the Creative Commons Attribution 4.0 License.
the Creative Commons Attribution 4.0 License.
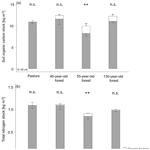
Soil organic carbon stocks did not change after 130 years of afforestation on a former Swiss Alpine pasture
Tatjana C. Speckert
Jeannine Suremann
Konstantin Gavazov
Maria J. Santos
Frank Hagedorn
Guido L. B. Wiesenberg
Soil organic matter (SOM) plays an important role in the global carbon cycle, especially in alpine ecosystems. However, ongoing forest expansion in high-elevation systems potentially alters SOM storage through changes in organic matter (OM) inputs and microclimate. In this study, we investigated the effects of an Picea abies L. afforestation chrono-sequence (0 to 130 years) of a former subalpine pasture in Switzerland on soil organic carbon (SOC) stocks and SOM dynamics. We found that SOC stocks remained constant throughout the chrono-sequence, with comparable SOC stocks in the mineral soils after afforestation and previous pasture (SOC forest40 = 11.6 ± 1.1 kg m−2, SOC forest130 = 11.0 ± 0.3 kg m−2 and SOC pasture = 11.5 ± 0.5 kg m−2). However, including the additional carbon of the organic horizons in the forest, reaching up to 1.7 kg m−2 in the 55-year old forest, resulted in an increase in the overall SOC stocks following afforestation. We found that the soil C:N ratio in the mineral soil increased in the topsoil (0–5 cm) with increasing forest stand age, from 11.9 ± 1.3 in the pasture to 14.3 ± 1.8 in the 130-year old forest. In turn, we observed a decrease in the soil C:N ratio with increasing depth in all forest stand ages. This suggests that litter-derived organic matter (C:N from 35.1 ± 1.9 to 42.4 ± 10.8) is likely to be incorporated and translocated from the organic horizon to the mineral topsoil (0–10 cm) of the profiles. Due to the high root C:N ratio (pasture 63.5 ± 2.8 and forests between 54.7 ± 3.9 and 61.2 ± 2.9), particulate root-derived organic matter seems to have a rather small effect on forest soil C:N ratios, as well as on SOC accumulation in the mineral soil. These results suggest that, although afforestation does not change the SOC stock in the mineral soil, there is an apparent alteration in the SOM dynamics through changes in the litter composition caused by the vegetation shift. We conclude that, at our study site, spruce afforestation on a former subalpine pasture does not change the total SOC stock and that, consequently, there is no additional SOC sequestration on a decadal to centennial scale.
- Article
(1353 KB) - Full-text XML
-
Supplement
(470 KB) - BibTeX
- EndNote
Soil organic matter (SOM) plays an important role in the global carbon cycle, and it is essential for soil fertility and nutrient availability (Prietzel et al., 2016). Furthermore, it increases soil stability and consequently reduces the risk of soil erosion (Garcia-Pausas et al., 2017). Especially in mountain ecosystems, SOM is of particular importance as it forms thick organic layers (Pizzeghello et al., 2017) and contributes significantly to slope stability (Djukic et al., 2010). In alpine environments, cold temperatures result in slower decomposition and lead to more labile particulate SOM compared to temperate soils (Hagedorn et al., 2019; Garcia-Pausas et al., 2017). Specifically, alpine grasslands contain proportionally more easily decomposable plant residues compared with temperate soils (Zimmermann et al., 2007). This makes alpine SOM extremely sensitive to climate warming (Hagedorn et al., 2010). With global warming, accelerated decomposition of SOM will lead to SOM losses, which increases CO2 emissions from soil that enhance the feedback on climate change (Prietzel et al., 2016; Soong et al., 2021).
Afforestation of former pastures is one promising measure of mitigating rising atmospheric CO2 levels and consequently also global warming as it typically contributes to carbon sequestration in biomass (Bastin et al., 2019). In the European Alps, the conversion of pastures to forests is the dominant land use change due to land abandonment (Zimmermann et al., 2010), resulting in an expansion of the forest area by one-third during the last 150 years (Brändli et al., 2020). Afforestation of pastures directly affects SOM dynamics through the alteration of OM inputs as well as through the quality of root and aboveground biomass litter and changes in soil properties (De Deyn et al., 2008; Hiltbrunner et al., 2013). In addition, mycorrhizal nutrient mining associated with ectomycorrhizal tree species can result in a net carbon loss from the mineral soil (Clemmensen et al., 2015; Friggens et al., 2020). In pastures, the OM input usually occurs through root turnover and rhizodeposition (Solly et al., 2013). In forests, however, OM accumulates in tree biomass, as well as in the organic horizons (Peichl et al., 2012). While the increase in aboveground OM following afforestation is widely demonstrated (Thuille and Schulze, 2006; Risch et al., 2008; Guidi et al., 2014; Smal et al., 2019), the effects on belowground OM are more controversial (Hong et al., 2020). Numerous studies have reported contrasting results concerning carbon sequestration following afforestation, including a decrease in SOC stocks (Guo et al., 2007), an initial decrease followed by increase in SOC stocks after decades (Thuille and Schulze, 2006), an increase in SOC stocks (Grünzweig et al., 2007; Risch et al., 2008; Poeplau and Don, 2013) and no effects on the SOC stocks (Davis et al., 2007). These diverging trends are likely context dependent – e.g. they depend on environmental and soil properties, tree species, as well as forest age (De Gryze et al., 2004; Guo and Gifford, 2002; Paul et al., 2002). To date, most studies have focused on the upper-soil horizons (e.g. 0–5 cm, Pérez-Cruzado et al., 2014), leaving gaps in our understanding of what happens at greater soil depths. For example, a decrease in SOC stock was observed following afforestation of previously SOC-rich soils, with the largest decrease being between 10–20 cm depth in an afforested area in northern China (Hong et al., 2020). Also, forest age affects SOM dynamics by directly altering the litter input and its decomposition due to less decomposable compounds with an increased C:N ratio (Gunina et al.,2017). Therefore, it could be expected that longer afforestation periods (> 100 years) might have different effects on SOM dynamics than shorter ones. This effect of forest ages on SOC stock changes remains an underexplored aspect as, more frequently, relatively young afforestation sequences that typically range between 30 and 50 years are studied (Guo et al., 2007; Grünzweig et al., 2007; Strand et al., 2021), and only a handful of studies have investigated afforestation sequences older than 80 years (Thuille and Schulze, 2006; Hiltbrunner et al., 2013).
Tree species composition of forests can also have effects on SOM dynamics as, for example, the SOC stock and SOC accumulation in the organic horizons is generally higher in coniferous forests compared to mixed-deciduous forests (Gosheva et al., 2017). Organic horizons are typically rich in OM that has not been stabilised by interactions with minerals, and they are thus more responsive to environmental changes compared to mineral soils (Yanai et al., 2003). Further, afforestation using coniferous trees on a former pasture resulted in a considerable change in the rooting system, with lower fine-root biomass and higher root C:N ratio and lignin content (Hiltbrunner et al., 2013) compared to pasture. This can result in a lower belowground carbon input into mineral soil. Pastures, on the other hand, are characterised by a higher fine-root biomass (< 2 mm) with higher turnover rates and may thus provide a greater carbon input into SOC than forests (Solly et al., 2013).
