the Creative Commons Attribution 4.0 License.
the Creative Commons Attribution 4.0 License.
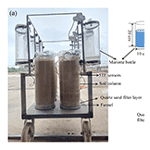
The higher relative concentration of K+ to Na+ in saline water improves soil hydraulic conductivity, salt-leaching efficiency and structural stability
Sihui Yan
Tibin Zhang
Binbin Zhang
Tonggang Zhang
Yu Cheng
Chun Wang
Min Luo
Hao Feng
Kadambot H. M. Siddique
Soil salinity and sodicity caused by saline water irrigation are widely observed globally. Clay dispersion and swelling are influenced by sodium (Na+) concentration and electrical conductivity (EC) of soil solution. Specifically, soil potassium (K+) also significantly affects soil structural stability, but for which concern was rarely addressed in previous studies or irrigation practices. A soil column experiment was carried out to examine the effects of saline water with different relative concentrations of K+ to Na+ (K+ Na+), including K+ Na+ of 0:1 (K0Na1), 1:1 (K1Na1) and 1:0 (K1Na0) at a constant EC (4 dS m−1), and deionized water as the control (CK), on soil physicochemical properties. The results indicated that at the constant EC of 4 dS m−1, the infiltration rate and water content were significantly (P<0.05) affected by K+ Na+ values, and K0Na1, K1Na1 and K1Na0 significantly (P<0.05) reduced saturated hydraulic conductivity by 43.62 %, 29.04 % and 18.06 %, respectively, compared with CK. The volumetric water content was significantly (P<0.05) higher in K0Na1 than CK at both 15 and 30 cm soil depths. K1Na1 and K1Na0 significantly (P<0.05) reduced the desalination time and required leaching volume. K0Na1 and K1Na1 reached the desalination standard after the fifth and second infiltration, respectively, as K1Na0 did not exceed the bulk electrical conductivity required for the desalination prerequisite throughout the whole infiltration cycle at 15 cm soil layer. Furthermore, due to the transformation of macropores into micropores spurred by clay dispersion, soil total porosity in K0Na1 dramatically decreased compared with CK, and K1Na0 even increased the proportion of soil macropores. The higher relative concentration of K+ to Na+ in saline water was more conducive to soil aggregate stability, alleviating the risk of macropores reduction caused by sodicity.
- Article
(3427 KB) - Full-text XML
- BibTeX
- EndNote
Freshwater shortage resulting from elevated demand for water resources as well as the irrational exploitation and use after economic and population growth (Zhang and Xie, 2019; Prajapati et al., 2021) constrains the sustainability of agricultural production (Aparicio et al., 2019). Alternative water resources with variable water quality (such as saline water) are being considered for agricultural irrigation in several desert and saline areas (Singh et al., 2021; Liu et al., 2022a). Utilizing saline water could partly alleviate the undersupply of freshwater for agricultural production (Yang et al., 2020). However, the other side of the coin is that saline water irrigation could result in soil salinization and/or sodicity. Once the soil is salinized and/or alkalized, soil hydraulic properties, like infiltration rate, saturated hydraulic conductivity and permeability, will change inevitably (Scudiero et al., 2017). And cations in the soil solution change the soil structural characteristics through the soil clay-particle dispersion and flocculation (Bouksila et al., 2013; Hack-ten Broeke et al., 2016; Zhang et al., 2018). Therefore, in order to optimize saline water utilization, the effects of saline water quality on the soil hydraulic properties and pore structure characteristics should be paid more attention.
Saline water irrigation can increase the monovalent ions concentration in soil solution and affect soil structure (Qadir et al., 2007, 2021). Excess sodium (Na+) from saline irrigation water is adsorbed onto the clay surface in salt-affected soils where sodium compounds predominate contributing to the disintegration of soil structure (Marchuk and Rengasamy, 2011; Belkheiri and Mula, 2013; Awedat et al., 2021). As percolation progresses, the thickness of the diffusion double-electron layers increases due to the relatively larger hydrated radius of Na+, and the repulsive force between adjacent diffusion double-electron layers appears to increase, resulting in the dispersion and swelling of soil particles (Alva et al., 1991; Reading et al., 2015).
Soil calcium (Ca2+) and magnesium (Mg2+) can alleviate soil dispersibility by replacing Na+ in soil colloids, the outer layers of the Ca2+ and Mg2+ containing colloidal particles do not adsorb water molecules, turning Na+ qualitative hydrophilic colloid into Ca2+ and Mg2+ hydrophobic colloids (Marchuk and Rengasamy, 2011; Tsai et al., 2012). Colloidal particles move closer to each other, promoting soil particles to form water-stable aggregates, thus improving soil structural stability (Gharaibeh et al., 2009; McKenna et al., 2019). Therefore, the concentration of Na+ in relation to Mg2+ and Ca2+ (sodium adsorption ratio, SAR) (US Salinity Laboratory Staff 1954) is a crucial criterion for soil structural stability and hydraulic conductivity (Rengasamy and Marchuk, 2011). Although SAR can be used to predict soil clay dispersion effect caused by cations, the controlling mechanism of dispersion in SAR is presumed to be exchangeable Na+. However, Na+ does not alone cause soil dispersion, since the chemical component of clay structure integrity is mainly a function of ionic valence and hydration radius (Marchuk et al., 2014). Potassium (K+) has been overlooked because salt-affected soils typically contain low amounts of K+. However, Li et al. (2022) reported that under the continuous recycling use of underground saline water, water-soluble and exchangeable K+ is higher than Ca2+ and Mg2+ in the Hetao irrigation district – one of the large irrigation districts in China. It is anticipated that the long-term use of irrigation water with high K+ concentrations may therefore create substantial challenges in preserving good soil structure and adequate infiltration rates (Sposito et al., 2016). K+ is not as effective as Na+ in generating soil particle dispersion and swelling problems, yet Marchuk and Marchuk (2018) pointed out that K+ could substitute Na+ on exchange sites to encourage Na+ leaching and increase water conductivity to some extent. A lower concentration of K+ may have positive effects on soil permeability due to the substitution of exchangeable Na+ by K+ with lower dispersive potential, increasing aggregates stability and soil pore connectivity (Buelow et al., 2015). Traditional SAR ignored the role of K+, and a newly proposed equation, the cation ratio of soil structural stability (CROSS), could integrate the effects of Na+ and K+ in soil, which is an important indicator for assessing the quality of saline water (Rengasamy and Marchuk, 2011).
