 2019.
Craswell, E. T. and Lefroy, R. D. B.: The role and function of organic matter in tropical soils, in: Managing Organic Matter in Tropical Soils: Scope and Limitations, edited by: Martius, C., Tiessen, H., and Vlek, P. L. G., Springer Netherlands, Dordrecht, 7–18, https://doi.org/10.1007/978-94-017-2172-1_2, 2001.
Crews, T. E. and Rumsey, B. E.: What Agriculture Can Learn from Native Ecosystems in Building Soil Organic Matter: A Review, Sustainability, 9, 578, https://doi.org/10.3390/su9040578, 2017.
Delgado, J. A.: Long-term nitrogen balance of an irrigated no-till soil-corn system, Nutr. Cycl. Agroecosyst., 126, 229–243, https://doi.org/10.1007/s10705-023-10287-9, 2023.
De Vries, W.: Nutrients trigger carbon storage, Nat. Clim. Change, 4, 425–426, https://doi.org/10.1038/nclimate2255, 2014.
Diekow, J., Mielniczuk, J., Knicker, H., Bayer, C., Dick, D. P., and Kögel-Knabner, I.: Soil C and N stocks as affected by cropping systems and nitrogen fertilisation in a southern Brazil Acrisol managed under no-tillage for 17 years, Soil Till. Res., 81, 87–95, https://doi.org/10.1016/j.still.2004.05.003, 2005.
Dragović, N. and Vulević, T.: Soil Degradation Processes, Causes, and Assessment Approaches, in: Life on Land, edited by: Leal Filho, W., Azul, A. M., Brandli, L., Lange Salvia, A., and Wall, T., Springer International Publishing, Cham, 1–12, https://doi.org/10.1007/978-3-319-71065-5_86-1, 2020.
Du, Z., Angers, D. A., Ren, T., Zhang, Q., and Li, G.: The effect of no-till on organic C storage in Chinese soils should not be overemphasized: A meta-analysis, Agr. Ecosyst. Environ., 236, 1–11, https://doi.org/10.1016/j.agee.2016.11.007, 2017.
Ellert, B. H. and Bettany, J. R.: Calculation of organic matter and nutrients stored in soils under contrasting management regimes, Can. J. Soil. Sci., 75, 529–538, https://doi.org/10.4141/cjss95-075, 1995.
Fermont, A. M., Van Asten, P. J. A., and Giller, K. E.: Increasing land pressure in East Africa: The changing role of cassava and consequences for sustainability of farming systems, Agr. Ecosyst. Environ., 128, 239–250, https://doi.org/10.1016/j.agee.2008.06.009, 2008.
Fowler, A. F., Basso, B., Millar, N., and Brinton, W. F.: A simple soil mass correction for a more accurate determination of soil carbon stock changes, Sci. Rep., 13, 2242, https://doi.org/10.1038/s41598-023-29289-2, 2023.
Frasier, I., Noellemeyer, E., Figuerola, E., Erijman, L., Permingeat, H., and Quiroga, A.: High quality residues from cover crops favor changes in microbial community and enhance C and N sequestration, Glob. Ecol. Conserv., 6, 242–256, https://doi.org/10.1016/j.gecco.2016.03.009, 2016.
Fujisaki, K., Chevallier, T., Chapuis-Lardy, L., Albrecht, A., Razafimbelo, T., Masse, D., Ndour, Y. B., and Chotte, J.-L.: Soil carbon stock changes in tropical croplands are mainly driven by carbon inputs: A synthesis, Agr. Ecosyst. Environ., 259, 147–158, https://doi.org/10.1016/j.agee.2017.12.008, 2018.
Hok, L., De Moraes Sá, J. C., Boulakia, S., Reyes, M., Leng, V., Kong, R., Tivet, F. E., Briedis, C., Hartman, D., Ferreira, L. A., Magno, T., and Pheav, S.: Short-term conservation agriculture and biomass-C input impacts on soil C dynamics in a savanna ecosystem in Cambodia, Agr. Ecosyst. Environ., 214, 54–67, https://doi.org/10.1016/j.agee.2015.08.013, 2015.