The objectives of this study were to investigate SOC stocks and the long-term carbon sequestration potential of an afforestation chrono-sequence of Norway spruce (Picea abies L.) on a former pasture in the Swiss Alps. The specific aims were (i) to examine whether SOC and nitrogen stocks changed in relation to different forest stand ages (0–130 years), (ii) to identify the potential sources of SOM, and (iii) to identify whether C and N dynamics are affected by litter input and the quality of forest stand ages (0–130 years). We hypothesised that, with increasing forest stand age after pasture conversion, there is (i) an increase in the SOC stock due to the changes in litter input and quality towards higher C:N ratios and less easily decomposable OM (e.g. fewer herbaceous leaves and more needles and woody tissues). Further, we expect (ii) an increase in SOC accumulation in the organic horizons with increasing forest age but declining SOC stocks in the mineral soil due to a shift from more root-derived carbon input in the pasture towards more aboveground litter-derived carbon input in the forest sites.
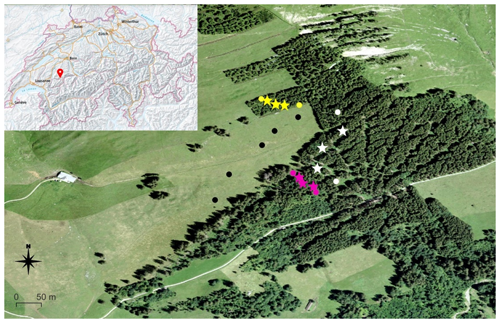
Figure 1Study area and sampling locations, colour-coded: black for pasture, yellow for the 40-year old forest, white for the 55-year old forest and purple for the 130-year old forest. Forest plots marked with a star represent the plots where the mineral soil and organic horizon samples were collected. The map was produced with QGIS 3.22.5; small map of Switzerland: http://map.geo.admin.ch, last access: 6 August 2023.
2.1 Study site
Our study was conducted on a south-exposed slope located above the village of Jaun in the Canton of Fribourg, Switzerland (46∘37′17 N, 7∘15′54 E) at an altitude between 1450 and 1600 m above sea level (Fig. 1). The soil was classified according to the World Reference Base (WRB, 2015) as a Leptic Eutric Cambisol Clayic for both pasture (clay: 60 %, silt: 30 %, sand: 8 %) and forest areas (clay: 50 %, silt: 35 %, sand: 12 %). The mean air temperature is 11.4 ∘C in summer and 0.6 ∘C in winter, with a mean annual precipitation of 1250 mm (Hiltbrunner et al., 2013). The pasture has been used for cattle grazing in the past (Hiltbrunner et al., 2013). After severe avalanches in 1954 and 1968 (Municipality of Jaun, 2021), the pasture was gradually afforested with Norway spruce (Picea abies L.). This human-induced afforestation process lasted several decades and resulted in stand ages between 40 and 55 years. An old forest stand (> 130 years) has been covered by trees for a much longer period, as assessed by tree rings and some strongly decomposed stumps that also revealed tree ring ages of more than 120 years, but strong decomposition hampered exact counting. However, the exact stand age for this old forest could not be determined, even using historical maps, which is the reason why we call this a 130-year old forest. All tree ages were estimated based on tree diameter and tree rings and were verified by aerial photographs and historical maps. The plant community on pasture soils mainly consisted of herbaceous species, with ribgrass (Plantago lanceolata L.) and reed fescue (Festuca arundinacea Schreb.) as the dominant species. Norway spruce (Picea abies L.) was the dominant species in all forest stand ages.
2.2 Sampling design
In our project, we focus on four different forest ages of the afforestation sequence: pasture (0-year old forest) as the control and forest stand ages of 40, 55 and 130 years. These were chosen because of a moderate elevation gradient and comparable soil properties among and within the different forest stand ages (Hiltbrunner et al., 2013), which allows us to investigate changes in SOC composition over several decades. Further, our choice was supported by field inspections, including terrain and soil properties. The size of the individual forest stand ages ranges from ca. 0.55 ha (40 years old) to ca. 0.7 ha (130 years old) to 1.3 ha (55 years old). For every forest stand age, five individual plots with a size of 10 m × 10 m were distributed along an elevational gradient over the entire area of the respective forest and pasture (Fig. 1).
2.3 Sampling and sample preparation
The sampling campaign was conducted in July 2020. Forest canopy cover and the number of trees were determined in the field for all five plots (Fig. 1) for all forest stand ages in a squared area of 10 m × 10 m at each plot, with the soil pit located in its centre, if a soil pit was prepared. Canopy cover was determined by the photographs taken during field work, which enabled the assessment of the canopy cover by pixel. The number of dead and living spruce trees and the number of deciduous trees in the same area were recorded in relation to forest age. The number of trees was assessed from living and recently dead trees (< 3–5 years) as they might have contributed to the litterfall until our sampling campaign in 2020. Trees which were cut off before were not counted. Material of organic soil horizons (O horizons) was collected in July 2020 from three plots (identical to those plots for the soil pits; Fig. 1) in the 40-, 55- and 130-year old forest stands (N = 27; n = 3). The samples of the O horizons were separated into Oi (slightly decomposed organic material), Oe (moderately decomposed organic material) and Oa (highly decomposed organic material; Jahn et al., 2006), and their thickness was measured during field work. O horizons were lacking in the pasture area and were therefore not collected and measured. Five soil pits in the pasture area (0 years old) and three soil pits for each forest stand age (40, 55 and 130 years old) were prepared with dimensions of at least 100 cm width × 50 cm depth. The slope ranged between 25 and 30∘ at all sites. Within the soil pits (five in the pasture area and three in each forest stand age), slope-parallel levels were prepared, and roots were counted on these levels using a grid of 50 cm × 50 cm, according to Gocke et al. (2016). In addition to the counting on slope-parallel levels, roots were counted on three profile walls using the same grid as above to identify the possible sources of SOM, as well as to get an overview of the three-dimensional distribution and variation of root abundance in the soil. The mineral soil samples (N = 118) were taken with two volumetric steel cylinders (100 cm3) on these slope-parallel levels; these were incrementally increased by 5 cm to a maximum depth of 45 cm (pasture n = 5; forest areas n = 3). The bulk density of the mineral soil was determined at every 5 cm interval using the volume of the steel cylinders (100 m3) and the mass of the dried soil after stone removal (Table S1 in the Supplement). All soil samples were stored in open plastic bags until their arrival in the laboratory, where they were stored at −20 ∘C. O-horizon material was freeze-dried and weighed until constant weight. The bulk density of the organic horizons was not obtained as samples of the organic horizons were collected in an area of 0.25 m × 0.25 m and upscaled to 1 m2. Afterwards, the Oi-horizon material was separated into residues of spruce cones, twigs, needles, leaves, moss, lichens, mycorrhiza and other organisms. Mineral soil samples were oven-dried (40 ∘C) until constant weight and passed through a 2 mm sieve to remove any stones, roots and plant residues (Ofiti et al., 2021). Afterwards, roots (N = 180) were manually removed with tweezers and separated into fine (< 2 mm, pasture N = 40, forest40 N = 21, forest55 N = 10, forest130 = 21) and coarse (2–5 mm, pasture N = 12, forest40 N = 25, forest55 N = 25, forest130 N = 26) roots (pasture n = 5, forest n = 3). For further analysis, however, the root samples were combined (0–5 mm), on the one hand, due to less fine-root material, particularly in deeper soil horizons in forest stands, and, on the other hand, to obtain representative and overall comparable results between pasture and forested areas. Soil particles that were attached to the roots were removed by washing the roots with deionised water (Solly et al., 2013) in a sonication bath (Richter-Heitmann et al., 2016). Roots were afterwards dried at 40 ∘C until constant weight (Solly et al., 2013).