Thus, we hypothesized that the amount of K+ relative to Na+ would certainly have an effect on soil structural stability, which could be evaluated in a column experiment under controlled conditions. The specific objectives of this study were to (1) ascertain the effect of irrigation saline water with different relative concentrations of K+ to Na+ (K+ Na+) on transport and distribution of water and salt, (2) determine the effect on soil pore structural characteristics, and (3) predict these effects using a newly proposed index (CROSS) rather than SAR.
2.1 Soil sampling location and properties
The study soil was collected from a layer of 0–40 cm field in Yangling (108∘04′ E, 34∘20′ N), Shaanxi Province, China. After being air-dried, the soil was grounded to pass through a 2 mm sieve. The soil's physical and chemical properties are listed in Table 1. Soil particle size distribution was measured by the Laser Mastersizer 2000 (Malvern Instruments; Malvern, UK), and according to the international classification system, soil texture was classified as silty clay. Soil bulk density was calculated using the soil core method. ECe and pH were measured using a conductivity meter (DDS-307; China) and pH meter (PHS-3C; China), respectively. Total soluble salts refer to the total amount of soluble salts in soil-saturated paste extract. Flame photometry (6400A; China) was used to measure soluble Na+ and K+, concentrations of CO and HCO were tested using the neutral titration method, Cl− was analyzed using the silver nitrate titration method, SO was determined using barium sulfate turbidimetric method, and Mg2+ and Ca2+ were specified using ethylene diamine tetraacetic acid (EDTA) titrimetric method (Bao, 2005).
2.2 Experimental design
Soil columns were prepared using transparent polyvinyl chloride cylinders, with an internal diameter of 20 cm and a height of 50 cm (Fig. 1). Round and small holes (6 mm diameter) were arranged equally at the bottom of each cylinder for drainage. A 5 cm depth quartz sand was laid at the bottom of the soil column as a filter layer before packing to prevent small soil particles from being washed away. After that, air-dried soil was packed at 40 cm height with a bulk density of 1.35 g cm−3 (referring to the original level of the soil). The sieved, dry soil was poured into each soil column in the 5 cm sections for uniform compaction, and the layer's surface was roughened to ensure a tight connection to the next layer. The soil column was then allowed to stand in the laboratory for 24 h before starting the experiments described herein. The constant water head (2 cm, using a Mariotte bottle) infiltration experiment was conducted with three replications for each treatment.
Three infiltration solutions were prepared with different ratios of K+ Na+ [(0:1 (K0Na1), 1:1 (K1Na1), 1:0 (K1Na0)] at constant EC of 4 dS m−1, with deionized water being used as the control (CK) (Table 2). The cation ratio of soil structural stability (CROSS) (Rengasamy and Marchuk, 2011) is an indicator of soil structural behavior as influenced by both Na+ and K+, and it was calculated as follows (Smith et al., 2015):
where the chemical element symbols denote charge concentrations (mmolc L−1).
Table 2Saline water settings with different K+ Na+ at a constant EC.
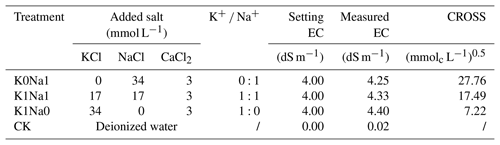
K0Na1, K1Na1 and K1Na0 indicate the saline water at EC of 4 dS m−1 with K+ Na+ of 0:1, 1:1 and 1:0, respectively. CK is deionized water. CROSS is cation ratio of soil structural stability.
The experiment implemented alternate leaching, as the prolonged leaching process of soil substrates is more helpful in illuminating the function of electrolyte effect and cation exchange (Shaygan et al., 2017). The next infiltration was performed 2 d after the drainage of the previous infiltration was completed. Soil layers were regarded as reaching the desalination prerequisite when the soil salt content came to less than 0.3 %, which meant that bulk electrical conductivity was less than 1.5 dS m−1 (transformation from salt content to bulk electrical conductivity) (Yin et al., 2022). Water application was stopped when the bulk electrical conductivity of all treatments at 15 cm depth reached the prerequisite for desalination. This experiment was planned to fill all the pores in the soil column throughout the infiltration cycle; therefore the water applied at the first infiltration was described by the pore volume equation (Huang and Xu, 2010) as follows:
where Vp is the pore volume (cm3), Vs is the volume of filled soil (cm3), TP is the soil total porosity (%), ds is the soil particle density (2.65 g cm−3) (Huang and Xu, 2010) and BD is the bulk density (g cm−3). According to Eqs. (2) and (3), around 6 L of water was required in the first infiltration. Required water volume for each subsequent leaching was determined by the volume of leachate at the first infiltration; it was 0.5 L each time.
2.3 Soil property measurements
During the whole experimental period, soil volumetric water content and bulk electrical conductivity were real-time monitored at 15 and 30 cm soil depths from the soil surface by capacitance sensors (ECH2O 5TE, METER Group; USA) (Fig. 1). Leachate was collected in the leachate catcher below the soil column. Cumulative leachate volume was monitored over time to determine the saturated hydraulic conductivity (Ksat; cm min−1) of each treatment by using a derivation of Darcy's approach (Sahin et al., 2011) as follows:
where Vl is the leachate volume (cm3), H is the length of filled soil (cm), A is the surface area of soil column (cm2), t is the leaching time of measurement (min) and h is the height of constant water head (cm).