Hok, L., De Moraes Sá, J. C., Reyes, M., Boulakia, S., Tivet, F., Leng, V., Kong, R., Briedis, C., Da Cruz Hartman, D., Ferreira, L. A., Inagaki, T. M., Gonçalves, D. R. P., and Bressan, P. T.: Enzymes and C pools as indicators of C build up in short-term conservation agriculture in a savanna ecosystem in Cambodia, Soil Till. Res., 177, 125–133, https://doi.org/10.1016/j.still.2017.11.015, 2018.
Hok, L., De Moraes Sá, J. C., Boulakia, S., Reyes, M., De Oliveira Ferreira, A., Elie Tivet, F., Saab, S., Auccaise, R., Massao Inagaki, T., Schimiguel, R., Aparecida Ferreira, L., Briedis, C., Santos Canalli, L. B., Kong, R., and Leng, V.: Dynamics of soil aggregate-associated organic carbon based on diversity and high biomass-C input under conservation agriculture in a savanna ecosystem in Cambodia, Catena, 198, 105065, https://doi.org/10.1016/j.catena.2020.105065, 2021.
Howeler, R. H. and Sieverding, E.: Potentials and limitations of mycorrhizal inoculation illustrated by experiments with field-grown cassava, Plant Soil, 75, 245–261, https://doi.org/10.1007/BF02375570, 1983.
Howeler, R. H., Cadavid, L. F., and Burckhardt, E.: Response of cassava to VA mycorrhizal inoculation and phosphorus application in greenhouse and field experiments, Plant Soil, 69, 327–339, https://doi.org/10.1007/BF02372454, 1982.
IUSS Working Group WRB: World reference base for soil resources 2014: international soil classification system for naming soils and creating legends for soil maps, FAO – Food and Agriculture Organization of the United Nations, Rome, World Soil Resources Reports No. 106, ISBN: 978-92-5-108369-7, 2015.
Junior, C. C., Corbeels, M., Bernoux, M., Píccolo, M. C., Siqueira Neto, M., Feigl, B. J., Cerri, C. E. P., Cerri, C. C., Scopel, E., and Lal, R.: Assessing soil carbon storage rates under no-tillage: Comparing the synchronic and diachronic approaches, Soil Till. Res., 134, 207–212, https://doi.org/10.1016/j.still.2013.08.010, 2013.
Kan, Z.-R., Liu, W.-Z., Liu, W.-S., Lal, R., Dang, Y. P., Zhao, X., and Zhang, H.-L.: Mechanisms of soil organic carbon stability and its response to no-till: A global synthesis and perspective, Glob. Change Biol., 28, 693–710, https://doi.org/10.1111/gcb.15968, 2021.
Ken, S., Sasaki, N., Entani, T., Ma, H. O., Thuch, P., and Tsusaka, T. W.: Assessment of the Local Perceptions on the Drivers of Deforestation and Forest Degradation, Agents of Drivers, and Appropriate Activities in Cambodia, Sustainability, 12, 9987, https://doi.org/10.3390/su12239987, 2020.
Kirkby, C. A., Richardson, A. E., Wade, L. J., Batten, G. D., Blanchard, C., and Kirkegaard, J. A.: Carbon-nutrient stoichiometry to increase soil carbon sequestration, Soil Biol. Biochem., 60, 77–86, https://doi.org/10.1016/j.soilbio.2013.01.011, 2013.
Kirkby, C. A., Richardson, A. E., Wade, L. J., Passioura, J. B., Batten, G. D., Blanchard, C., and Kirkegaard, J. A.: Nutrient availability limits carbon sequestration in arable soils, Soil Biol. Biochem., 68, 402–409, https://doi.org/10.1016/j.soilbio.2013.09.032, 2014.
Koun, P., Vernet, P., Filloux, T., Sar, V., Seng, V., Srimongkol, P., Tantachasatid, P., Sen, R., Pheap, S., Tivet, F., and Thoumazeau, A.: Early effects of conservation agriculture on soil organic carbon dynamics of Mollisols in Cambodia, Soil Use Manag., 40, e12922, https://doi.org/10.1111/sum.12922, 2023.