2.4 Laboratory analysis
A subsample of all dried samples was ground in a ball mill (MM400, Retsch, Haan, Germany). The milled and homogenised mineral soil samples were acidified with HCl to remove carbonates, washed with deionised water and afterwards dried in the oven at 40 ∘C (Volk et al., 2018). Results of the elemental analysis were corrected for mass loss. Dried root samples were combined (0–5 mm) due to low amounts of the fine-root samples. The material of the roots, organic horizons and mineral soil samples before and after carbonate removal were analysed in duplicate for carbon (C) and nitrogen (N) concentrations, as well as for stable carbon isotope (δ13C) composition, using a Thermo Fisher Scientific Flash HT Elemental Analyser coupled to a Delta V Plus isotope ratio mass spectrometer via ConFlo IV (Thermo Fisher Scientific, Bremen, Germany). The calibration was performed with caffeine (Merck, Germany) and a soil reference sample originating from a Haplic Chernozem soil (clay: 19 %, fine sand: 53 %; Harsum, northern Germany; University of Zurich, 2023). The results of the δ13C values are presented in per mil [‰] relative to the Vienna Pee Dee Belemnite (V-PDB) standard. For the mineral soil samples, total nitrogen (TN concentrations and stocks) was derived from the measurements without carbonate removal, while soil organic carbon (SOC concentrations and stocks) was obtained from the samples after carbonate removal.
2.5 Data analysis
Total SOC and TN stocks per unit area [kg m−2] for the organic horizons were calculated per individual plot based on their organic carbon concentration C [%] multiplied by the dry weight DW [g] of the respective sample upscaled to 1 m2 (multiplied by 16), as in Eq. (1):
Total SOC and TN stocks per unit area [kg m−2] of the mineral soil were calculated per individual plot by first calculating the SOC and TN stocks per individual depth interval [0.05 m] using the soil thickness D of the horizon [m], its bulk density ρ [g cm−3], its SOC concentration C [%] and 10 as a unit conversion factor, as in Eq. (2):
where X represents the SOC and TN stocks [kg m−2] of the individual depth interval. The total SOC and TN stocks of the entire soil to a given depth (0–45 cm) were calculated as follows:
whereby h is the number of the 5 cm depth interval from 0 to 45 cm (h = 9, except for one plot in the pasture where h = 7). We then calculated the average ± standard error (SE) for each stand age for SOC and TN stocks, as well as the δ13C values of mineral soil, organic horizons and roots.
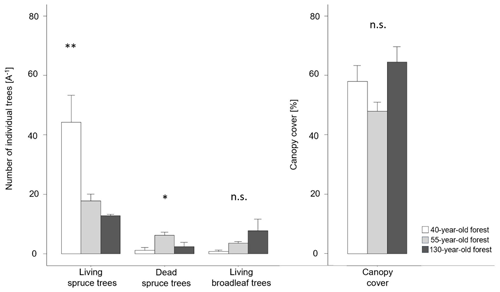
Figure 2Vegetation composition and canopy cover (average ± SE) obtained in an area of 5 m × 5 m for the 40-, 55- and 130-year old forests. ∗ < 0.05; < 0.01, n.s. = not significant.
To test whether SOC, TN and δ13C values differed between land use practices (pasture or forest) or among individual stand ages, we used a one-way analysis of variance (ANOVA, p < 0.05) followed by a post hoc Tukey HSD test (p adj < 0.95). The difference in the vegetation composition in context of forest age was also tested using ANOVA followed by a post hoc Tukey HSD test. The effect of afforestation on the SOM dynamics was tested by means of mixed-effect models with forest age as predictor and soil depth as a fixed effect for each depth interval and the distinct plots as the random effect in the stand age classes in order to reflect the grouped sampling within soil pits. We tested model fit with the REML (restricted maximum likelihood) method using the nlme package, setting the significance level to 0.05. Data analysis was performed with R software 4.0.4 (R Core Team, 2020).
3.1 Vegetation composition
The number of living spruce trees was significantly higher in the 40-year old forest (forest40 = 44 ± 10 A−1, forest55 = 18 ± 2 A−1, forest130 = 13 ± 1 A−1) than in other forest stand ages (F(2,12) = 9.55, p = 0.003; Fig. 2). Further, we also found that the 55-year old forest had a significantly higher number of dead spruce trees (forest55 = 6 ± 1 A−1, forest40 = 1 ± 1 A−1, forest130 = 2 ± 1 A−1) than the other stands (F(2,12) = 4.94, p = 0.027). The 130-year old forest showed a higher number of broadleaf tree species (forest130 = 8 ± 4 A−1, forest55 = 4 ± 1 A−1, forest40 = 1 ± 1 A−1) than the other stand ages (F(2,12) = 2.44, p = 0.129). The largest (65 ± 5 %) canopy cover was observed in the 130-year old forest, followed by the 55-year old (48 ± 3 %) and 40-year old forests (58 ± 5 %), but these were not significantly different (F(2,12) = 3.24, p = 0.075).
3.2 Macroscopic organic matter remains in upper (Oi) organic horizons
In all forest stand ages, spruce cones and branches were significantly more abundant in the Oi horizon of all forest stand ages (F(5,8) = 9.161, p = 0.004; Table S2 in the Supplement) in comparison to moss or grass residues. The highest proportion of cone residues was found in the 55-year old forest (65 mass % of the Oi horizon), followed by the 130-year old (58 mass %) and 40-year old forests (33 mass %). Also, needles made up almost one-fourth (25 %) of the mass in Oi horizons of the 40-year old forest but only around 10 % in the 55-year old (5 %) and 130-year old forests (8 %).
3.3 Root biomass and frequency
We found significant differences in the fine-root biomass (0–2 mm) between the pasture and the different forest ages (F(3,88) = 5.21, p = 0.002). The highest fine-root biomass (0–45 cm) was found in the pasture (184.9 ± 39.5 g m−2), and the lowest fine-root biomass was observed in the 55-year old forest (2.9 ± 1.4 g m−2; Fig. 3a). The highest coarse-root biomass (2–5 mm) was found in the 130-year old forest (683.5 ± 202.1 g m−2) and was lowest in pasture areas (274.2 ± 209.2 g m−2). However, the overall differences between the pasture and the different forest stand ages remained insignificant (F(3, 84) = 1.29, p = 0.282; Fig. 3b). Both, fine- (F(8,72) = 3.43, p = 0.002) and coarse-root biomass (F(8,68) = 6.28, p = 8.0 × 10−4) decreased with increasing soil depth (Table S3 in the Supplement). This is in line with the root frequency that was counted on horizontal levels and profile walls, where we observed the highest fine-root frequency (∼ 15 800 m−2) in the pasture and the highest coarse-root frequency in the 40-year old forest (∼ 2500 m−2; Table S3), with decreasing numbers with depth.
