the Creative Commons Attribution 4.0 License.
the Creative Commons Attribution 4.0 License.
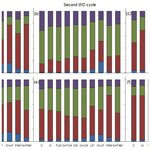
Wetting and drying cycles, organic amendments, and gypsum play a key role in structure formation and stability of sodic Vertisols
Sara Niaz
J. Bernhard Wehr
Ram C. Dalal
Peter M. Kopittke
Neal W. Menzies
In the natural environment, soils undergo wetting and drying (WD) cycles due to precipitation and evapotranspiration. The WD cycles have a profound impact on soil physical, chemical, and biological properties and drive the development of structure in soils. Degraded soils are often lacking structure, and the effect of organic amendments and WD cycles on structure formation of these soils is poorly understood. The aim of this study was to evaluate the role of biotic and abiotic factors on aggregate formation and stabilization of sodic soils after the addition of gypsum and organic amendments (feedlot manure, chicken manure, lucerne pallets, and anionic poly acrylamide). Amended soils were incubated at 25 ∘C over four WD cycles, with assessment of soil microbial respiration, electrical conductivity, pH, sodium adsorption ratio (SAR), aggregate stability in water (ASWAT), aggregate size distribution, and mean weight diameter. Our results demonstrate that WD cycles can improve aggregate stability after the addition of amendments in sodic Vertisols, but this process depends on the type of organic amendment. Lucerne pellets resulted in highest soil microbial respiration, proportions of large macroaggregates (>2000 µm), and mean weight diameter. In contrast, dispersion was significantly reduced when soils were treated with chicken manure, whilst anionic polyacrylamide only had a transient effect on aggregate stability. When these organic amendments were applied together with gypsum, the stability of aggregates was further enhanced, and dispersion became negligible after the second WD cycle. The formation and stability of small macroaggregates (2000–250 µm) was less dependent on the type of organic amendments and more dependent on WD cycles as the proportion of small macroaggregates also increased in control soils after four WD cycles, highlighting the role of WD cycles as one of the key factors that improves aggregation and stability of sodic Vertisols.
- Article
(3314 KB) - Full-text XML
-
Supplement
(424 KB) - BibTeX
- EndNote
Soils are subjected to seasonal and daily variations of water and temperature. These variations effect the physical, biological, and chemical properties of soils. Natural variations in soil water content can lead to wetting and drying (WD) cycles, which are affected by rainfall, solar radiation, capillarity, wind, condensation (Utomo and Dexter, 1982), and evapotranspiration. In most terrestrial ecosystems, surface soils experience rapid changes in soil water content with a longer dry period followed by a relatively rapid rewetting (Borken and Matzner, 2009). Southern Queensland (Australia) usually has hot wet summers with cool dry winters, and soils of this region and other semi-arid areas are particularly susceptible to drying and rewetting stresses due to the infrequency of rainfall. In the field, most soils experience more than one WD cycle throughout the year. These WD cycles have a substantial influence on soil aggregation and structure stabilization because of its direct effect on hydration of minerals, and an indirect effect on plant ecology and soil microbial activity (Denef et al., 2001; Cosentino et al., 2006).
Soil aggregation is an important mechanism for stabilization of soil organic matter (Six et al., 2000a). Furthermore, it also supports soil fertility, as it reduces soil erosion and controls soil aeration, water infiltration, hydraulic conductivity, and nutrient cycling (Oades, 1984; Six et al., 2000b). Soil aggregation is caused by various aggregate stabilizing compounds, which work together at different spatial scales (Tisdall and Oades, 1982). Aggregate formation also depends on soil microbial activity, since the latter influences the production of binding materials such as microbial exudates and hyphae (Rahman et al., 2017). Aggregate stability often exhibits seasonal and inter-annual variability that is controlled mainly by rainfall, temperature, humidity, insolation, and organic matter (Perfect et al., 1990).
Sodic soils are generally characterized as soils with poor structure, which makes those soils difficult to work with when wet or dry (Rengasamy and Olsson, 1991). Poor structural stability of sodic soils restricts seedling emergence and root growth, which directly limits crop growth and development and indirectly affects plant nutrition by limiting water infiltration, nutrient uptake, and gaseous exchange (Curtin and Naidu, 1998). Rehabilitation of sodic soils requires an understanding of the complex interactions between biological and physico-chemical factors that contribute to soil structure formation and stability (Oades, 1993; Nelson and Oades, 1998). Traditionally the management practices used to improve the structure of sodic soils involve the displacement of Na ions from the soil exchange complex with the help of divalent cations such as Ca or increasing the ionic strength of soil solution (Ghosh et al., 2010), both of which can be achieved by the application of gypsum to these soils. The effect of gypsum on increasing ionic strength is immediate but short-lived. In contrast, the effect of gypsum for providing the counter ion (Ca to replace Na) is permanent unless additional Na is added to system (e.g., using poor quality irrigation water). Another frequently-used management practice to ameliorate sodic soils is the use of organic amendments. The addition of organic matter affects aggregate stability within a period of days to weeks due to the stimulation of microbial activity (Six et al., 2004), depending upon the quality and quantity of organic matter (Monnier, 1965 cited in Abiven et al., 2009). While organic matter increases soil microbial respiration resulting in the formation of extracellular polysaccharides which help in the formation of soil aggregates (Bossuyt et al., 2001), studies investigating the effect of organic amendments in improving the soil structure are inconclusive. For instance, the extracellular polysaccharides and large polyanions can bind clay particles into stable macroaggregates. On the other hand, organic anions can enhance dispersion by increasing the negative charge on clay particles and by complexing calcium and other polyvalent cations (such as those of aluminium), hence reducing their activity in soil solution (Ghosh et al., 2010)
Apart from the changes in soil structure due to the addition of different ameliorants, WD cycles can lead to more intensive changes in the structure of soils dominated by smectitic clays (Vertisols) through physical processes (Utomo and Dexter, 1982; Denef et al., 2001). These soils are generally characterized as self-mulching soils, as they exhibit shrink–swell properties imposed by the WD cycles (Pal et al., 2012). Vertisols cover a total of an estimated 340 million ha in the world (Australia, Asia, Africa, and America), out of which approximately 150 million ha is potential crop land. However, the physical properties and moisture regimes of Vertisols represent serious management constraints (Pal et al., 2012). Sodic Vertisols are common in arid parts of the world. The effect of sodicity on the physical properties of Vertisols is still a subject of debate. For example, Rahman et al. (2018) reported that WD cycles improved (increased) the mean weight diameter (MWD, a proxy of aggregate stability measurement) of Vertisols when treated with maize straw after four WD cycles. In a similar manner, WD cycles also increased the proportion of large macroaggregates of smectite Vertisols (Bravo-Garza et al., 2009). Peng et al. (2011), comparing swelling and non-swelling soils, reported that WD cycles decreased the MWD of swelling soils but not of non-swelling soils. However, Six et al. (2000b) found that repeated WD cycles decreased the MWD due to physical disturbance of aggregates, with this being related to the loss of soil organic matter. These apparently contradictory results can potentially be explained on the basis of the initial conditions of the soil, such as the physical conditions of aggregates, organic matter content and quality, and the intensities and duration of the WD cycles (Cosentino et al., 2006).