Kuzyakov, Y.: Review: Factors affecting rhizosphere priming effects, Z. Pflanzenernähr. Bodenk., 165, 382–396, 2002.
Kuzyakov, Y., Friedel, J. K., and Stahr, K.: Review of mechanisms and quantification of priming effects, Soil Biol., 32, 1485–1498, https://doi.org/10.1016/S0038-0717(00)00084-5, 2000.
Lal, R.: Restoring Soil Quality to Mitigate Soil Degradation, Sustainability, 7, 5875–5895, https://doi.org/10.3390/su7055875, 2015a.
Lal, R.: Sequestering carbon and increasing productivity by conservation agriculture, J. Soil Water Conserv., 70, 55A–62A, https://doi.org/10.2489/jswc.70.3.55A, 2015b.
Lal, R.: Digging deeper: A holistic perspective of factors affecting soil organic carbon sequestration in agroecosystems, Glob. Change Biol., 24, 3285–3301, https://doi.org/10.1111/gcb.14054, 2018.
Lavallee, J. M., Soong, J. L., and Cotrufo, M. F.: Conceptualizing soil organic matter into particulate and mineral-associated forms to address global change in the 21st century, Glob. Change Biol., 26, 261–273, https://doi.org/10.1111/gcb.14859, 2020.
Lavelle, P., Spain, A., Blouin, M., Brown, G., Decaëns, T., Grimaldi, M., Jiménez, J. J., McKey, D., Mathieu, J., Velasquez, E., and Zangerlé, A.: Ecosystem Engineers in a Self-organized Soil: A Review of Concepts and Future Research Questions, Soil Sci., 181, 91–109, https://doi.org/10.1097/SS.0000000000000155, 2016.
Leng, V., Cardinael, R., Tivet, F. E., Seng, V., Mark, P., Lienhard, P., Filloux, T., Six, J., Hok, L., Boulakia, S., Briedis, C., Sá, D. M. J. C., and Thuriès, L.: Data for “Diachronic assessment of soil organic C and N dynamics under long-term no-till cropping systems in the tropical upland of Cambodia”, CIRAD Dataverse [data set], https://doi.org/10.18167/DVN1/NNBBAQ, 2024.
Li, G., Yu, C., Shen, P., Hou, Y., Ren, Z., Li, N., Liao, Y., Li, T., and Wen, X.: Crop diversification promotes soil aggregation and carbon accumulation in global agroecosystems: A meta-analysis, J. Environ. Manag., 350, 119661, https://doi.org/10.1016/j.jenvman.2023.119661, 2024.
Liang, C., Cheng, G., Wixon, D. L., and Balser, T. C.: An Absorbing Markov Chain approach to understanding the microbial role in soil carbon stabilization, Biogeochemistry, 106, 303–309, https://doi.org/10.1007/s10533-010-9525-3, 2011.
Lienhard, P., Tivet, F., Chabanne, A., Dequiedt, S., Lelièvre, M., Sayphoummie, S., Leudphanane, B., Prévost-Bouré, N. C., Séguy, L., Maron, P.-A., and Ranjard, L.: No-till and cover crops shift soil microbial abundance and diversity in Laos tropical grasslands, Agron. Sustain. Dev., 33, 375–384, https://doi.org/10.1007/s13593-012-0099-4, 2013.
Liu, X., Wu, X., Liang, G., Zhang, M., Li, S., and Zheng, F.: A global meta-analysis of the impacts of no-tillage on soil aggregation and aggregate-associated organic carbon, Land Degrad. Dev., 32, 5292–5305, https://doi.org/10.1002/ldr.4109, 2021.
Lorenz, K. and Lal, R.: The Depth Distribution of Soil Organic Carbon in Relation to Land Use and Management and the Potential of Carbon Sequestration in Subsoil Horizons, in: Advances in Agronomy,Vol. 88, Elsevier, 35–66, https://doi.org/10.1016/S0065-2113(05)88002-2, 2005.
Luo, Z., Wang, E., and Sun, O. J.: Can no-tillage stimulate carbon sequestration in agricultural soils? A meta-analysis of paired experiments, Agr. Ecosyst. Environ., 139, 224–231, https://doi.org/10.1016/j.agee.2010.08.006, 2010.