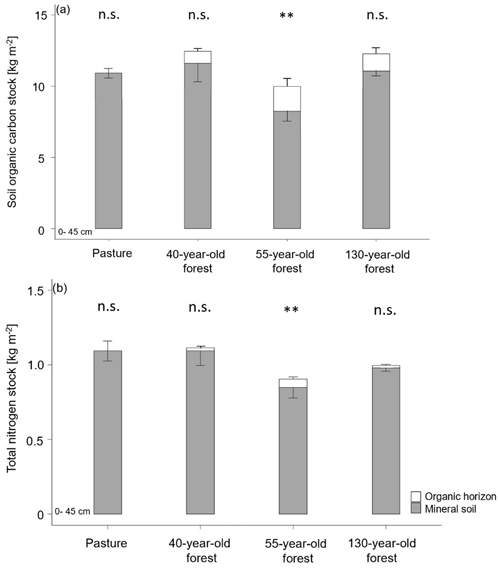
Figure 4(a) Soil organic carbon stocks and (b) total nitrogen stocks in the O horizons and the mineral soil (0–45 cm; average ± SE) by vegetation cover and forest age. < 0.01; n.s. = not significant.
3.4 Soil organic C and N stocks
As organic matter horizons were completely missing in the pasture during the sampling campaign, organic horizons were only quantified in afforested areas. There was no significant difference in the SOC stock of the organic horizons between the different forest stand ages (F(1,25) = 0.065, p = 0.801; Fig. 4a). The highest SOC stock was found in the Oi horizon in the 55-year old forest (0.9 ± 0.1 kg m−2) with a drop in the SOC stock from Oi to Oe in all forest stand ages (Table 1). Also, the combined SOC stock of the organic horizons (Oi, Oe and Oa) was highest in the 55-year old forest (1.7 ± 0.2 kg m−2) followed by the 130-year old (1.3 ± 0.2 kg m−2) and 40-year old forests (0.8 ± 0.1 kg m−2). The total SOC stocks of the mineral soil (0–45 cm) in the 40-year old (11.6 ± 1.1 kg m−2) and 130-year old forests (11.0 ± 0.3 kg m−2) were identical to that in the pasture soil (11.5 ± 0.5 kg m−2). In contrast, the total SOC stock of the mineral soil (0–45 cm) in the 55-year old forest (8.3 ± 0.6 kg m−2) was significantly lower compared to the soils of the pasture, as well as those of the other forest stand ages (F(3,114) = 4.02, p = 0.009). Including the organic horizons, there was an increased C accumulation of +8 % in the 40-year old forest and +7 % in the 130-year old forest as compared to the pasture soils, but this increase was not significant (F(2,14) = 1.64, p = 0.229). Within the soil profiles, the highest SOC stocks occurred in the topsoil (0–5; 5–10 cm) in both pasture and forest soils, and these decreased with increasing soil depth (F(8,98) = 20.45, p = 2 × 10−16; Tables 1 and S4 in the Supplement). The TN stock of the organic horizons did not differ between the different forest ages (F(1,27) = 0.157, p = 0.696). However, the largest TN stock of the combined organic horizons (Oi, Oe, Oa) was observed in the 55-year old forest (0.05 ± 0.01 kg m−2) followed by the 130-year old and 40-year old forests (Fig. 4b). The TN stock of the mineral soil (0–45 cm) of the pasture (1.16 ± 0.08 kg m−2) equals that of the 40-year old forest (1.09 ± 0.08 kg m−2), followed by the 130-year old (0.98 ± 0.02 kg m−2) and 55-year old forests (0.85 ± 0.06 kg m−2; F(3,114) = 5.31, p = 0.002). Similarly to the SOC stock, the TN stock also significantly (F(8,98) = 11.99, p = 1.34 × 10−15) decreased with depth (Tables 1 and S4).
Table 2C:N ratio and δ13C values of root (0–5 mm) samples (n = 5 for pasture; n = 3 for forest). Values are average ± SE (n.a. = not available, average ± SE of the individual plots; n = 5 for pasture; n = 3 for forest).
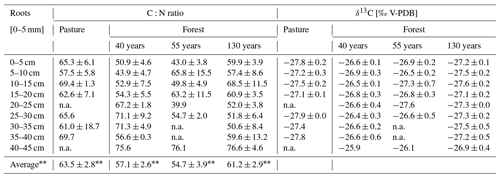
3.5 Soil organic matter composition
The C:N ratio of the O horizons (Oi, Oe, Oa) in the forest was not significantly different between the different forest ages (F(1,25) = 2.134, p = 0.157; Table 3). In all forest stand ages, the highest C:N ratios were observed in the Oi horizons (forest130 = 42.4 ± 10.8, forest55 = 37.5 ± 3.1, forest40 = 35.5 ± 1.9), which consistently decreased from the Oi to Oa horizons. The C:N ratio of roots (0–5 mm diameter) did not significantly differ (F(3,98) = 1.55, p = 0.208) between the pasture and afforested areas (Table 2). The highest root C:N ratio was observed in the pasture (0–5 cm) soil with 65.3 ± 6.1, followed by the 130-year old (59.9 ± 3.9), the 40-year old (50.9 ± 4.6) and the 55-year old forests (43.0 ± 3.8). Within the O horizons, the δ13C values increased from Oi towards Oa in the 55-year old forest (F(2,6) = 12.15, p = 0.008). The δ13C values in the 40-year old (F(2,6) = 2.389, p = 0.173) and 130-year old forests (F(2,6) = 0.212, p = 0.815) did not changed significantly from the Oi horizon towards the Oa horizon. Within the mineral soil, the δ13C values increased (F(3,114) = 12.04, p = 5.97 × 10−8) with increasing forest age. They decreased with increasing soil depth (F(8,98) = 12.05, p = 1.13 × 10−12; Table S5 in the Supplement), with the highest differences being pronounced in the subsoil (30–45 cm; Table S5), ranging between −23.3 ± 2.4 ‰ in the 40-year old forest and −25.5 ± 0.2 ‰ in the pasture site (Table 3). Pasture roots had δ13C values that varied between −27.8 ± 0.2 ‰ and −27.1 ± 0.1 ‰ with depth. These values were significantly different from roots (F(3,98) = 11.80, p = 1.32 × 10−9) sampled in the soils of afforested areas (forest40 = between −26.9 ± 0.3 ‰ and −25.9 ‰; forest55 = between −27.3 ± 0.7 ‰ and −26.1 ‰; forest130 = between −27.6 ± 0.2 and −26.9 ± 0.4 ‰). Considering the soil depth of 45 cm, the δ13C values of the roots became more (∼ +1 ‰) positive with increasing soil depth in the pasture, as well as in afforested areas (Table 2), although not significantly (F(8,82) = 0.78, p = 0.625).