Similarly, studies investigating the effects of WD cycles on microbial activity were inconclusive, with results differing due to varying experimental designs, incubation period and temperatures, soil properties, and treatments applied. Xiang et al. (2008) found that multiple WD cycles increased the microbial respiration of grassland soils up to 6-fold when compared to the soil that remained wet. Drying and rewetting of these soils gave a new pulse of respiration with each WD cycle, but when these soils were kept at constant water content, microbial respiration decreased to almost zero. An increase in cumulative respiration from forest soils of China was also observed when these soils experience a WD cycle compared to constant moisture conditions (Zhang et al., 2022). In contrast however, Rahman et al. (2018) reported that repeated WD cycles in Vertisols decreased soil respiration significantly but that the magnitude of this decrease became smaller over the time. Similarly, Yu et al. (2014) found that repeated WD cycles decreased the cumulative soil respiration compared to constant moist conditions in a loamy sand soil.
Although the interaction of biotic and abiotic factors and its effect on aggregate stability and formation is complex and inconsistent, little effort has been put into studying the underlying mechanisms, particularly in sodic Vertisols. Furthermore, there is little information available on the relationship between aggregate formation and stability following repeated WD cycles after addition of gypsum and organic amendments. Thus in the present study we used two sodic Vertisols, with the aim to: (i) determine the role of gypsum and different organic amendments on aggregate formation and stability, (ii) explore the combined effect of gypsum and organic amendments on soil physico-chemical and microbial properties, (iii) investigate the effect of WD cycles on microbial respiration, (iv) assess the effects of WD cycles on aggregate formation and stability, and (v) determine how many WD cycles are needed to improve aggregate stability. We hypothesized that (i) organic amendments will increase the microbial respiration and improve the formation of large macroaggregates and MWD, (ii) gypsum will improve aggregate stability due to increases Ca concentration and ionic strength, (iii) organic amendments act synergistically with gypsum on aggregation, and (iv) repeated WD cycles will increase the process of aggregate formation and stability.
2.1 Soils
The two soils used in this experiment were collected from a farm located in southern Queensland near Goondiwindi (28.54∘ S, 150.30∘ E), Australia. The soils were being cropped using a maize (Zea mays) and wheat (Triticum aestivum) rotation system. Both soils were classified as sodic Vertisols according to the FAO World Reference Base (2015) (Vertisols in the Australian Soil Classification). Soils were collected with a shovel to a depth of 10 cm at two locations from the cropped land. These sites were selected as they were in close proximity to each other but were slightly different in regard to their physical properties (Table 1), with Soil 1 being more dispersive than Soil 2. Three core samples were also collected for the measurement of bulk density from each site (Soil 1 and Soil 2) and dried at 105 ∘C for several days. After collection, soils were air dried, and the larger clods gently broken into smaller aggregates by hand. The soils were then passed through a 10 mm sieve to remove stones, visible roots, and plant litter. For chemical analysis, a portion of each soil was sieved to <2 mm. Soil pH (ISO, 2005) and electrical conductivity (EC) (ISO, 1994) were measured in 1:5 soil : water suspension. Particle size analysis was performed using the pipette method (Day, 1965), and field water capacity (−10 kPa) was measured using a pressure plate apparatus (Cassel and Nielsen, 1986). Exchangeable cations (Ca2+, Mg2+, Na+, K+) and effective cation exchange capacity were determined after pre-washing with 60 % ethanol and then leaching with non-alcoholic 1 M NH4Cl solution at pH 7 (Tucker, 1985). The exchangeable sodium percentage was calculated as exchangeable Na concentration divided by the effective cation exchange capacity. Total organic C and total N analyses were conducted using a LECO TruMac instrument using the Dumas method (Nelson and Sommers, 1996). The electrochemical stability index was used as an indication of the potential for soil dispersion. The threshold electrochemical stability index below which structural breakdown occurs is 0.05 (McKenzie, 1998). The dispersion index (DI), another measure of soil structural stability, is defined as the amount of dispersed silt + clay expressed as a percentage of the total silt + clay of the soil (see Sect. 2.3) (Mustafa and Letey, 1969). Soil dispersion assessed by aggregate stability in water (ASWAT) is described in detail in Sect. 2.3.
2.2 Collection of different organic materials used as amendments
Four different organic amendments were used for this study, viz. feedlot manure (FLM), chicken manure (CM), lucerne pellets (LP), and polyacrylamide (PAM). The FLM and CM were collected from a cattle feedlot and chicken sheds, respectively, located at The University of Queensland (Gatton, Australia) before being air dried, whilst the LP was a commercial animal feed product. The four organic amendments were chosen for three reasons: (1) they were easily available and are used by farmers, (2) LP is used as green manure and studies have shown it is effective in ameliorating sodic soils, and (3) PAM is used in mining and construction to treat sodic dispersive soils. Furthermore, these amendments were different in terms of their chemical properties (Table 2) and C functional groups (Niaz et al., 2022) and may give a good contrast between the amendments. All organic amendments were ground and sieved through a 0.5 mm sieve in order to minimize the effect of different size particles and to create a homogenized sample. Anionic PAM Flobond L33 liquid (SNF Australia) had a charge density of 30 % and the molecular weight was 12–15 million Dalton (as per product specifications). Gypsum (CaSO4 ⋅ 2H2O) was laboratory-grade obtained from Sigma Aldrich.