Lützow, M. V., Kögel-Knabner, I., Ekschmitt, K., Matzner, E., Guggenberger, G., Marschner, B., and Flessa, H.: Stabilization of organic matter in temperate soils: mechanisms and their relevance under different soil conditions – a review, Europ. J. Soil Sci., 57, 426–445, https://doi.org/10.1111/j.1365-2389.2006.00809.x, 2006.
MAFF: Land Degradation Neutrality Targets, https://www.unccd.int/sites/default/files/ldn_targets/Cambodia LDN TSP Country Report.pdf (last access: 11 October 2023), 2018.
Morugán-Coronado, A.: The impact of crop diversification, tillage and fertilization type on soil total microbial, fungal and bacterial abundance: A worldwide meta-analysis of agricultural sites, Agr. Ecosyst. Environ., 329, 107867, https://doi.org/10.1016/j.agee.2022.107867, 2022.
Neto, M. S., Scopel, E., Corbeels, M., Cardoso, A. N., Douzet, J.-M., Feller, C., Piccolo, M. D. C., Cerri, C. C., and Bernoux, M.: Soil carbon stocks under no-tillage mulch-based cropping systems in the Brazilian Cerrado: An on-farm synchronic assessment, Soil Till. Res., 110, 187–195, https://doi.org/10.1016/j.still.2010.07.010, 2010.
Nkonya, E., Mirzabaev, A., and Von Braun, J. (Eds.): Economics of Land Degradation and Improvement – A Global Assessment for Sustainable Development, Springer International Publishing, Cham, Switzerland, ISBN: 978-3-319-19167-6, https://doi.org/10.1007/978-3-319-19168-3, 2016.
Nut, N., Mihara, M., Jeong, J., Ngo, B., Sigua, G., Prasad, P. V. V., and Reyes, M. R.: Land Use and Land Cover Changes and Its Impact on Soil Erosion in Stung Sangkae Catchment of Cambodia, Sustainability, 13, 9276, https://doi.org/10.3390/su13169276, 2021.
Obalum, S. E., Chibuike, G. U., Peth, S., and Ouyang, Y.: Soil organic matter as sole indicator of soil degradation, Environ. Monit. Assess., 189, 176, https://doi.org/10.1007/s10661-017-5881-y, 2017.
Ogle, S. M., Swan, A., and Paustian, K.: No-till management impacts on crop productivity, carbon input and soil carbon sequestration, Agr. Ecosyst. Environ., 149, 37–49, https://doi.org/10.1016/j.agee.2011.12.010, 2012.
Oliveira, F. C. C., Ferreira, G. W. D., Souza, J. L. S., Vieira, M. E. O., and Pedrotti, A.: Soil physical properties and soil organic carbon content in northeast Brazil: long-term tillage systems effects, Sci. Agric., 77, e20180166, https://doi.org/10.1590/1678-992x-2018-0166, 2020.
Olson, K., Ebelhar, S. A., and Lang, J. M.: Long-Term Effects of Cover Crops on Crop Yields, Soil Organic Carbon Stocks and Sequestration, Open J. Soil Sci., 4, 284–292, https://doi.org/10.4236/ojss.2014.48030, 2014.
Paustian, K., Andrén, O., Janzen, H. H., Lal, R., Smith, P., Tian, G., Tiessen, H., Van Noordwijk, M., and Woomer, P. L.: Agricultural soils as a sink to mitigate CO 2 emissions, Soil Use Manag., 13, 230–244, https://doi.org/10.1111/j.1475-2743.1997.tb00594.x, 1997.
Pheap, S., Lefèvre, C., Thoumazeau, A., Leng, V., Boulakia, S., Koy, R., Hok, L., Lienhard, P., Brauman, A., and Tivet, F.: Multi-functional assessment of soil health under Conservation Agriculture in Cambodia, Soil Till. Res., 194, 104349, https://doi.org/10.1016/j.still.2019.104349, 2019.