4.1 SOC stock changes depending on forest age
We measured SOC stock of 11.5 ± 0.5 kg m−2 in the subalpine pasture (Fig. 4a), which is in line with the SOC stock observed by Hiltbrunner et al. (2013), with a SOC stock of 13.4 ± 1.2 kg m−2 in the alpine pasture. One possible reason for this minor difference in the SOC stock could be the difference in sampling depth: 0–60 cm in Hiltbrunner et al. (2013) and 0–45 cm in this study. Nevertheless, our reported SOC stock in the pasture is comparable with the reported SOC stock of Budge et al. (2011), who observed a SOC stock between 5.5 and 10.2 kg m−2 down to 30 cm depth in alpine grasslands in the Swiss Alps. In another study, Zeeman et al. (2010) reported lower SOC stocks between 4 and 6 kg m−2 (0–20 cm) in subalpine grasslands in Switzerland, which could be due to the fact that they focused on managed agricultural grasslands compared to a natural grassland that was investigated in the current study. The SOC stock of forest areas in this study, ranging between 8.3 ± 0.6 kg m−2 (55-year old forest) and 11.6 ± 1.1 kg m−2 (40-year old forest), is in line with the reported SOC stocks for Swiss forest soils (Nussbaum et al. 2014), ranging between 1 and 36 kg m−2 (average 13 kg m−2). Our findings that observe an increase in the SOC stock in the mineral soil after 40 years of afforestation conforms with the findings of other studies which reported an increase in the SOC stock after 40 to 50 years of afforestation (Thuille and Schulze, 2006; Hiltbrunner et al. 2013). In our study, the 40-year old forest is not only the youngest in the investigated chrono-sequence, but it experienced some transversal clear cuts a few years ago as part of forest management. Consequently, the higher SOC stock in the 40-year old forest as compared to that in older stands in our study can further be explained by the presence of grass roots. They typically dominate the carbon input in grasslands (Thuille and Schulze 2006) and generate additional SOC input (Laganière et al., 2010), which possibly occurred in the 40-year old forest but not in the 55-year old and 130-year old forests, where the grasses disappeared. Along the same line, the 40-year old forest showed an identical TN stock to that of the pasture (Fig. 4b), which suggests that only a small amount of nitrogen is sequestered by trees. The decrease in the SOC stock from the 40-year old to 55-year old forests agrees with other chrono-sequences (Post and Kwon, 2000; Thuille and Schulze, 2006; Poeplau et al., 2011). One possible explanation could be the absence of grass cover, as reported by Hiltbrunner et al. (2013), which is also supported by the lower fine- and coarse-root biomass in the 55-year old forest found in this study compared to that in the 40-year old forest. Further, avalanche protection infrastructure was built only 10–15 m uphill from one of our profiles, which might have resulted in potential soil disturbances resulting from avalanche barrier construction or former avalanches in that area. The cause of the observed effects cannot be entirely elucidated to date, but all of these factors could have caused a loss of carbon compared with the other forest stands. In particular, the latter explanation might align with the SOC stock in relation to soil depth, where we observed a lower SOC stock at 15 to 25 cm soil depth compared to the 40- and 130-year old forests (Table 1). In addition, there is no significant difference in the SOC stocks reported by Hiltbrunner et al. (2013) 10 years ago in the same study site and the reported SOC stock in this study. Therefore, additionally, 10 years of afforestation might not increase SOC stocks in the mineral soil. To better understand why we have no increased C sequestration, even after decades of afforestation, the use of molecular proxies would be one possible option to identify potential sources of OM, as well as to identify the alteration in its composition (Jansen and Wiesenberg, 2017).
In addition to the carbon stored in the mineral soil, all forest soils showed well-differentiated organic horizons, where additional carbon has been accumulated. Consequently, SOC sequestration of an additional 0.8 ± 0.1 and 1.7 ± 0.2 kg m−2 occurred in the organic horizons of afforested areas compared to the pasture (Fig. 4a) at Jaun. This agrees with other studies reporting the potential of an additional carbon sequestration in organic horizons of 2.3 kg m−2 (Hiltbrunner et al., 2013) and of 2.5 kg m−2 (Thuille and Schulze, 2006) following afforestation. In contrast to Hiltbrunner et al. (2013), we did not find a gradual increase in the SOC stock of the organic horizons with increasing forest age at the same site. This could be due to the sampled locations and erosion of the unconsolidated organic horizons that might have occurred at selected areas due to snowmelt or heavy rainfall events. The higher SOC stock in the organic horizons of the 55-year old forest compared to the 40- and 130-year old forests reported in the current study may be explained by the litter composition, which mainly consisted of dead wood residues (Table S2). This material is less decomposable than leaf or needle litter, which resulted in a lower decomposition (Cotrufo et al., 2013) and higher carbon accumulation and therefore thicker organic horizons (3.2 cm ± 0.4 cm) compared to the 40- and 130-year old forest stands. Overall, if the organic horizons were combined with the mineral soil, then there was an additional but small SOC sequestration within 40 and 130 years of afforestation. This confirms our hypothesis of an increase in the SOC sequestration caused by an additional carbon accumulation on the organic horizons. However, this pathway of carbon accumulation has to be regarded with caution as organic matter found in organic horizons in steep alpine areas is more biochemically and much less physically protected than mineral-bound SOC (Thuille and Schulze, 2006). This argues for an increased sensitivity of SOC stocks in forested alpine areas and demands further protection measures, e.g. during forest renewal, where specifically organic horizons can be exposed to erosion and degradation (Bettoni et al., 2023).
4.2 SOC alteration vs. sequestration with forest age
While the mineral SOC stock tended to remain unchanged within the investigated afforestation sequence, and while the SOC stock increased within the first 55 years, it remained an open question as to what extent mineral SOM contains preserved carbon from the pasture or how this is replaced by forest-derived carbon. The incorporation of forest-derived carbon can occur either via litterfall and the continuous incorporation and degradation of litter within the organic horizons on the one hand and via root organic matter on the other hand. With increasing forest age, the C:N ratio of the Oi horizons (Table 3) increased, although not significantly, which argues for a lower decomposability of the litter caused by an increase in woody litter with increasing stand age (Schulp et al., 2008). This shift in the litter composition towards less decomposable material was previously described (Pérez-Cruzado et al., 2014; Strand et al., 2021). Furthermore, a higher C:N ratio in the organic horizons as a function of tree age was also reported by Thuille and Schulze (2006), with an increase in woody litter such as twigs, branches and spruce cones. In addition, element concentration, such as nitrogen, decreased with increasing needle age (Linder, 1995), which could be another reason for the observed shift towards less decomposable material. With ongoing decomposition from the Oi to Oa horizons, the C:N ratio decreased, which was confirmed in all forest ages at Jaun. On the one hand, the C:N ratio in the mineral soil increased as a function of forest age, resulting in the highest C:N ratio of 14.3 ± 1.8 in the 130-year old forest (Table 3), and on the other hand, the C:N ratio decreased with soil depth, specifically between 10 and 20 cm (Table S5). This demonstrates that litter-derived organic matter was likely incorporated and translocated from litter to mineral soil in the top 20 cm of the profiles, while, partially, very low C:N ratios in deeper soils argue for an increased degradation of organic matter in forest soils (Lorenz et al., 2020). As roots had very high C:N ratios, particulate root-derived organic matter might not directly contribute to SOM. Organic matter input from roots cannot be completely excluded as the release of root exudates into the soil promote microbial decomposition of such compounds and thereby results in SOM with a low C:N ratio (Cotrufo et al., 2013; Jílková et al., 2022). Consequently, in this study, the aboveground litter quantity and quality seem to have the biggest effect on SOM dynamics in the topsoil. This is in line with previous findings, where a large proportion of the carbon input in afforested areas is mainly through litterfall (Hiltbrunner et al., 2013, Bárcena et al., 2014), whereas this can be restricted to the top 20 cm in our study. Based on the δ13C values, there is a shift from −25.5 ± 0.2 ‰ to −26.9 ± 0.3 ‰ in the original pasture to −24.4 ± 0.3 ‰ to −26.0 ± 0.2 ‰ in the 130-year old forest (Table 1). This indicates a transition towards tree-derived carbon as a main source for the SOM in afforested areas. The enrichment in 13C with increasing forest age could be indicative for an enrichment of the heavier 13C isotope during decomposition. As forest roots are more depleted in 13C (−1 to 3 ‰) compared to the mineral soil, it seems that roots do not have an effect on the δ13C values of the mineral soil. In contrast, fungal species, especially the saprotrophic fungi, are known to have higher δ13C values (−22.8 ± 0.3 ‰; Hobbie et al., 2001), which could thus be a potential explanation for the observed 13C enrichment in the forest soil, especially in the 130-year old forest (Table 3). Along with the study of Hiltbrunner et al. (2013), we also observed a high variability between the δ13C values of the roots and the mineral soil in the oldest (130 year old) forest stand age, which is another argument for a slower root turnover with increasing forest age. Additionally, the mineral soil in the pasture areas is less enriched in 13C, which is also the case for the pasture root δ13C values, compared to roots from the forest. Also, this suggests that fine roots are a major C source in the pasture areas (Solly et al., 2013; Hiltbrunner et al., 2013).