A chemical analysis of the organic amendments was performed before mixing with the soils (Table 2). Total C and N analyses were performed using the LECO TruMac instrument with the Dumas method (Nelson and Sommers, 1996). The major cations, including Na+, Ca2+, Mg2+, and K+, were quantified by inductively coupled plasma-optical emission spectrometry (ICP-OES) after nitric acid microwave digestion (Kovács et al., 1996). The pH and EC of organic amendments were measured in (1:5) water suspensions after shaking for 1 h.
2.3 Incubation experiment
An incubation experiment was conducted using a complete randomized design. The treatments consisted of the five amendments (gypsum (G), PAM, FLM, CM, and LP) plus an unamended control. In addition, the FLM, CM, LP, and PAM were also applied in combination with G in order to check for synergistic effects between gypsum and organic amendments. This yielded a total of 10 treatments, each with three replicates, totaling 60 experimental units. Soil samples (300 g) were mixed with the respective organic amendments (Table 3) added at rates that were commercially feasible (10 Mg ha−1), except PAM which was added at 1 kg ha−1. The gypsum requirement of both soil samples was calculated based on the formula given by Oster and Jayawardane (1998) as follows:
where F is exchanged efficiency of Ca–Na and for this case considered equal to 1, D is the depth of soil to be reclaimed (cm), ∂b is soil bulk density (g cm−3), CEC is cation exchange capacity (cmol+ kg−1), ESPi is initial soil exchangeable sodium percentage, and ESPf is final or desired exchangeable sodium percentage. For simplicity, a single gypsum rate of 2.5 Mg ha−1 was selected for both soils
The experiment was performed with jars 12 cm in diameter and 15 cm in height, and the soil was packed to a height of 3–5 cm. The soil water content at field capacity (−10 kPa) was ∼ 0.30 g water g−1 soil. The WD cycles were imposed as follows. First deionized water was added to the soils (0.30 g g−1 for Soil 1 and 0.31 g g−1 for Soil 2) at 25 ∘C, and after 1 d, the lids were removed and soils were allowed to dry at 25 ∘C during which the soil water content decreased to air-dry conditions (∼ 0.1 g water g−1 soil). The dry-down was typically completed in 14 d. The WD regime was applied 4 times, with the total duration of the experiment being 60 d. Although the WD regime might not entirely represent the field conditions due to lack of plant growth, it is representative of the mean temperature and the field water content.
Soil solutions (∼ 3–5 mL) were extracted using polyacrylonitrile hollow fibre samplers (Menzies and Guppy, 2000) embedded in the jars containing the treated soils. Soil solution was collected 4 times at the start of each WD cycle after the water was added to soils. Soil solution pH, EC, NH-N, NO-N, dissolved organic carbon (DOC), Na, Ca, Mg, and K were measured as follows: NH-N by the phenate method (Rice et al., 2017), NO-N by cadmium reduction (Rice et al., 2017) using a segmented flow analyzer (SEAL AA3), DOC using a total organic carbon liquid analyzer (Shimadzu, Japan), and the cations using ICP-OES. The sodium adsorption ratio (SAR) was calculated from the soil solution concentrations of Na+, Ca2+, and Mg2+.
Soil dispersion was determined using the ASWAT test (Field et al., 1997) and DI (Mustafa and Letey, 1969). Air-dried aggregates from each sample after completion of each cycle were placed in a Petri dish filled with deionized water. A visual assessment of aggregate dispersion was made after 10 min and 2 h, assessing dispersion on a scale of 0–4. The scores were as follows: 0 – no dispersion, 1 – slight dispersion (slight milkiness adjacent to aggregates in water), 2 – moderate dispersion (obvious milkiness), 3 – strong dispersion (considerable milkiness with about half of the material dispersed in water), and 4 – complete dispersion (leaving sand particles in a cloud of dispersed clay). Dispersion scores that were determined after 10 min and 2 h were added together and then added to 8, thus giving a range of values from 9–16. Aggregates which were not dispersed after 2 h were remolded, submerged in water, and dispersion reassessed again after 10 min and 2 h. Scores were given from 0–4 for both times and added (giving values from 0–8). Thus, aggregates were considered stable if they had a low ASWAT score, and the higher the ASWAT score, the more unstable the aggregate.
For the measurement of DI, 15 g of air-dry soil (<2 mm) was placed in a sedimentation cylinder with an approximate volume of 1.2 L. Deionized water was added to the cylinder to bring the volume to 1 L. The suspension was then shaken for 30 min on an end-over-end shaker. The suspension was then stirred with 10 strokes of a plunger. The temperature was noted, the suspension was allowed to settle, and after an appropriate time (8 h) the percentage of clay was measured with a hydrometer. The measurements were done after the first, second, and fourth WD cycles. Results were expressed as DI (Mustafa and Letey, 1969) as follows:
Aggregate size distribution was also determined to quantify changes in large and small aggregates with WD cycles. Air-dried soil samples (25 g) were broken gently to pass through a 10 mm sieve and then slowly wetted up in water for 5 min (Hernandez et al., 2017). They were then wet-sieved for 5 min at 33 oscillations min−1 (Cook et al., 1992) using the following set of sieves: 2000, 250, and 53 µm (Kemper and Rosenau, 1986). Three replications were made of each sample. The water level was adjusted so that the aggregates on the upper sieve were submerged in water at the highest point of the oscillation. The material retained on each sieve and the fraction passing through after wet sieving was collected, dried at 40 ∘C, and weighed (Kemper and Rosenau, 1986). Measurements were made after the first, second, and fourth WD cycles. The >2000 µm aggregates hereafter are referred to as large macroaggregates, 2000–250 µm as small macroaggregates, 250–53 µm as microaggregates (MIC), and <53 µm as silt + clay fraction. The MWD was calculated from the aggregate fractions obtained after wet sieving on each sieve size as follows:
where xi is the mean diameter of each fraction and wi is the proportion of the total sample weight in the corresponding size fraction (Kemper and Rosenau, 1986).