Poeplau, C. and Don, A.: Carbon sequestration in agricultural soils via cultivation of cover crops – A meta-analysis, Agr. Ecosyst. Environ., 200, 33–41, https://doi.org/10.1016/j.agee.2014.10.024, 2015.
Polyakov, V. and Lal, R.: Modeling soil organic matter dynamics as affected by soil water erosion, Environ. Int., 30, 547–556, https://doi.org/10.1016/j.envint.2003.10.011, 2004.
Powlson, D. S., Prookes, P. C., and Christensen, B. T.: Measurement of soil microbial biomass provides an early indication of changes in total soil organic matter due to straw incorporation, Soil Biol. Biochem., 19, 159–164, https://doi.org/10.1016/0038-0717(87)90076-9, 1987.
Powlson, D. S., Stirling, C. M., Thierfelder, C., White, R. P., and Jat, M. L.: Does conservation agriculture deliver climate change mitigation through soil carbon sequestration in tropical agro-ecosystems?, Agr. Ecosyst. Environ., 220, 164–174, https://doi.org/10.1016/j.agee.2016.01.005, 2016.
Prommer, J., Walker, T. W. N., Wanek, W., Braun, J., Zezula, D., Hu, Y., Hofhansl, F., and Richter, A.: Increased microbial growth, biomass, and turnover drive soil organic carbon accumulation at higher plant diversity, Glob. Change Biol., 26, 669–681, https://doi.org/10.1111/gcb.14777, 2019.
R Core Team: R: A language and environment for statistical computing, R Foundation for Statistical Computing, Vienna, Austria, https://www.R-project.org/ (last access: 4 December 2023), 2023.
Rodrigues, A. T. L., Giacomini, S. J., Dieckow, J., Cherubin, M. R., Sangiovo Ottonelli, A., and Bayer, C.: Carbon saturation deficit and litter quality drive the stabilization of litter-derived C in mineral-associated organic matter in long-term no-till soil, Catena, 219, 106590, https://doi.org/10.1016/j.catena.2022.106590, 2022.
Rosolem, C. A., Li, Y., and Garcia, R. A.: Soil carbon as affected by cover crops under no-till under tropical climate, Soil Use Manag., 32, 495–503, https://doi.org/10.1111/sum.12309, 2016.
Sá, D. M. J. C., Tivet, F., Lal, R., Briedis, C., Hartman, D. C., Dos Santos, J. Z., and Dos Santos, J. B.: Long-term tillage systems impacts on soil C dynamics, soil resilience and agronomic productivity of a Brazilian Oxisol, Soil Till. Res., 136, 38–50, https://doi.org/10.1016/j.still.2013.09.010, 2014.
Saïdou, A., Kuyper, T. W., Kossou, D. K., Tossou, R., and Richards, P.: Sustainable soil fertility management in Benin: learning from farmers, NJAS – Wagen. J. Life Sc., 52, 349–369, https://doi.org/10.1016/S1573-5214(04)80021-6, 2004.
Sar, V.: Biofunctool® Approach Assessing Soil Quality under Conservation Agriculture and Conventional Tillage for Rain- fed Lowland Rice Systems in Cambodia, Int. J. Environ. Rural Dev., 12, 162–171, 2021.
Séguy, L., Bouzinac, S., and Husson, O.: Direct-seeded tropical soil systems with permanent soil cover: Learning from Brazilian experience, in: Biological approaches to sustainable soil systems, edited by: Uphoff, N., Ball, S. A., Fernandes, E., Herren, H., Husson, O., Laing, M., Palm, C., Pretty, J., Sanchez, P., Sanginga, N., and Thies, J., Taylor and Francis, New York, USA, CRC Press, 323–342, ISBN: 978-0-429-13429-6, 2006.
Schmidt, R., Mitchell, J., and Scow, K.: Cover cropping and no-till increase diversity and symbiotroph:saprotroph ratios of soil fungal communities, Soil Biol. Biochem., 129, 99–109, https://doi.org/10.1016/j.soilbio.2018.11.010, 2019.