Afforestation of abandoned alpine pastures and a rise in the treeline have led to a forest expansion of European alpine areas during the past decades. In our study, we investigated the effects of a 130-year old afforestation chrono-sequence on a former subalpine pasture on the total SOC stock, as well as on the possible alteration of the SOM dynamics. Conclusively, the SOC stock of the mineral soil did not change 40 and 130 years after afforestation compared with the original pasture soil. If the organic horizons are included, there was an increase in carbon stocks by 1–2 kg m−2. This lack of additional sequestration in mineral soil C and the restriction of C sequestration specifically in organic horizons poses a limitation to the suitability of afforestation measures for climate change mitigation. Specifically, organic horizons are highly vulnerable to erosion in steep alpine areas as well as to mineralisation losses upon disturbances. Therefore, the restriction to C sequestration in organic horizons is less sustainable than in mineral soil. Although the effects on SOC stocks following afforestation are only moderate in the investigated area, the vegetation shift resulted in an obvious alteration in the SOC dynamics through changes in the litter composition and changes in root-derived organic matter. The alteration towards a higher C:N ratio with increasing forest age, especially in the Oi horizon, reflects this alteration in less easily decomposable compounds. Specifically, the δ13C values and C:N ratios strongly suggest an alteration of soil organic matter composition with increasing forest age likely resulting from changes in organic matter sources and its degradation without allowing final conclusions. To better understand the processes following afforestation and climate change in alpine areas, further investigations are needed to specifically quantify the sources of organic matter and SOM degradation under different land uses in alpine areas.
To access the code, please contact the correspondence author.
The dataset used in this study is available in the EnviDat data repository (https://www.doi.org/10.16904/envidat.464, Speckert et al., 2023).
The supplement related to this article is available online at: https://doi.org/10.5194/soil-9-609-2023-supplement.
TCS: conceptualisation, methodology, writing and editing – original draft, investigation, data curation, formal analysis. JS: investigation, writing – review and editing. KG: conceptualisation, resources, validation, writing – review and editing. MJS: data curation, formal analysis, writing – review and editing. FH: resources, validation, writing – review and editing. GLBW: conceptualisation, methodology, funding, resources, writing – review and editing.
The contact author has declared that none of the authors has any competing interests.
Publisher's note: Copernicus Publications remains neutral with regard to jurisdictional claims made in the text, published maps, institutional affiliations, or any other geographical representation in this paper. While Copernicus Publications makes every effort to include appropriate place names, the final responsibility lies with the authors.
We thank Silvan Wick, Yves Brügger and Carrie L. Thomas for their help during the field work. We further thank Jeannine Suremann, Aline Hobie, Barbara Siegfried, Dmitry Tichomirov and Esmail Taghizadeh for their support during the lab work. We acknowledge funding by the Swiss National Science Foundation (SNSF) under contract no. 188684 of the IQ-SASS project (Improved Quantitative Source Assessment of organic matter in Soils and Sediments using molecular markers and inverse modelling) to GLBW and under project no. PZ00P2_174047 to Konstantin Gavazov. We further thank Boris Jansen and two anonymous reviewers for their helpful comments that improved the manuscript.
This research has been supported by the Schweizerischer Nationalfonds zur Förderung der Wissenschaftlichen Forschung (grant nos. 188684 and 174047).
This paper was edited by Boris Jansen and reviewed by two anonymous referees.
Bárcena, T. G., Kiær, L. P., Vesterdal, L., Stefánsdóttir, H. M., Gundersen, P., and Sigurdsson, B. D.: Soil carbon stock change following afforestation in Northern Europe: A meta-analysis, Glob. Change Biol., 20, 2393–2405, https://doi.org/10.1111/gcb.12576, 2014.
Bastin, J. F., Finegold, Y., Garcia, C., Mollicone, D., Rezende, M., Routh, D., Zohner, C. M., and Crowther, T. W.: The global tree restoration potential, Science, 365, 76–79, https://doi.org/10.1126/science.aax0848, 2019.
Brändli, U. B., Abegg, M., and Allgaier Leuch, B.: Schweizerisches Landesforstinventar, Ergebnisse der vierten Erhebung 2009–2017, Birmensdorf: Eidgenöss Forschanstalt WSL, 341 pp., 2020.
Bettoni, M., Maerker, M., Sacchi, R., Bosino, A., Conedera, M., Simoncelli, L., and Vogel, S.: What makes soil landscape robust? Landscape sensitivity towards land use changes in a Swiss southern Alpine valley, Sci. Total Environ., 858, 159779, https://doi.org/10.1016/j.scitotenv.2022.159779, 2023.
Budge, K., Leifeld, J., Hiltbrunner, E., and Fuhrer, J.: Alpine grassland soils contain large proportion of labile carbon but indicate long turnover times, Biogeosciences, 8, 1911–1923, https://doi.org/10.5194/bg-8-1911-2011, 2011.
Clemmensen, K. E., Finlay, R. D., Dahlberg, A., Stenlid, J., Wardle, D. A., and Lindahl, B. D.: Carbon sequestration is related to mycorrhizal fungal community shifts during long-term succession in boreal forests, New Phytol., 205, 1525–1536, https://doi.org/10.1111/nph.13208, 2015.
Cotrufo, M. F., Wallenstein, M. D., Boot, C. M., Denef, K., and Paul, E.: The Microbial Efficiency-Matrix Stabilization (MEMS) framework integrates plant litter decomposition with soil organic matter stabilization: Do labile plant inputs form stable soil organic matter?, Glob. Change Biol., 19, 988–995, https://doi.org/10.1111/gcb.12113, 2013.
Davis, M., Nordmeyer, A., Henley, D., and Watt, M.: Ecosystem carbon accretion 10 years after afforestation of depleted subhumid grassland planted with three densities of Pinus nigra, Glob. Change Biol., 13, 1414–1422, https://doi.org/10.1111/j.1365-2486.2007.01372.x, 2007.