2.4 Soil microbial respiration
The biolability (susceptibility to microbial decomposition) of the organic amendments was determined by measuring CO2 release over a 60 d incubation period comprising four WD cycles. Briefly, 50 g of each soil sample was added to 250 mL jars with the relevant amendments (Table 3) and covered with lids having two 5 mm holes. All the treatments were replicated 3 times and randomized. The soils were wetted to field capacity on the first day of incubation using deionized water and incubated at 25 ∘C. After the first day of soil incubation, the jars were left open to allow the soil to dry at 25 ∘C. For the microbial respiration measurements, rubber tubes with Luer locks were inserted into the holes and connected to a CO2 analyzer (WMA-4 CO2 analyzer, John Morris USA). The lids were kept closed for 1 h prior to the measurement being taken. The readings were then converted in g C-CO2 kg−1 of soil d−1. Cumulative CO2 produced was calculated as the sum of the daily rate and the interval days between the two measurements (by linear extrapolation) for the incubation period. The measurements were taken daily for the first 7 d. Thereafter, there was no significant change in microbial respiration, so the measurements were performed after 10 and 15 d of each WD cycle.
2.5 Statistical analysis
Statistical analyses were performed using R x64 3.3.3 statistical software (R Core Team, 2020). Histograms were plotted to check the normality of each parameter separately, and it was found that the transformation of data was not necessary. Soil microbial respiration was recorded on a daily basis; hence, respiration data were subjected to analysis of variance with days as the repeated measures (mixed effect model). All the remaining parameters including EC, pH, NH-N and NO-N, SAR, ASWAT, DI, aggregate size distribution, and MWD were measured after each WD cycle. Hence, these data were analyzed using a two-way analysis of variance (ANOVA), taking treatments and WD cycles as two factors. The ANOVA for each soil was conducted separately using a general linear model. Tukey's honest significant difference was used for pairwise comparisons between treatment means. After checking the significance of data, mean data were graphed using Sigma Plot v14.0 (Systat Software Inc., 2017). Principal component analyses (PCAs) were performed to identify the roles of microbial, chemical and physical properties after the addition of different treatments in each WD cycle.
3.1 Soil aggregation dynamics measured by aggregate size distribution and mean weight diameter
A significant (p<0.001) interaction between WD cycles and amendments was found for all the aggregate sizes in both soils except for the proportion of large macroaggregates and silt + clay fraction in Soil 2 (Fig. 1), although the main effects of treatments and WD cycles were highly significant (p<0.001) in Soil 2. The finding of a significant interaction between amendments and WD cycles indicates that although aggregate size distribution changed with the WD cycles, the pattern of change depended on the amendment. Overall, the proportion of large macroaggregates decreased after the first WD cycle and increased by the end of the fourth WD cycle. Among the various amendments, the LP and G + LP treatments had the highest proportion of large macroaggregates, whereas PAM, FLM, and CM had little or no effect on large macroaggregates for either soil. We also observed changes in the small macroaggregate fraction, with this markedly decreasing by the second WD cycle but greatly increasing by the fourth WD cycle. In contrast to the changes in small macroaggregates, the proportion of microaggregates increased considerably (ca. 2-fold) by the second WD cycle and greatly decreased by the fourth WD cycle in both soils. The silt + clay fraction slightly increased by the end of second WD cycle and again decreased slightly by the fourth WD cycle in both soils.
The MWD of both soils was calculated from the aggregate size distribution. The main effects of amendments and WD cycles were found to be highly significant (p<0.001) in both soils; however, the interaction between amendments and WD cycles was significant only for Soil 1. It was observed that MWD decreased after the second WD cycle (Fig. 2) and increased after the fourth WD cycle, irrespective of amendments or soil, reflecting the changes in actual aggregate sizes (see Fig. 1). Expressing changes in MWD relative to the control or gypsum-treated soil showed that LP increased the MWD in both soils over the four WD cycles (Fig. S1), with PAM being less effective than LP, whereas the other amendments had no significant effect.
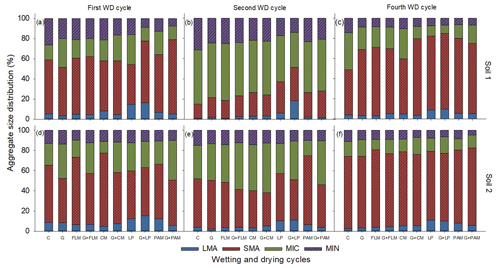
Figure 1Distribution of the aggregate sizes in the two soils after addition of amendments after first, second, and fourth WD cycles. First WD cycle (a: Soil 1 and d: Soil 2), second WD cycle (b: Soil 1 and e: Soil 2), and fourth WD cycle (c: Soil 1 and f: Soil 2). C: control, G: gypsum, PAM: anionic polyacrylamide, FLM: feedlot manure, CM: chicken manure, and LP: lucerne pellets. Large macroaggregates (>2000 µm), small macroaggregates (2000–250 µm), microaggregates (250–53 µm), and silt + clay fraction (<53 µm). Error bars have been omitted to improve readability.