To determine the amount of salt released, we measured the volume and EC of the leachate. The leachate was collected at 3 h intervals when the leachate started to drain, and leachate was stored in 100 mL wide-mouth polypropylene reagent bottles. The salt accumulated in the soil column was determined by subtracting the salt in the leachate from the applied water, the salination rate (Rs, %) indicated the ratio of salt accumulated in the soil column at every time of infiltration to the salt content at the first applied water. Leaching efficiency (Le; g L−1) refers to the amount of desalination per unit of water volume in the desalination process. Rs and Le were calculated as follows:
where ms is the salt content accumulated in soil column at each infiltration (g), mw is the salt content in the total water used for the first infiltration (g), m1 is the mass of salts after the first infiltration (g) and w is the total water volume used for leaching (L).
Soil samples were collected from each soil column at 5 cm intervals with the 0–40 cm soil layer 3 d after the final infiltration. Soil BD was calculated using the soil core method, and TP was calculated by Eq. (3) based on BD. The soil water characteristics' curve was measured with the high-velocity centrifugal method (CR21 Hitachi; Japan) and calibrated by RETC software (PC Progress Inc.; Prague, Czech Republic). Currently, several defining sizes of macropores are proposed, rather than a precise definition and pore size range (Cameira et al., 2003; Kim et al., 2010; Hu et al., 2018; Budhathoki et al., 2022; Aldaz-Lusarreta et al., 2022). In this study, macropores were defined as the pores with diameters larger than 1 mm, whereas micropores were defined as smaller than 1 mm (Luxmoore, 1981; Wilson and Luxmoore, 1988). Based on the capillary pressure data, the relationship between pore diameter (d; mm) and water suction (S; Pa) was described according to the capillary bundle model (Jury et al., 1991):
2.4 Statistical analysis
Statistical analysis among all treatments with different K+ Na+ was performed in SPSS 22.0 software, using one-way analysis of variance (ANOVA) based on the least significant difference (LSD) test at 95 % significance level (P<0.05). All figures were created through Origin 2022b.
3.1 Soil-saturated hydraulic conductivity (Ksat)
The K0Na1, K1Na1 and K1Na0 significantly (P<0.05) reduced Ksat by 43.62 %, 29.04 % and 18.06 % compared with CK, respectively (Fig. 2). Additionally, Ksat was negatively correlated with CROSS of saline water, and increasing the CROSS of the applied saline water generally reduced Ksat.
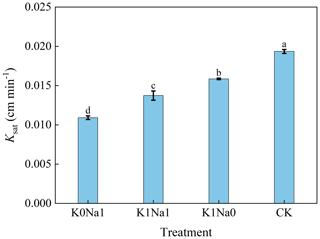
Figure 2Saturated hydraulic conductivity (Ksat) under different treatments. K0Na1, K1Na1 and K1Na0 indicate the saline water at EC of 4 dS m−1 with K+ Na+ of 0:1, 1:1 and 1:0, respectively. CK is deionized water. Different letters after the means of Ksat indicate statistical differences (P<0.05) among treatments based on LSD. Bars indicate standard deviations of means.
3.2 Soil water content
Water content increased immediately after each infiltration in all treatments and then gradually decreased, and the degree of variation tends to stabilize (Fig. 3). Also, water content at deeper soil depths was greater than at shallow soil depths at the same time during the whole infiltration period. The water content ranged from 0.39–0.41 and 0.40–0.42 cm3 at 15 and 30 cm soil depths, respectively. K0Na1 had the highest water content at both 15 and 30 cm soil depths. K1Na1 and K1Na0 were greater than CK at 15 cm soil depth and lower than CK at 30 cm soil depth, and the water content of K1Na1 was higher than K1Na0 at both 15 and 30 cm soil layers.
3.3 Soil salination rate (Rs) and leaching efficiency (Le)
The Rs and Le under CK were not shown in Fig. 4; because deionized water was used as the control and there was almost no salt contained in the study soil, CK was considered negligible for salt accumulation and leaching. Rs peaked at the first infiltration, and approximately 70 %–80 % of the salt in the saline water was retained in the soil column, after which the subsequent leaching had lower Rs values (Fig. 4). The lower the relative concentration of K+ to Na+, the larger soil Rs. Among the three saline water treatments, K1Na0 had the lowest Rs and highest Le at five infiltrations.
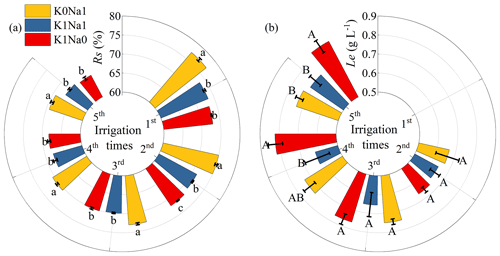
Figure 4Salination rate (Rs) (a) and leaching efficiency (Le) (b) at five infiltrations under all saline water treatments. K0Na1, K1Na1 and K1Na0 indicate the saline water at EC of 4 dS m−1 with K+ Na+ of 0:1, 1:1 and 1:0, respectively. Different lowercase letters followed by means of Rs indicate statistical differences (P<0.05) among treatments based on LSD, and different uppercase letters followed by means of Le indicate statistical differences (P<0.05) among treatments based on LSD. Bars indicate standard deviations of means.
3.4 Soil bulk electrical conductivity
Bulk electrical conductivity of K0Na1, K1Na1 and K1Na0 ranged from 1.0–2.0 dS m−1 at 15 cm and 1.5–2.5 dS m−1 at 30 cm soil depth (Fig. 5). After the first infiltration, bulk electrical conductivity in 15 cm soil depth peaked and then exhibited a general downward trend in the following infiltrations. However, more salts were leached to deeper layers, where salt began to accumulate instead of desalination occurring, and bulk electrical conductivity at 30 cm soil depth gradually increased following the infiltration events. Overall, K0Na1 had the highest bulk electrical conductivity among all treatments at both 15 and 30 cm, and K1Na1 was higher than K1Na0.