De Deyn, G. B., Cornelissen, J. H., and Bardgett, R. D.: Plant functional traits and soil carbon sequestration in contrasting biomes, Ecol. Lett., 11, 516–531, https://doi.org/10.1111/j.1461-0248.2008.01164.x, 2008.
De Gryze, S., Six, J., Paustian, K., Morris, S. J., Paul, E. A., and Merckx, R.: Soil organic carbon pool changes following land-use conversions, Glob. Change Biol., 10, 1120–1132, https://doi.org/10.1111/j.1529-8817.2003.00786.x, 2004.
Djukic, I., Zehetner, F., Tatzber, M., and Gerzabek, M. H.: Soil organic matter stocks and characteristics along an Alpine elevation gradient, J. Plant Nutr. Soil Sc., 173, 30–38, https://doi.org/10.1002/jpln.200900027, 2010.
Friggens, N. L., Hester, A. J., Mitchell, R. J., Parker, T. C., Subke, J. A., and Wookey, P. A.: Tree planting in organic soils does not result in net carbon sequestration on decadal timescales, Glob. Change Biol., 26, 5178–5188, https://doi.org/10.1111/gcb.15229, 2020.
Garcia-Pausas, J., Romanyà, J., Montané, F., Rios, A. I., Taull, M., Rovira, P., and Casals, P.: Are soil carbon stocks in Mountain grasslands compromised by land-use changes?, in: High Mountain Conservation in a Changing World. Advances in Global Change Research, vol 62, edited by: Catalan, J., Ninot, J., and Aniz, M., Springer, Cham, https://doi.org/10.1007/978-3-319-55982-7_9, 2017.
Grünzweig, J. M., Gelfand, I., Fried, Y., and Yakir, D.: Biogeochemical factors contributing to enhanced carbon storage following afforestation of a semi-arid shrubland, Biogeosciences, 4, 891–904, https://doi.org/10.5194/bg-4-891-2007, 2007.
Gocke, M. I., Kessler, F., van Mourik, J. M., Jansen, B., and Wiesenberg, G. L. B.: Paleosols can promote root growth of recent vegetation – a case study from the sandy soil–sediment sequence Rakt, the Netherlands, SOIL, 2, 537–549, https://doi.org/10.5194/soil-2-537-2016, 2016.
Gosheva, S., Walthert, L., Niklaus, P. A., Zimmermann, S., Gimmi, U., and Hagedorn, F.: Reconstruction of historic forest cover changes indicates minor effects on carbon stocks in Swiss forest soils, Ecosystems, 20, 1512–1528, https://doi.org/10.1007/s10021-017-0129-9, 2017.
Guo, L. B. and Gifford, R. M.: Soil carbon stocks and land use change: A meta-analysis, Glob. Change Biol., 8, 345–360, https://doi.org/10.1046/j.1354-1013.2002.00486.x, 2002.
Guo, L. B., Wang, M., and Gifford, R. M.: The change of soil carbon stocks and fine root dynamics after land use change from a native pasture to a pine plantation, Plant Soil, 299, 251–262, https://doi.org/10.1007/s11104-007-9381-7, 2007.
Guidi, C., Vesterdal, L., Gianelle, D., and Rodeghiero, M.: Changes in soil organic carbon and nitrogen following forest expansion on grassland in the Southern Alps, Forest Ecol. Manag., 328, 103–116, https://doi.org/10.1016/j.foreco.2014.05.025, 2014.
Gunina, A., Smith, A. R., Godbold, D. L., Jones, D. L., and Kuzyakov, Y.: Response of soil microbial community to afforestation with pure and mixed species, Plant Soil, 412, 357–368, https://doi.org/10.1007/s11104-016-3073-0, 2017.
Hagedorn, F., Martin, M., Rixen, C., Rusch, S., Bebi, P., Zürcher, A., Siegwolf, R. T. W., Wipf, S., Escape, C., Roy, J., and Hättenschwiler, S.: Short-term responses of ecosystem carbon fluxes to experimental soil warming at the Swiss alpine treeline, Biogeochemistry, 97, 7–19, https://doi.org/10.1007/s10533-009-9297-9, 2010.
Hagedorn, F., Gavazov, K., and Alexander, J. M.: Above – and belowground linkages shape responses of mountain vegetation to climate change, Science, 365, 1119–1123, https://doi.org/10.1126/science.aax4737, 2019.
Hiltbrunner, D., Zimmermann, S., and Hagedorn, F.: Afforestation with Norway spruce on a subalpine pasture alters carbon dynamics but only moderately affects soil carbon storage, Biogeochemistry, 115, 251–266, https://doi.org/10.1007/s10533-013-9832-6, 2013.
Hobbie, E. A., Weber, N. S., and Trappe, J. M.: Mycorrhizal vs saprotrophic status of fungi: The isotopic evidence, New Phytol., 150, 601–610, 2001.
Hong, S., Yin, G., Piao, S., Dybzinski, R., Cong, N., Li, X., Wang, K., Peñuelas, J., Zeng, H., and Chen, A.: Divergent responses of soil organic carbon to afforestation, Nature Sustainability, 3, 694–700, https://doi.org/10.1038/s41893-020-0557-y, 2020.
Jahn, R., Blume, H. P., Asio, V., Spaargaren, O., and Schad, P.: Guidelines for Soil Description, FAO, 2006.
Jansen, B. and Wiesenberg, G. L. B.: Opportunities and limitations related to the application of plant-derived lipid molecular proxies in soil science, SOIL, 3, 211–234, https://doi.org/10.5194/soil-3-211-2017, 2017.
Jílková, V., Jandová, K., Cajthaml, T., Kukla, J., and Jansa, J.: Differences in the flow of spruce-derived needle leachates and root exudates through a temperate coniferous forest mineral topsoil, Geoderma, 405, 115441, https://doi.org/10.1016/j.geoderma.2021.115441, 2022.
Laganière, J., Angers, D. A., and Pare, D.: Carbon accumulation in agricultural soils after afforestation: A meta-analysis, Glob. Change Biol., 16, 439–453, https://doi.org/10.1111/j.1365-2486.2009.01930.x, 2010.
Linder, S.: Foliar analysis for detecting and correcting nutrient imbalances in Norway spruce, Ecol. Bull., 44, 178–190, 1995.
Lorenz, M., Derrien, D., Zeller, B., Udelhoven, T., Werner, W., and Thiele-Bruhn, S.: The linkage of 13C and 15N soil depth gradients with C:N and O:C stoichiometry reveals tree species effects on organic matter turnover in soil, Biogeochemistry, 151, 203–220, https://doi.org/10.1007/s10533-020-00721-3, 2020.
Municipality of Jaun: https://www.jaun.ch (last access: 2021), 2021.
Nussbaum, M., Papritz, A., Baltensweiler, A., and Walthert, L.: Estimating soil organic carbon stocks of Swiss forest soils by robust external-drift kriging, Geosci. Model Dev., 7, 1197–1210, https://doi.org/10.5194/gmd-7-1197-2014, 2014.
Ofiti, N. O., Zosso, C. U., Soong, J. L., Solly, E. F., Torn, M. S., Wiesenberg, G. L. B., and Schmidt, M. W. I.: Warming promotes loss of subsoil carbon through accelerated degradation of plant-derived organic matter, Soil Biol. Biochem., 156, 108185, https://doi.org/10.1016/j.soilbio.2021.108185, 2021.