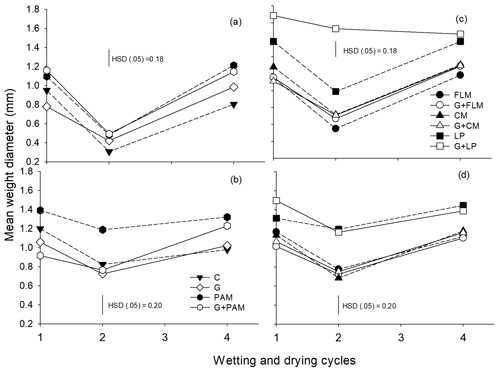
Figure 2The mean weight diameter (MWD) of the soils after addition of amendments during four wetting and drying (WD) cycles, Soil 1 (a, c) and Soil 2 (b, d). The treatments are C: control, G: gypsum, PAM: anionic polyacrylamide, FLM: feedlot manure, CM: chicken manure, and LP: lucerne pellets. Vertical bars represent Tukey's honest significant difference (HSD) values at p=0.05 for pairwise comparisons among cycles.
3.2 Soil dispersion dynamics as measured by ASWAT and DI
We assessed soil dispersion using both the ASWAT test (Fig. 3) and DI (see Supplement data, Fig. S2), with good agreement between the DI results and ASWAT scores (Fig. S3). Overall, we observed that soil dispersion decreased with WD cycles in both soils. Although Soil 1 was more dispersive than Soil 2, both soils showed a similar decrease in dispersion with WD cycles. The addition of gypsum significantly decreased dispersion in all WD cycles, and this effect of gypsum was greater than the effect of organic amendments in decreasing soil dispersion. PAM had only a short-term effect, with PAM decreasing dispersion for the first WD cycle but not thereafter. Of the organic amendments, only CM decreased the dispersion in both soils, but FLM and LP did not decrease dispersion in both soils in the first and second WD cycles. Given that both EC (Fig. S4) and SAR (Fig. S5) are known to affect soil dispersion, it was not surprising that the ASWAT scores were negatively correlated with EC (Fig. S6) and positively correlated with SAR (Fig. S7). However, the ASWAT scores became less affected by SAR after the second WD cycle, with this highlighting the importance of WD cycles on structure improvement.
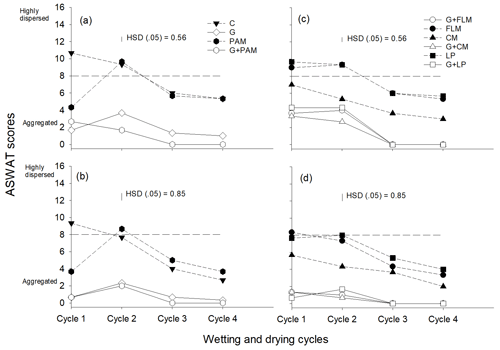
Figure 3The ASWAT scores of soils after the addition of amendments during four wetting and drying (WD) cycles, Soil 1 (a, c) and Soil 2 (b, d). The amendments are C: control, G: gypsum, PAM: anionic polyacrylamide, G + PAM: gypsum + anionic polyacrylamide, FLM: feedlot manure, G + FLM: gypsum + feedlot manure, CM: chicken manure, G + CM: gypsum + chicken manure, LP: lucerne pellets, and G + LP: gypsum + lucerne pellets. Vertical bars represent Tukey's honest significant difference (HSD) values at p=0.05 for pairwise comparisons among cycles.
3.3 Soil microbial respiration
Soil WD cycles impose a significant stress on the soil microbial community. The addition of treatments resulted in a significant increase (p<0.001) in soil microbial respiration for both the soils (Fig. 4). Rewetting of the dried soils caused a transient increase in microbial respiration (Fig. 4), with respiration being highest during the first WD cycle and decreasing during subsequent WD cycles. The lowest microbial respiration rates were observed in the fourth WD cycle in both soils (Fig. 4). The highest microbial respiration rates were observed in soils amended with LP or CM in the first WD cycle, whereas FLM had little effect on microbial respiration, whilst PAM and gypsum had no effect on microbial respiration.
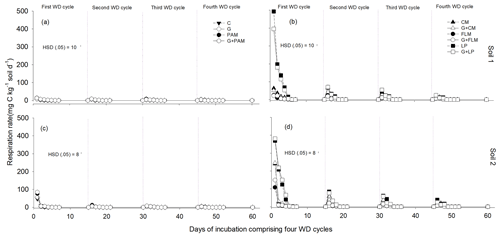
Figure 4Changes in soil respiration rate after addition of amendments during four alternate WD cycles, Soil 1 (a, b) and Soil 2 (c, d). The treatments were C: control, G: gypsum, PAM: anionic polyacrylamide, G + PAM: gypsum + anionic polyacrylamide, FLM: feedlot manure, G + FLM: gypsum + feedlot manure, CM: chicken manure, G + CM: gypsum+chicken manure, LP: lucerne pallets, and G + LP: gypsum + lucerne pallets. Vertical bars represent Tukey's honest significant difference (HSD) values at p=0.05 for pairwise comparisons among the four WD cycles. The vertical dotted lines show the days when deionized water was added to start the next WD cycle.
3.4 Relationship between soil physical, chemical, and microbial properties
Soil properties determined for both soils after the first, second, and fourth WD cycles (Fig. 5) were used to examine inter-relationships by PCA. Overall, the PCA biplots indicated that soil microbial respiration, MWD, and the proportion of large macroaggregates were positively correlated with each other. A positive correlation between ASWAT and DI with SAR was observed in both soils, whereas EC had a negative correlation with ASWAT and DI. Surprisingly, there was not a strong correlation between silt + clay and DI or ASWAT scores. The effect of SAR and EC on soil dispersion decreased after the second WD cycle (Fig. 5), underlining the importance of WD cycles on structure formation and stability, which offsets the detrimental effects of SAR on stability. Obvious treatment effects on different soil properties could also be seen in each WD cycle (Fig. 5). The G + LP and LP treatments were positively correlated with microbial respiration, the proportion of large macroaggregates, and MWD in all the cycles. The gypsum added with and without organic amendments produced the highest EC in all the cycles. The control soils had highest SAR, ASWAT scores, and DI followed by FLM and LP. It was also observed that the proportion of large macroaggregates and the MWD were negatively correlated with the silt + clay fraction in each WD cycle.