At 15 cm soil depth, K0Na1 reached the soil desalination prerequisite after the fifth infiltration, while K1Na1 reached the desalination prerequisite after the second infiltration, and K1Na0 did not exceed the desalination prerequisite during the whole infiltration period. Among all saline water treatments, K1Na0 reduced the desalination time and required leaching volume to reach the standard of desalination. K0Na1, K1Na1 and K1Na0 did not meet the desalination prerequisite at 30 cm soil depth, and the increased volume of infiltration water also increased the bulk electrical conductivity.
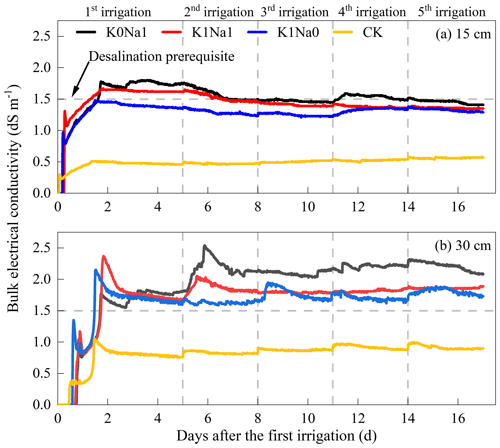
Figure 5Variation of bulk electrical conductivity over time under treatments with different K+ Na+ at constant EC at 15 (a) and 30 cm (b) soil depths in the period of five times of infiltration. K0Na1, K1Na1 and K1Na0 indicate the saline water at EC of 4 dS m−1 with K+ Na+ of 0:1, 1:1 and 1:0, respectively. CK is deionized water.
3.5 Soil bulk density (BD) and total porosity (TP)
Soil BD varied from 1.30–1.40 g cm−3 across all treatments, and BD was below 1.35 g cm−3 at 0–10 and 35–40 cm soil layers; however it was over 1.35 g cm−3 at 10–35 cm soil depth (Fig. 6). K0Na1 significantly (P<0.05) enhanced soil BD throughout the soil column profile compared with CK. TP first diminished with soil depth to reach a minimum at about 30–35 cm and then slightly increased at 35–40 cm. The TP of K1Na1 and K1Na0 slightly improved after five times of infiltration, and only K0Na1 showed a decline compared with CK. Overall, over the whole infiltration period, K1Na0 was most conducive to the formation of soil pore structure and increasing the total pore volume. The saline water with lower CROSS was beneficial for reducing soil BD and increasing TP.
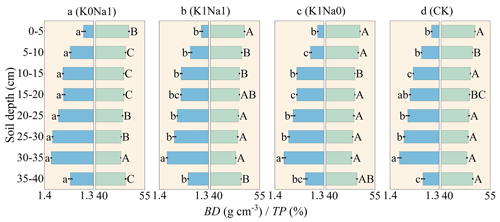
Figure 6Soil bulk density (BD) and total porosity (TP) throughout the soil column profile under different treatments. K0Na1, K1Na1 and K1Na0 indicate the saline water at EC of 4 dS m−1 with K+ Na+ of 0:1, 1:1 and 1:0, respectively. CK is deionized water. The blue horizon columns represent BD, while the green horizon columns represent TP. Different lowercase letters followed by means of BD indicate statistical differences (P<0.05) among treatments based on LSD, and different uppercase letters followed by means of TP indicate statistical differences (P<0.05) among treatments based on LSD. Bars indicate standard deviations of means.
3.6 Proportion of micropores and proportion of macropores
Micropores were the most dominant pores for all treatments: the volume of micropores accounted for more than 40 % of the total soil volume; however, the proportion of macropores did not exceed 8 % (Fig. 7). The 0–5 cm soil layer had the lowest proportion of macropores and retained the largest proportion of micropores compared with other depths. K0Na1 had the highest proportion of micropores and the lowest proportion of macropores. K1Na0 had a greater proportion of macropores in the soil column than CK.
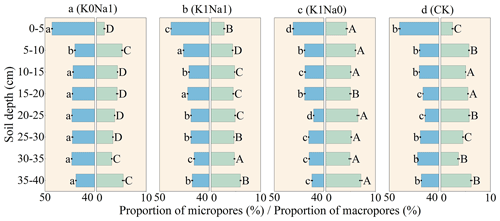
Figure 7Proportion of micropores and proportion of macropores in total soil volume throughout the soil column profile under different treatments. K0Na1, K1Na1 and K1Na0 indicate the saline water at EC of 4 dS m−1 with K+ Na+ of 0:1, 1:1 and 1:0, respectively. CK is deionized water. The blue horizon columns represent proportion of micropores, while the green horizon columns represent proportion of macropores. Different lowercase letters followed by means of proportion of micropores indicate statistical differences (P<0.05) among treatments based on LSD, and different uppercase letters followed means of proportion of macropores indicate statistical differences (P<0.05) among treatments based on LSD. Bars indicate standard deviations of means.
4.1 Effects of saline water on soil water movement and redistribution
As a crucial soil hydraulic characteristic, Ksat reflects the transportation ability of water and solutes (Braud et al., 2001; Maillard et al., 2011; Albalasmeh et al., 2022). The cation composition and EC of soil solution affect Ksat by controlling electrostatic repulsive pressure through surface potential and midpoint potential between adjacent particles and consequently influence water movement (Fares et al., 2000; Liu et al., 2022b). Specifically, the relative concentration of K+ to Na+ in saline water was related to the swelling and dispersion of soil particles (Yu et al., 2016; Zhu et al., 2019). Dispersed clay particles clogged soil macropores to subsequently restrict water transport (Awedat et al., 2021). The Na+ has a relatively higher ionicity index than K+; as a result, the low relative concentration of K+ to Na+ decreased the degree of covalency in clay–cation bonds, which was detrimental to clay particles aggregation (Marchuk and Rengasamy, 2011). Therefore, in our study, the high relative concentration of K+ to Na+ promoted the flocculation and stabilization of soil clay particles, resulting in an increased water hydraulic conductivity (Fig. 2).