Shumba, A., Chikowo, R., Thierfelder, C., Corbeels, M., Six, J., and Cardinael, R.: Mulch application as the overarching factor explaining increase in soil organic carbon stocks under conservation agriculture in two 8-year-old experiments in Zimbabwe, SOIL, 10, 151–165, https://doi.org/10.5194/soil-10-151-2024, 2024.
Sisti, C. P. J., Dos Santos, H. P., Kohhann, R., Alves, B. J. R., Urquiaga, S., and Boddey, R. M.: Change in carbon and nitrogen stocks in soil under 13 years of conventional or zero tillage in southern Brazil, Soil Till. Res., 76, 39–58, https://doi.org/10.1016/j.still.2003.08.007, 2004.
Sithole, N. J., Magwaza, L. S., and Thibaud, G. R.: Long-term impact of no-till conservation agriculture and N-fertilizer on soil aggregate stability, infiltration and distribution of C in different size fractions, Soil Till. Res., 190, 147–156, https://doi.org/10.1016/j.still.2019.03.004, 2019.
Six, J., Conant, R. T., Paul, E. A., and Paustian, K.: Stabilization mechanisms of soil organic matter: Implications for C-saturation of soils, Plant Soil, 241, 155–176, 2002.
Six, J., Bossuyt, H., Degryze, S., and Denef, K.: A history of research on the link between (micro)aggregates, soil biota, and soil organic matter dynamics, Soil Till. Res., 79, 7–31, 2004.
Sokol, N. W., Kuebbing, Sara. E., Karlsen-Ayala, E., and Bradford, M. A.: Evidence for the primacy of living root inputs, not root or shoot litter, in forming soil organic carbon, New Phytol., 221, 233–246, https://doi.org/10.1111/nph.15361, 2019.
Sourn, T., Pok, S., Chou, P., Nut, N., Theng, D., and Prasad, P. V. V.: Assessment of Land Use and Land Cover Changes on Soil Erosion Using Remote Sensing, GIS and RUSLE Model: A Case Study of Battambang Province, Cambodia, Sustainability, 14, 4066, https://doi.org/10.3390/su14074066, 2022.
Sourn, T., Pok, S., Chou, P., Nut, N., Theng, D., and Hin, L.: Assessing Land Use and Land Cover (LULC) Change and Factors Affecting Agricultural Land: Case Study in Battambang Province, Cambodia, Res. World Agr. Econ., 4, 41–54, https://doi.org/10.36956/rwae.v4i4.925, 2023.
Stavi, I. and Lal, R.: Achieving Zero Net Land Degradation: Challenges and opportunities, J. Arid Environ., 112, 44–51, https://doi.org/10.1016/j.jaridenv.2014.01.016, 2015.
Suong, M., Chapuis, E., Leng, V., Tivet, F., Waele, D. D., Thi, H. N., and Bellafiore, S.: Impact of a conservation agriculture system on soil characteristics, rice yield, and root-parasitic nematodes in a Cambodian lowland rice field, J. Nematol., 51, 1–15, https://doi.org/10.21307/jofnem-2019-085, 2019.
TerAvest, D., Carpenter-Boggs, L., Thierfelder, C., and Reganold, J. P.: Crop production and soil water management in conservation agriculture, no-till, and conventional tillage systems in Malawi, Agr. Ecosyst. Environ., 212, 285–296, https://doi.org/10.1016/j.agee.2015.07.011, 2015.
Tiecher, T., Gubiani, E., Santanna, M. A., Veloso, M. G., Calegari, A., Canalli, L. B. D. S., Finckh, M. R., Caner, L., and Rheinheimer, D. D. S.: Effect of 26-years of soil tillage systems and winter cover crops on C and N stocks in a Southern Brazilian Oxisol, Rev. Bras. Cienc. Solo, 44, e0200029, https://doi.org/10.36783/18069657rbcs20200029, 2020.
Tiemann, L. K., Grandy, A. S., Atkinson, E. E., Marin-Spiotta, E., and McDaniel, M. D.: Crop rotational diversity enhances belowground communities and functions in an agroecosystem, Ecol. Lett., 18, 761–771, https://doi.org/10.1111/ele.12453, 2015.