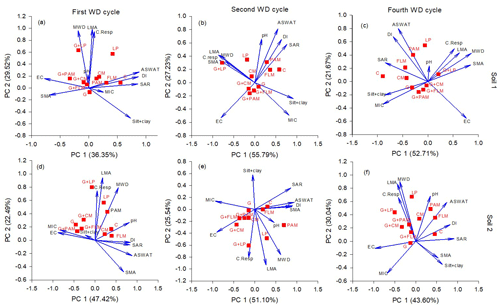
Figure 5Principal component analysis (PCA) biplot of the physico-chemical and microbial properties effected by different treatments in two soils after first, second, and fourth WD cycle: Soil 1 (a, b, c) and Soil 2 (d, e, f). The treatments are C: control, G: gypsum, PAM: anionic polyacrylamide, FLM: feedlot manure, CM: chicken manure, and LP: lucerne pellets. The data presented in these PCA biplots are the mean data of all the days for each parameter (blue arrows) and factorial scores of all the treatments (red squares). The parameters are ASWAT scores (aggregate stability in water test), DI (dispersion index), C. Resp (cumulative microbial respiration), SAR (sodium adsorption ratio), EC (electrical conductivity 1:5), LMA (large macroaggregates), SMA (small macroaggregates), MIC (microaggregates), silt + clay, and MWD (mean weight diameter).
The results of this experiment showed clear differences in the role of organic amendments, gypsum (Ca), and WD cycles on the formation and stabilization of soil aggregates. We showed that the formation and stability of large macroaggregates was controlled by the type of organic amendments, whereas the WD cycles increased the formation and stability of small macroaggregates. Formation of microaggregates is beneficial in that it decreases dispersion, but microaggregates may not be sufficient to improve soil water infiltration (Collis-George and Greene, 1979; Nemati et al., 2002). Regardless, even a small increase either in large macroaggregates and small macroaggregates may increase infiltration, with this facilitating the leaching of excess Na. Here we discuss the importance of each factor (WD cycles, organic amendments, and Ca) individually.
4.1 The relative role of WD cycles on aggregation
The WD cycles result in rearrangement of pores and soil particles and may lead to increased rigidity and stability of soil aggregates (Horn et al., 2014). We observed a marked change in aggregate size distribution with repeated WD cycles (Fig. 1), from macroaggregates (large macroaggregates and small macroaggregates) at the completion of the first WD cycle, to microaggregates at the completion of the second WD cycle, and back to macroaggregates (large macroaggregates and small macroaggregates) at the completion of the fourth WD cycle. We suggest that extracellular polysaccharides formed by microbial activity (indicated by soil microbial respiration, Fig. 3) are responsible for the formation of large macroaggregates at the completion of the first WD cycle. After the second WD cycle, the microbial activity greatly decreased (Fig. 3) and macroaggregates (large macroaggregates and small macroaggregates) were broken down into microaggregates and silt + clay. This allowed the soil particles to settle into tightly packed configurations, resulting in stronger interconnections upon WD cycles (Kemper and Rosenau, 1984). Macroaggregates were more susceptible to disintegration during wet sieving compared to microaggregates at the completion of second WD cycle. By the fourth WD cycle, some rearrangements of soil particles likely occurred, facilitated by soil drying, thereby rebuilding macroaggregates. The decrease in large macroaggregates (Fig. 1) at the completion of the first WD cycle is also consistent with the findings of Denef et al. (2001), Rahman et al. (2018), and Zhang et al. (2022). Macroaggregates are expected to be more susceptible to disintegration due to water content changes, because of the large number of planes of weakness and greater angular momentum (Kay, 1990; Denef et al., 2001; Zhang et al., 2022).
The changes observed in the proportion of small macroaggregates followed the same pattern as large macroaggregates, with an initial increase in the proportion of small macroaggregates when the soils were treated with organic amendments. In contrast to these results, no significant differences were observed when the same soils were treated with organic amendments under continuous wet conditions (Niaz et al., 2022). Also, the increase in proportion of larger aggregates (large macroaggregates and small macroaggregates) is 2 times greater when the same soils were exposed to WD cycles as compared to constant wet regime (Niaz et al., 2022). In this study, the proportion of small macroaggregates did not increase after the second WD cycle, but the proportion of microaggregates and silt + clay fraction increased, suggesting that upon repeated WD cycles, large macroaggregates break down into microaggregates and silt + clay fractions. These observations support the finding that macroaggregates are composed of microaggregates (Six et al., 2000a). In addition, we observed that the control soils (where no amendments were added) also showed an increase in the proportion of small macroaggregates with WD cycles. This highlights the importance of WD cycles and suggests that the formation of small macroaggregates is less dependent on the organic matter content, in agreement with the PCA biplots (Fig. 5). The significant increase in the proportion of small macroaggregates in control soils after the fourth WD cycle also indicates that there could be an increase in interparticle bond strength with aging or time (Utomo and Dexter, 1982; Dexter, 1988; Kong et al., 2005; Bravo-Garza et al., 2009).
4.2 The role of organic amendments on soil respiration and aggregation
The addition of organic amendments provided an energy and nutrient source for microorganisms and resulted in increased respiration rates, although we observed that the respiration rate decreased with repeated WD cycles (Fig. 4), presumably due to depletion of easily metabolizable organic compounds (Harrison-Kirk et al., 2013; Yu et al., 2014; Rahman et al., 2018; Fraser et al., 2016; Brangarí et al., 2022; Zhang et al., 2022). In contrast to LP, CM had a modest effect on microbial respiration, whereas FLM had no significant effect on microbial respiration as it was already decomposed. Rewetting of dry soil led to immediate flush of microbial respiration (Fig. 4), similar to the results of Brangarí et al. (2022). This could be attributed to the slaking of larger aggregates (Fig. 1), thereby exposing some occluded organic matter, or due to the utilization of substrates that become available upon rewetting (Wu and Brookes, 2005; Borken and Matzner, 2009; Yu et al., 2014; Zhang et al., 2022). These available (labile) substrates after rewetting include remnants of the added organic matter and microbial biomass (Wu and Brookes, 2005; Zhang et al., 2022). Over time, with successive WD cycles, it is likely that the labile organic C pool was either exhausted or became physically protected within aggregates, and hence became less accessible to microbial degradation (Zhang et al., 2022).