After a certain period of irrigation, soil moisture redistributed at different depths of soil column (Fig. 3). Soil water moved further down during the phase of water redistribution soon after each irrigation, reducing the water content in the upper soil layers. As the upper soil layers drained, the lower soil layers still had water inflow (Kargas et al., 2021), increasing the water content in the lower soil layers. The results also implicated that the retention of soil water by Na+ was stronger than that by K+; the cause may be that Na+ can increase the thickness of the diffuse double layers around soil colloids theoretically due to its larger hydrated radius and lower charge than K+, and the adjacent double layers overlapped to provide more space between layers (He et al., 2015), where, subsequently, more water can be retained (Fig. 3).
Additionally, our study showed that K1Na1 was even more beneficial than deionized water for water downward transport (Fig. 3), which was because deionized water (CK) (below 0.2 dS m−1) tended to leach soluble minerals and salts, especially Ca2+, from the surface soil layers. This would lead to the reduction of its original, solid soil structural stability. In the absence of salt and Ca2+, the dispersed tiny particles filled the smaller pore spaces in soil, reducing even more channels for water flow and exacerbating water retention in deeper soil layers (Ayers and Westcot, 1985). However, a lower concentration of soluble salts could increase colloid flocculation and thereby improve soil aeration and water conductivity (Tang and She, 2016).
4.2 Effects of saline water on soil salination and desalination process
Numerous factors influence soil salt-leaching efficiency; for example, increasing EC and reducing SAR definitely improve clay flocculation, which can enhance salt leaching (Ebrahim Yahya et al., 2022). Na+ is more likely to trigger soil clay dispersion and swelling than K+; thus Na+ generally inhibits water infiltration, which is detrimental to salt leaching (Smiles and Smith, 2004). Adding K+ could promote displacement of the adsorbed Na+ and then decrease Na+ concentration and salt accumulation in soil solute through leaching.
A greater reduction in Na+ concentration was associated with a higher rate of cation exchange rate, and the slow rate of solute leaching from aggregates reduced the total leaching efficiency (Shaygan et al., 2017). During the leaching process, water flow preferentially passed through the macropores rather than aggregates. The slow water transportation through aggregates induced the slow removal of solutes from the aggregates, leading to a reduced leaching efficiency. In our study, the alternate leaching was implemented to improve solute leaching (Figs. 4 and 5). The soil solutes diffused into the aggregates surface during the rest period, improving salt leaching due to the water flow in macropores (Al-Sibai et al., 1997). Saline water with more K+ could increase the magnitude of cation exchange due to the substitution of Na+ on exchange sites by K+ with lower dispersive potential (Shaygan et al., 2017); the intensive release of cations from the soil further improved salt's leaching efficiency. In addition, the integrity of soil aggregates created by combining clay particles and the other soil components enhanced by K+ can benefit solute transportation (Marchuk and Rengasamy, 2011).
4.3 Effects of saline water on soil pore structure characteristics
Due to the long-term continuous irrigation, the upper layer of soil was immersed in water, resulting in the swelling and loosening of soil particles (Vaezi et al., 2017; Håkansson and Lipiec, 2000), which caused the reduction of BD in the surface layer (Fig. 6). The subsoil BD increased with depth under the impact of water pressure and self weight due to the declining pore diameter and pore branching closure (Schjønning et al., 2013). And for soil at the bottom, the loss of soil particles from small holes was responsible for the abrupt reduction in BD (Fig. 6). The value of CROSS in saline water could reflect changes in soil BD and TP, in agreement with the result of Marchuk and Marchuk (2018). The high CROSS implied an increase in the proportion of monovalent exchangeable cations, thickening the double layer at the interface between the clay surface and soil solution. Hence, soil swelling occurred at the expense of water-conducting pores. Additionally, aggregates slaking and subsequent clay dispersion and deposition of clay particles within the pore space contributed to the reduction in TP (Marchuk and Marchuk, 2018).
Soil macropores play a crucial role in water and solute transport, accounting for 85 % of the total infiltration volume (Wilson and Luxmoore, 1988; Weiler and Naef, 2003; Kotlar et al., 2020). For saline water with the same EC, a decrease in K+ concentration may enhance soil clay dispersion, resulting in the loosening of clay particles from the aggregates. The dispersed clay particles moving with the water could clog the soil macropores, promoting the conversion of macropores to micropores (Cameira et al., 2003), thus reducing the macropore volume (Fig. 7).
We explored the effects of the relative ratio of K+ to Na+ in saline water on soil hydraulic characteristics and structural stability via a soil column experiment. Irrigation with saline water of K+ Na+ of 1:0 caused fewer pore blockages due to soil clay particle dispersion than 0:1, which increased soil-saturated hydraulic conductivity. The presence of K+ accelerated the sustained Na+ replacement and leaching, alleviating salt accumulation and enhancing leaching efficiency. K+ positively affected the establishment of soil structure due to the transformation of micropores into macropores, and the ever-increasing unobstructed water-conducting channels sped up water and solute transport. The rational use of saline water with adequate K+ could help mitigate the structural deterioration caused by Na+. Appropriate adjustment of the relative concentration of K+ to Na+ in saline water during infiltration could ameliorate soil structural properties. In addition to Ca2+ and Mg2+ (primary concerns in earlier studies), the relative concentration of K+ to Na+ is an essential indicator for assessing the suitability of saline water quality for irrigation and should be considered when using saline water.
The data will be available upon reasonable request.
SY and TZ conceived and designed the experiments. SY, BZ and TZ led the data processing and statistical analysis, SY, YC, CW and ML performed the experiments. SY wrote the initial draft. HF and KHMS contributed to review and editing of the paper.