Tivet, F., De Moraes Sá, J. C., Lal, R., Briedis, C., Borszowskei, P. R., Dos Santos, J. B., Farias, A., Eurich, G., Hartman, D. D. C., Nadolny Junior, M., Bouzinac, S., and Séguy, L.: Aggregate C depletion by plowing and its restoration by diverse biomass-C inputs under no-till in sub-tropical and tropical regions of Brazil, Soil Till. Res., 126, 203–218, https://doi.org/10.1016/j.still.2012.09.004, 2013.
UNCCD: Country profile of Cambodia. Investing in land degradation neutrality: Making te case, An overview of indicator and assessments, Global mechanism of the UNCCD, Bonn, Germany, https://www.unccd.int/sites/default/files/ldn_targets/2018-12/Cambodia.pdf (last access: 11 September 2023), 2018.
USDA: Soil Taxonomy: A Basic System of Soil Classification for Making and Interpreting Soil Surveys, 2nd Edn., Agriculture Handbook No. 436, U.S. Government Printing Office, Washington, D.C., 1999.
Veiga, J. P. S., Valle, T. L., Feltran, J. C., and Bizzo, W. A.: Characterization and productivity of cassava waste and its use as an energy source, Renew. Energ., 93, 691–699, https://doi.org/10.1016/j.renene.2016.02.078, 2016.
Veloso, M. G., Angers, D. A., Tiecher, T., Giacomini, S., Dieckow, J., and Bayer, C.: High carbon storage in a previously degraded subtropical soil under no-tillage with legume cover crops, Agr. Ecosyst. Environ., 268, 15–23, https://doi.org/10.1016/j.agee.2018.08.024, 2018.
Virto, I., Barré, P., Burlot, A., and Chenu, C.: Carbon input differences as the main factor explaining the variability in soil organic C storage in no-tilled compared to inversion tilled agrosystems, Biogeochemistry, 108, 17–26, https://doi.org/10.1007/s10533-011-9600-4, 2012.
Von Haden, A. C., Yang, W. H., and DeLucia, E. H.: Soils' dirty little secret: Depth-based comparisons can be inadequate for quantifying changes in soil organic carbon and other mineral soil properties, Glob. Change Biol., 26, 3759–3770, https://doi.org/10.1111/gcb.15124, 2020.
Witzgall, K., Vidal, A., Schubert, D. I., Höschen, C., Schweizer, S. A., Buegger, F., Pouteau, V., Chenu, C., and Mueller, C. W.: Particulate organic matter as a functional soil component for persistent soil organic carbon, Nat. Commun., 12, 4115, https://doi.org/10.1038/s41467-021-24192-8, 2021.
World Bank Group: Cambodia country climate and development report, The World Bank, Washinton DC, The US, https://doi.org/10.1596/978-1-4648-0483-0_ch2_EAP, 2023.
Wuaden, C. R., Nicoloso, R. S., Barros, E. C., and Grave, R. A.: Early adoption of no-till mitigates soil organic carbon and nitrogen losses due to land use change, Soil Till. Res., 204, 104728, https://doi.org/10.1016/j.still.2020.104728, 2020.
Xiao, L., Zhou, S., Zhao, R., Greenwood, P., and Kuhn, N. J.: Evaluating soil organic carbon stock changes induced by no-tillage based on fixed depth and equivalent soil mass approaches, Agr. Ecosyst. Environ., 300, 106982, https://doi.org/10.1016/j.agee.2020.106982, 2020.
Zhang, W.-P., Fornara, D., Yang, H., Yu, R.-P., Callaway, R. M., and Li, L.: Plant litter strengthens positive biodiversity-ecosystem functioning relationships over time, Trend. Ecol. Evol., 38, 473–484, https://doi.org/10.1016/j.tree.2022.12.008, 2023.
Zhang, X., Gregory, A. S., Whalley, W. R., Coleman, K., Neal, A. L., Bacq-Labreuil, A., Mooney, S. J., Crawford, J. W., Soga, K., and Illangasekare, T. H.: Relationship between soil carbon sequestration and the ability of soil aggregates to transport dissolved oxygen, Geoderma, 403, 115370, https://doi.org/10.1016/j.geoderma.2021.115370, 2021.