For the LP and G + LP treatments, where the increase in respiration rate was ∼ 4-fold greater in Soil 1 and ∼ 2-fold greater in Soil 2 than for any other treatment (Fig. 4), we observed that the soils also had a significant increase in the proportion of large macroaggregates (Fig. 1) and MWD (Fig. 2), suggesting that labile organic compounds are important for structure formation (also confirmed by PCA biplots, Fig. 5). The formation of large macroaggregates after incorporation of organic amendments, as observed here for LP, has also been reported by Denef et al. (2001), Bravo-Garza et al. (2009), and Rahman et al. (2018). Although the maximum respiration rate was observed during the first WD cycle for LP and G + LP, the proportion of large macroaggregates and MWD increased at the end of the fourth WD cycle. During later WD cycles, it is likely that the conversion of readily metabolizable organic compounds (e.g., microbial polymers) to more resistant forms resulted in the formation of macroaggregates (large macroaggregates and small macroaggregates), but in this case, aggregation may have been caused by more hydrophobic compounds. However, the proportion of large macroaggregates did not increase much as compared to the first WD cycle, likely because microbial activity was lower. One of the possible reasons of increased MWD could be the accumulation of microbial binding agents over time that are released continuously from microbial activity due to organic matter decomposition (Rahman et al., 2018).
When the soils were treated with organic amendments, a decrease in dispersion was observed but was not significantly different from control except in CM-treated soils. The possible reason for increased stability in CM-treated soils was its higher Ca content (Table 2), increased EC (Fig. S4), and decreased SAR (Fig. S5). For the remaining organic amendments, viz. FLM, LP, and PAM, an improvement in aggregate stability was observed after the second WD cycle. Although LP had the highest MWD, it still did not reduce dispersion in the first WD cycle. We suggest that high MWD in itself is a misleading measure of soil stability, since only a few large aggregates can result in a high MWD (Niaz et al., 2022). Some dispersion can occur in parallel, which is sensitively detected by the ASWAT test but not by the MWD. Both assays detect different physical processes; hence, a high MWD (Fig. 1) and dispersion (Fig. 2) are not mutually exclusive (Niaz et al., 2022). Organic amendments rich in aliphatic compounds or waxes can lead to hydrophobicity of aggregate surfaces during the drying step which slows down the wetting of aggregate surfaces, reducing the disruption caused during rewetting (Piccolo and Mbagwu, 1999; Borken and Matzner, 2009). Monnier (1965) proposed that fresh organic amendments can increase aggregate stability in the time frame of weeks and months as compared to already decomposed stable organic amendments. But in our study we found no significant differences in dispersion after the addition of LP (fresh) and FLM (partially decomposed). These findings suggest that there might be some other mechanisms which are responsible for the binding of organic matter with clay particles to control dispersion, such as the size of the organic matter molecule and charge density. This requires further investigation.
4.3 The relative role of Ca (gypsum) in improving aggregate stability
The results of this experiment showed that addition of gypsum (Ca) significantly reduced soil dispersion and increased aggregate stability. This improvement in aggregate stability is because of the increased EC (ionic strength, Fig. S4) and decreased SAR (Fig. S5) after the addition of gypsum. The increased EC likely resulted in the flocculation of soil particles by reducing the diffuse double layer (van Olphen, 1977; Ghosh et al., 2010; Bennett et al., 2015). Improved stability was also observed when organic amendments were applied with gypsum, especially in G + PAM-, G + LP-, and G + FLM-treated soils. The PAM had an initial positive effect but led to decreased stability at completion of the second WD cycle. Although the addition of gypsum increased aggregate stability, it was observed that the addition of gypsum did not affect the proportion of large macroaggregates and MWD. However, when organic amendments were added with gypsum, an improvement in proportion of large macroaggregates and MWD was observed in G + LP-treated soils. This can be explained as a Ca-bridging effect through which clay particles are attached to organic matter and polyvalent cations, resulting in the formation of macro- and microaggregates (Wuddivira and Camps-Roach, 2007).
The stability of dispersive sodic Vertisols was improved by the application of organic amendments and gypsum, which was further enhanced by WD cycles. Gypsum reduced soil dispersion but did not affect the proportion of large macroaggregates and MWD. We observed that not all organic amendments were equally beneficial in improving soil aggregation and aggregate stability. LP significantly increased the proportion of large macroaggregates compared to FLM and PAM. In contrast, CM significantly reduced soil dispersion, as it had higher calcium content. It was also found that PAM only had a transient effect in controlling dispersion. In the absence of organic amendments, repeated WD cycles reduced the dispersion of sodic soils, but when organic amendments were added (with or without gypsum), soil aggregation and soil stability was improved even more. It is likely that soil microbial activity contributed to the aggregate formation. Implementation of these findings in the field would favor the use of organic amendments with gypsum to improve the physico-chemical properties of sodic soils, which is further enhanced by WD cycles. The aim should be initially to prevent soil dispersion which can be achieved by the application of Ca (through the application of gypsum), and then to build larger aggregates which can be achieved by the application of organic amendments.
The data used in this paper are available on Zenodo at https://doi.org/10.5281/zenodo.7646256 (Niaz and Wehr, 2023).
The supplement related to this article is available online at: https://doi.org/10.5194/soil-9-141-2023-supplement.
Conceptualization: SN, JBW, PMK, RCD, and NWM. Formal analysis, investigation, and writing original draft: SN. Review and editing: JBW, PMK, RCD, and NWM. Supervision: JBW and NWM.
The contact author has declared that none of the authors has any competing interests.
Publisher’s note: Copernicus Publications remains neutral with regard to jurisdictional claims in published maps and institutional affiliations.
This research did not receive any specific grants from funding agencies in the public, commercial, or not-for-profit sectors. We thank David Appleton and Stephen Appleton (analytical services, UQ) for the soil solution analyses. We express our gratitude to Brigid McKenna (UQ) for general help with the laboratory work.