Paul, K. I., Polglase, P. J., Nyakuengama, J. G., and Khanna, P. K.: Change in soil carbon following afforestation, Forest Ecol. Manag., 168, 241–257, https://doi.org/10.1016/S0378-1127(01)00740-X, 2002.
Peichl, M., Leava, N. A., and Kiely, G.: Above-and belowground ecosystem biomass, carbon and nitrogen allocation in recently afforested grassland and adjacent intensively managed grassland, Plant Soil, 350, 281–296, https://doi.org/10.1007/s11104-011-0905-9, 2012.
Pérez-Cruzado, C., Sande, B., Omil, B., Rovira, P., Martin-Pastor, M., Barros, N., Salgado, J., and Merino, A.: Organic matter properties in soils afforested with Pinus radiata, Plant Soil, 374, 381–398, https://doi.org/10.1007/s11104-013-1896-5, 2014.
Pizzeghello, D., Francioso, O., Concheri, G., Muscolo, A., and Nardi, S.: Land use affects the soil C sequestration in alpine environment, NE Italy, Forests, 8, 197, https://doi.org/10.3390/f8060197, 2017.
Poeplau, C. and Don, A.: Sensitivity of soil organic carbon stocks and fractions to different land-use changes across Europe, Geoderma, 192, 189–201, https://doi.org/10.1016/j.geoderma.2012.08.003, 2013.
Poeplau, C., Don, A., Vesterdal, L., Leifeld, J., Van Wesemael, B. A. S., Schumacher, J., and Gensior, A.: Temporal dynamics of soil organic carbon after land-use change in the temperate zone – Carbon response functions as a model approach, Glob. Change Biol., 17, 2415–2427, https://doi.org/10.1111/j.1365-2486.2011.02408.x, 2011.
Post, W. M. and Kwon, K. C.: Soil carbon sequestration and land-use change: Processes and potential, Glob. Change Biol., 6, 317–327, https://doi.org/10.1046/j.1365-2486.2000.00308.x, 2000.
Prietzel, J., Zimmermann, L., Schubert, A., and Christophel, D.: Organic matter losses in German Alps Forest soils since the 1970s most likely caused by warming, Nat. Geosci., 9, 543–548, https://doi.org/10.1038/ngeo2732, 2016.
R Core Team: A language and environment for statistical computing, R Foundation for Statistical Computing, Vienna, Austria, https://www.R-project.org/ (last access: 20 October 2023), 2020.
Richter-Heitmann, T., Eickhorst, T., Knauth, S., Friedrich, M. W., and Schmidt, H.: Evaluation of strategies to separate root-associated microbial communities: A crucial choice in rhizobiome research, Front. Microbiol., 7, 773, https://doi.org/10.3389/fmicb.2016.00773, 2016.
Risch, A. C., Jurgensen, M. F., Page-Dumroese, D. S., Wildi, O., and Schütz, M.: Long-term development of above-and below-ground carbon stocks following land-use change in subalpine ecosystems of the Swiss National Park, Can. J. Forest Res., 38, 1590–1602, https://doi.org/10.1139/X08-014, 2008.
Schulp, C. J., Nabuurs, G. J., Verburg, P. H., and de Waal, R. W.: Effect of tree species on carbon stocks in forest floor and mineral soil and implications for soil carbon inventories, Forest Ecol. Manag., 256, 482–490, https://doi.org/10.1016/j.foreco.2008.05.007, 2008.
Smal, H., Ligęza, S., Pranagal, J., Urban, D., and Pietruczyk-Popławska, D.: Changes in the stocks of soil organic carbon, total nitrogen and phosphorus following afforestation of post-arable soils: A chronosequence study, Forest Ecol. Manag., 451, 117536, https://doi.org/10.1016/j.foreco.2019.117536, 2019.
Solly, E., Schöning, I., Boch, S., Müller, J., Socher, S. A., Trumbore, S. E., and Schrumpf, M.: Mean age of carbon in fine roots from temperate forests and grasslands with different management, Biogeosciences, 10, 4833–4843, https://doi.org/10.5194/bg-10-4833-2013, 2013.
Soong, J. L., Castanha, C., Hicks Pries, C. E., Ofiti, N., Porras, R. C., Riley, W. J., Schmidt, M. W. I., and Torn, M. S.: Five years of whole soil warming led to loss of subsoil carbon stocks and increased CO2 efflux, Science Advances, 7, eabd1343, https://doi.org/10.1126/sciadv.abd1343, 2021.
Speckert, T., Suremann, J., Gavazov, K., Santos, M. J., Hagedorn, F., and Wiesenberg, G. L. B.: Soil organic carbon stock of an afforestation sequence on a subalpine pasture, EnviDat [data set], https://www.doi.org/10.16904/envidat.464 (last access: 30 November 2023), 2023.
Strand, L. T., Fjellstad, W., Jackson-Blake, L., and De Wit, H. A.: Afforestation of a pasture in Norway did not result in higher soil carbon, 50 years after planting, Landscape Urban Plan., 207, 104007, https://doi.org/10.1016/j.landurbplan.2020.104007, 2021.
Thuille, A. and Schulze, E. D.: Carbon dynamics in successional and afforested spruce stands in Thuringia and the Alps, Glob. Change Biol., 12, 325–342, https://doi.org/10.1111/j.1365-2486.2005.01078.x, 2006.
University of Zurich: https://www.geo.uzh.ch/en/units/2b/Services/BC-material/Environmental-matrices.html (last access: 20 November 2021), 2023.
Volk, M., Bassin, S., Lehmann, M. F., Johnson, M. G., and Andersen, C. P.: 13C isotopic signature and C concentration of soil density fractions illustrate reduced C allocation to subalpine grassland soil under high atmospheric N deposition, Soil Biol. Biochem., 125, 178–184, https://doi.org/10.1016/j.soilbio.2018.07.014, 2018.
WRB: FAO, World reference base for soil resources 2014, update 2015: International soil classification system for naming soils and creating legends for soil maps, World Soil Resources, 2015.
Yanai, R., Currie, W., and Goodale, C.: Soil Carbon Dynamics after Forest Harvest: An ecosystem paradigm reconsidered, Ecosystems 6, 197–212, https://doi.org/10.1007/s10021-002-0206-5, 2003.
Zeeman, M. J., Hiller, R., Gilgen, A. K., Michna, P., Plüss, P., Buchmann, N., and Eugster, W.: Management and climate impacts on net CO2 fluxes and carbon budgets of three grasslands along an elevational gradient in Switzerland, Agr. Forest Meteorol., 150, 519–530, https://doi.org/10.1016/j.agrformet.2010.01.011, 2010.
Zimmermann, M., Leifeld, J., and Fuhrer, J.: Quantifying soil organic carbon fractions by infrared-spectroscopy, Soil Biol. Biochem., 39, 224–231, https://doi.org/10.1016/j.soilbio.2006.07.010, 2007.
Zimmermann, P., Tasser, E., Leitinger, G., and Tappeiner, U.: Effects of land-use and land-cover pattern on landscape-scale biodiversity in the European Alps, Agr. Ecosyst. Environ. 139, 13–22, https://doi.org/10.1016/j.agee.2010.06.010, 2010.