The contact author has declared that none of the authors has any competing interests.
Publisher’s note: Copernicus Publications remains neutral with regard to jurisdictional claims in published maps and institutional affiliations.
We are grateful to the reviewers and editors who made constructive comments on this paper.
This work was supported by the National Key R&D Program of China (grant no. 2021YFD1900700), the Innovation Capability Support Program of Shaanxi (grant no. 2022PT-23), the Key R&D Program of Shaanxi (grant no. 2023-ZDLNY-53), and China 111 project (B12007).
This paper was edited by Simeon Materechera and reviewed by Manfred Sager and two anonymous referees.
Albalasmeh, A., Mohawesh, O., Gharaibeh, M., Deb, S., Slaughter, L., and El Hanandeh, A.: Artificial neural network optimization to predict saturated hydraulic conductivity in arid and semi-arid regions, Catena, 217, 106459, https://doi.org/10.1016/J.CATENA.2022.106459, 2022.
Aldaz-Lusarreta, A., Giménez, R., Campo-Bescós, M. A., Arregui, L. M., and Virto, I.: Effects of innovative long-term soil and crop management on topsoil properties of a Mediterranean soil based on detailed water retention curves, SOIL, 8, 655–671, https://doi.org/10.5194/soil-8-655-2022, 2022.
Al-Sibai, M., Adey, M. A., and Rose, D. A.: Movement of solute through a porous medium under intermittent leaching, Eur. J. Soil Sci., 48, 711–725, https://doi.org/10.1046/j.1365-2389.1997.00126.x, 1997.
Alva, A. K., Sumner, M. E., and Miller, W. P.: Relationship between ionic strength and electrical conductivity for soil solutions, Soil Sci., 152, 239–242, https://doi.org/10.1097/00010694-199110000-00001, 1991.
Aparicio, J., Tenza-Abril, A. J., Borg, M., Galea, J., and Candela, L.: Agricultural irrigation of vine crops from desalinated and brackish groundwater under an economic perspective, A case study in Siġġiewi, Malta, Sci. Total Environ., 650, 734–740, https://doi.org/10.1016/j.scitotenv.2018.09.059, 2019.
Awedat, A. M., Zhu, Y., Bennett, J. M., and Raine, S. R.: The impact of clay dispersion and migration on soil hydraulic conductivity and pore networks, Geoderma, 404, 115297, https://doi.org/10.1016/J.GEODERMA.2021.115297, 2021.
Ayers, R. S. and Westcot, D. W.: Water Quality for Agriculture, FAO Irrigation and Drainage Paper 29 Rev 1, Rome, ISBN 92-5-102263-1, Italy.
Bao, S. D.: Soil Analysis in Agricultural Chemistry, China Agricultural Press ,Beijing, China, ISBN 9787109066441, 2005 (in Chinese).
Belkheiri, O. and Mulas, M.: The effects of salt stress on growth, water relations and ion accumulation in two halophyte Atriplex species, Environ. Exp. Bot., 86, 17–28, https://doi.org/10.1016/j.envexpbot.2011.07.001, 2013.
Bouksila, F., Bahri, A., Berndtsson, R., Persson, M., Rozema, J., and Van der Zee, S. E. A. T. M.: Assessment of soil salinization risks under irrigation with brackish water in semiarid Tunisia, Environ. Exp. Bot., 92, 176–185, https://doi.org/10.1016/j.envexpbot.2012.06.002, 2013.
Braud, I., Vich, A. I. J., Zuluaga, J., Fornero, L., and Pedrani, A.: Vegetation influence on runoff and sediment yield in the Andes region: observation and modelling, J. Hydrol., 254, 124–144, https://doi.org/10.1016/S0022-1694(01)00500-5, 2001.
Budhathoki, S., Lamba, J., Srivastava, P., Malhotra, K., Way, T. R., and Katuwal, S.: Using X-ray computed tomography to quantify variability in soil macropore characteristics in pastures, Soil Tillage Res., 215, 105194, https://doi.org/10.1016/J.STILL.2021.105194, 2022.
Buelow, M. C., Steenwerth, K., and Parikh, S. J.: The effect of mineral-ion interactions on soil hydraulic conductivity, Agr. Water. Manage., 152, 277–285, https://doi.org/10.1016/j.agwat.2015.01.015, 2015.
Cameira, M. R., Fernando, R. M., and Pereira, L. S.: Soil macropore dynamics affected by tillage and irrigation for a silty loam alluvial soil in southern Portugal, Soil Tillage Res., 70, 131–140, https://doi.org/10.1016/S0167-1987(02)00154-X, 2003.
Ebrahim Yahya, K., Jia, Z., Luo, W., Yuanchun, H., and Ame, M. A.: Enhancing salt leaching efficiency of saline-sodic coastal soil by rice straw and gypsum amendments in Jiangsu coastal area, Ain Shams Eng. J., 13, 101721, https://doi.org/10.1016/J.ASEJ.2022.101721, 2022.
Fares, A., Alva, A. K., Nkedi-Kizza, P., and Elrashidi, M. A.: Estimation of soil hydraulic properties of a sandy soil using capacitance probes and Guelph Permeameter, Soil Sci. Soc. Am. J., 165, 768–777, https://doi.org/10.1097/00010694-200010000-00002, 2000.
Gharaibeh, M. A., Eltaif, N. I., and Shunnar, O. F.: Leaching and reclamation of calcareous saline-sodic soil by moderately saline and moderate-SAR water using gypsum and calcium chloride, J. Plant Nutr. Soil Sci., 172, 713–719, https://doi.org/10.1002/jpln.200700327, 2009.
Hack-ten Broeke, M. J. D., Kroes, J. G., Bartholomeus, R. P., van Dam, J. C., de Wit, A. J. W., Supit, I., Walvoort, D. J. J., van Bakel, P. J. T., and Ruijtenberg, R.: Quantification of the impact of hydrology on agricultural production as a result of too dry, too wet or too saline conditions, SOIL, 2, 391–402, https://doi.org/10.5194/soil-2-391-2016, 2016.
Håkansson, I. and Lipiec, J.: A review of the usefulness of relative bulk density values in studies of soil structure and compaction, Soil Tillage Res., 53, 71–85, https://doi.org/10.1016/S0167-1987(99)00095-1, 2000.
He, Y. B., Desutter, T. M., Casey, F., Clay, D., Franzen, D., and Steele, D.: Field capacity water as influenced by Na and EC: Implications for subsurface drainage, Geoderma, 245, 83–88, https://doi.org/10.1016/j.geoderma.2015.01.020, 2015.
Hu, X., Li, Z. C., Li, X. Y., Wang, P., Zhao, Y. D., Liu, L. Y., and Lü, Y. L.: Soil macropore structure characterized by X-Ray computed tomography under different land uses in the Qinghai Lake watershed, Qinghai-Tibet plateau, Pedosphere, 28, 478–487, https://doi.org/10.1016/S1002-0160(17)60334-5, 2018.
Huang, C. Y and Xu, J. M.: Soil Science, China Agriculture Press, 40 Beijing, China, 2010 (in Chinese).
Jury, W., Gardner, W. R., and Gardner, W. H.: Soil Physics, John Wiley and Sons, New York, USA, ISBN 9780471059653, 1991.
Kargas, G., Soulis, K. X., and Kerkides, P.: Implications of hysteresis on the horizontal soil water redistribution after infiltration, Water, 13, 2773–2773, https://doi.org/10.3390/W13192773, 2021.
Kim, H., Anderson, S. H., Motavalli, P. P., and Gantzer, C. J.: Compaction effects on soil macropore geometry and related parameters for an arable field, Geoderma, 160, 244–251, https://doi.org/10.1016/j.geoderma.2010.09.030, 2010.
Kotlar, A. M., de Jong van lier, Q., Andersen, H. E., Nørgaard, T., and Iversen, B. V.: Quantification of macropore flow in Danish soils using near-saturated hydraulic properties, Geoderma, 375, 114479, https://doi.org/10.1016/j.geoderma.2020.114479, 2020.
Li, Z. Y., Cao, W. G., Wang, Z. R., Li, J. C., and Ren, Y.: Hydrochemical characterization and irrigation suitability analysis of shallow groundwater in Hetao Irrigation District, Inner Mongolia., Geoscience, 36, 418–426, https://doi.org/10.19657/j.geoscience.1000-8527.2022.012, 2022 (in Chinese).
Liu, B. X., Wang, S. Q., Liu, X. J., and Sun, H. Y.: Evaluating soil water and salt transport in response to varied rainfall events and hydrological years under brackish water irrigation in the North China Plain, Geoderma, 422, 115954, https://doi.org/10.1016/J.GEODERMA.2022.115954, 2022a.
Liu, X. M., Zhu, Y. C., Mclean Bennett, J., Wu, L. S., and Li, H.: Effects of sodium adsorption ratio and electrolyte concentration on soil saturated hydraulic conductivity, Geoderma, 414, 115772, https://doi.org/10.1016/J.GEODERMA.2022.115772, 2022b.
Luxmoore, R. J.: Micro-, meso-, and macroporosity of soils, Soil Sci. Soc. Am. J., 45, 671–672, https://doi.org/10.2136/sssaj1981.03615995004500030051x, 1981.
Maillard, E., Payraudeau, S., Faivre, E., Grégoire, C., Gangloff, S., and Imfeld, G.: Removal of pesticide mixtures in a stormwater wetland collecting runoff from a vineyard catchment, Sci. Total Environ., 409, 2317–2324, https://doi.org/10.1016/j.scitotenv.2011.01.057, 2011.
Marchuk, A., Marchuk, S. A., Bennett, J. A., Eyres, M. A., and Scott, E.: An alternative index to ESP to explain dispersion occurring in Australian soils when Na content is low, National Soils Conference: Proceedings of the 2014 National Soils Conference Soil Science Australia Melbourne, Australia, PhD, 2014.
Marchuk, A. and Rengasamy, P.: Clay behaviour in suspension is related to the ionicity of clay–cation bonds, Appl. Clay Sci., 53, 754–759, https://doi.org/10.1016/j.clay.2011.05.019, 2011.
Marchuk, S. and Marchuk, A.: Effect of applied potassium concentration on clay dispersion, hydraulic conductivity, pore structure and mineralogy of two contrasting Australian soils., Soil Tillage Res., 182, 35–44, https://doi.org/10.1016/j.still.2018.04.016, 2018.
Mckenna, B. A., Kopittke, P. M., Macfarlane, D. C., Dalzell, S. A., and Menzies, N. W.: Changes in soil chemistry after the application of gypsum and sulfur and irrigation with coal seam water, Geoderma, 337, 782–791, https://doi.org/10.1016/j.geoderma.2018.10.019, 2019.
Prajapati, M., Shah, M., and Soni, B.: A review of geothermal integrated desalination: A sustainable solution to overcome potential freshwater shortages, J. Clean. Prod., 326, 129412, https://doi.org/10.1016/J.JCLEPRO.2021.129412, 2021.
Qadir, M., Oster, J. D., Schubert, S., Noble, A. D., and Sahrawat, K. L.: Phytoremediation of Sodic and Saline-Sodic Soils, Adv. Agron., 96, 197–247, https://doi.org/10.1016/S0065-2113(07)96006-X, 2007.
Qadir, M., Sposito, G., Smith, C. J., and Oster, J. D.: Reassessing irrigation water quality guidelines for sodicity hazard, Agr. Water. Manage., 255, 107054, https://doi.org/10.1016/J.AGWAT.2021.107054, 2021.
Reading, L. P., Lockington, D. A., Bristow, K. L., and Baumgartl, T.: Are we getting accurate measurements of Ksat for sodic clay soils?, Agr. Water. Manage., 158, 120–125, https://doi.org/10.1016/j.agwat.2015.04.015, 2015.
Rengasamy, P. and Marchuk, A.: Cation ratio of soil structural stability (CROSS), Soil Res., 49, 280–285, https://doi.org/10.1071/SR10105, 2011.
Sahin, U., Eroğlu, S., and Sahin, F.: Microbial application with gypsum increases the saturated hydraulic conductivity of saline–sodic soils, Appl. Soil Ecol., 48, 247–250, https://doi.org/10.1016/j.apsoil.2011.04.001, 2011.
Schjønning, P., Lamandé, M., Berisso, F. E., Simojoki, A., Alakukku, L., and Andreasen, R. R.: Gas diffusion, non-darcy air permeability, and computed tomography images of a clay subsoil affected by compaction, Soil Sci. Soc. Am. J., 77, 1977–1990, https://doi.org/10.2136/sssaj2013.06.0224, 2013.
Scudiero, E., Skaggs, T. H., and Corwin, D. L.: Simplifying field-scale assessment of spatiotemporal changes of soil salinity, Sci. Total Environ., 587, 273–281, https://doi.org/10.1016/j.scitotenv.2017.02.136, 2017.
Shaygan, M., Reading, L. P., and Baumgartl, T.: Effect of physical amendments on salt leaching characteristics for reclamation, Geoderma, 292, 96–110, https://doi.org/10.1016/j.geoderma.2017.01.007, 2017.
Singh, G., Mavi, M. S., Choudhary, O. P., Gupta, N., and Singh, Y.: Rice straw biochar application to soil irrigated with saline water in a cotton-wheat system improves crop performance and soil functionality in north-west India, J. Environ. Manage., 295, 113277, https://doi.org/10.1016/J.JENVMAN.2021.113277, 2021.
Smiles, D. E. and Smith, C. J.: A survey of the cation content of piggery effluents and some consequences of their use to irrigate soils, Soil Res., 42, 231–246, https://doi.org/10.1071/SR03059, 2004.
Smith, C. J., Oster, J. D., and Sposito, G.: Potassium and magnesium in irrigation water quality assessment, Agr. Water. Manage., 157, 59–64, https://doi.org/10.1016/j.agwat.2014.09.003, 2015.
Sposito, G., Oster, J. D., Smith, C. J., and Assouline, S.: Assessing soil permeability impacts from irrigation with marginal-quality waters, AB Reviews: Perspectives in Agriculture, Veterinary Science, Nutr. Nat. Res., 11, 15, https://doi.org/10.1079/PAVSNNR201611015, 2016.
Tang, S. Q. and She, D. L.: Influence of water quality on soil saturated hydraulic conductivity and infiltration properties, Transactions of the Chinese Society for Agricultural Machinery, 47, 108–114, https://doi.org/10.6041/j.issn.1000-1298.2016.10.015, 2016 (in Chinese).
Tsai, W. T., Liu, S. C., Chen, H. R., Chang, Y. M., and Tsai, Y. L.: Textural and chemical properties of swine-manure-derived biochar pertinent to its potential use as a soil amendment, Chemosphere, 89, 198–203, https://doi.org/10.1016/j.chemosphere.2012.05.085, 2012.
Vaezi, A. R., Ahmadi, M., and Cerdà, A.: Contribution of raindrop impact to the change of soil physical properties and water erosion under semi-arid rainfalls, Sci. Total Environ., 583, 382–392, https://doi.org/10.1016/j.scitotenv.2017.01.078, 2017.
Weiler, M. and Naef, F.: Simulating surface and subsurface initiation of macropore flow, J. Hydrol., 273, 139–154, https://doi.org/10.1016/S0022-1694(02)00361-X, 2003.
Wilson, G. V. and Luxmoore, R. J.: Infiltration, macroporosity, and mesoporosity distributions on two forested watersheds, Soil Sci. Soc. Am. J., 52, 329–335, https://doi.org/10.2136/sssaj1988.03615995005200020005x, 1988.
Yang, G., Li, F., Tian, L., He, X., Gao, Y., Wang, Z., and Ren, F.: Soil physicochemical properties and cotton (Gossypium hirsutum L.) yield under brackish water mulched drip irrigation, Soil Tillage Res., 199, 104592, https://doi.org/10.1016/j.still.2020.104592, 2020.
Yin, C. Y., Zhao, J., Chen, X. B., Li, L. J., Liu, H., and Hu, Q. L.: Desalination characteristics and efficiency of high saline soil leached by brackish water and Yellow River water, Agr. Water. Manage., 263, 107461, https://doi.org/10.1016/J.AGWAT.2022.107461, 2022.
Yu, Z. H., Liu, X. M., Xu, C. Y., Xiong, H. L., and Li, H.: Specific ion effects on soil water movement, Soil Tillage Res., 161, 63–70, https://doi.org/10.1016/j.still.2016.03.004, 2016.
Zhang, H. X. and Xie, Y. Z.: Alleviating freshwater shortages with combined desert-based large-scale renewable energy and coastal desalination plants supported by Global Energy Interconnection, Glob. Energy Interconnec., 2, 205–213, https://doi.org/10.1016/j.gloei.2019.07.013, 2019.
Zhang, T. B., Zhan, X. Y., He, J. Q., Feng, H., and Kang, Y. H.: Salt characteristics and soluble cations redistribution in an impermeable calcareous saline-sodic soil reclaimed with an improved drip irrigation, Agr. Water. Manage., 197, 91–99, https://doi.org/10.1016/j.agwat.2017.11.020, 2018.
Zhu, Y., Bennett, J. M., and Marchuk, A.: Reduction of hydraulic conductivity and loss of organic carbon in non-dispersive soils of different clay mineralogy is related to magnesium induced disaggregation, Geoderma, 349, 1–10, https://doi.org/10.1016/j.agwat.2017.11.020, 2019.