This paper was edited by Fuensanta García-Orenes and reviewed by Manfred Sager and five anonymous referees.
Abiven, S., Menasseri, S., and Chenu, C.: The effects of organic inputs over time on soil aggregate stability – A literature analysis, Soil Biol. Biochem., 41, 1–12, 2009.
Bennett, J. M., Cattle, S., Singh, B., and Quilty, J.: Influence of gypsum enhanced chicken-manure-and-wheat-straw compost on amelioration of an irrigated sodic brown vertisol–laboratory experiment, Arid Land Res. Manag., 29, 415–431, 2015.
Borken, W. and Matzner, E. J.: Reappraisal of drying and wetting effects on C and N mineralization and fluxes in soils, Glob. Change Biol., 15, 808–824, 2009.
Bossuyt, H., Denef, K., Six, J., Frey, S., Merckx, R., Paustian, K.: Influence of microbial populations and residue quality on aggregate stability, Appl. Soil Ecol., 16, 195–208, https://doi.org/10.1016/S0929-1393(00)00116-5, 2001.
Brangarí, A. C., Lyonnard, B., and Rousk, J.: Soil depth and tillage can characterize the soil microbial responses to drying-rewetting, Soil Biol. Biochem., 173, 108806, https://doi.org/10.1016/j.soilbio.2022.108806, 2022.
Bravo-Garza, M. R., Bryan, R. B., and Voroney, P.: Influence of wetting and drying cycles and maize residue addition on the formation of water stable aggregates in Vertisols, Geoderma, 151, 150–156, 2009.
Cassel, D. and Nielsen, D.: Methods of Soil Analysis, in: Part 1 – Physical and Mineralogical Methods, edited by: Klute, A., 2nd Edn., American Society of Agronomy/Soil Science Society of America, Madison, https://doi.org/10.2136/sssabookser5.1.2ed.c36, USA, 1986.
Collis-George, N. and Greene, R.: The effect of aggregate size on the infiltration behaviour of a slaking soil and its relevance to ponded irrigation, Aus. J. Soil Res., 17, 65–73, 1979.
Cook, G. D., So, H. B., and Dalal, R. C.: Structural degradation of two Vertisols under continuous cultivation, Soil Till. Res., 24, 47–64, 1992.
Cosentino, D., Chenu, C., and Le Bissonnais, Y.: Aggregate stability and microbial community dynamics under drying-wetting cycles in a silt loam soil, Soil Bio. Biochem., 68, 2053–2062, 2006.
Curtin, D. and Naidu, R.: Fertility constraints to plant production, in: Sodic soil: distribution, management and environmental consequences, Oxford University Press, New York, USA, 107–123, ISBN 019509655X, 1998.
Day, P. R.: Particle fractionation and particle-size analysis, in: Methods of Soil Analysis, edited by: Black, C. A., Part 1. Physical and Minerological Methods, American Society of Agronomy, Madison, W, USA, 545–567, https://doi.org/10.2134/agronmonogr9.1.c43, 1965.
Denef, K., Six, J., Bossuyt, H., Frey, S. D., Elliott, E. T., Merckx, R., and Paustian, K.: Influence of dry–wet cycles on the interrelationship between aggregate, particulate organic matter, and microbial community dynamics, Soil Biol. Biochem., 33, 1599–1611, 2001.
Dexter, A.: Advances in characterization of soil structure, Soil Till. Res., 11, 199–238, 1988.
Field, D. J., McKenzie, D. C., and Koppi, A. J. J.: Development of an improved Vertisol stability test for SOILpak, Aus. J. Soil Res., 35, 843–852, 1997.
Fraser, F., Corstanje, R., Deeks, L., Harris, J. A., Pawlett, M., Todman, L. C., Whitmore, A., and Ritz, K.: On the origin of carbon dioxide released from rewetted soils, Soil Biol. Biochem., 101, 1–5, 2016.
Ghosh, S., Lockwood, P., Hulugalle, N., Daniel, H., Kristiansen, P., and Dodd, K.: Changes in properties of sodic Australian Vertisols with application of organic waste products, Soil Sci. Soc. Am. J., 74, 153–160, 2010..
Harrison-Kirk, T., Beare, M. H., Meenken, E. D., and Condron, L. M.: Soil organic matter and texture affect responses to dry/wet cycles: Effects on carbon dioxide and nitrous oxide emissions, Soil Bio. Biochem., 57, 43–55, 2013.
Hernandez, T., Hernandez, M. C., and Garcia, C.: The effects on soil aggregation and carbon fixation of different organic amendments for restoring degraded soil in semiarid areas, Eur. J. Soil Sci., 68, 941–950, 2017.
Horn, R., Peng, X., Fleige, H., and Dörner, J.: Pore rigidity in structured soils-only a theoretical boundary condition for hydraulic properties?, Soil Sci. Plant Nutr., 60, 3–14, 2014.
ISO (International Organization for Standardization): ISO 11265:1994 Soil Quality – Determination of the specific electrical conductivity, ISO: Geneva, https://www.iso.org/standard/19243.html (last access: 15 February 2021), 1994.
ISO (International Organization for Standardization): ISO 10390:2005 Soil Quality – Determination of pH, ISO: Geneva, https://www.iso.org/standard/40879.html (last access: 15 February 2021), 2005.
Kay, B. D.: Rates of change of soil structure under different cropping systems, in: Advances in soil science, Springer Publishing, New York, 12, 1–52, ISBN 9781461279648, 1990.
Kemper, W. and Rosenau, R.: Aggregate stability and size distribution, in: Methods of Soil Analysis: Part 1. Physical and Mineralogical Methods, 2nd Edn., American Society of Agronomy/Soil Science Society of America, Madison, WI, https://doi.org/10.2136/sssabookser5.1.2ed.c17, 1986.
Kemper, W. and Rosenau, R.: Soil cohesion as affected by time and water content, Soil Sci. Soc. Am. J., 48, 1001–1006, 1984.
Kong, A. Y., Six, J., Bryant, D. C., Denison, R. F., and Van Kessel, C.: The relationship between carbon input, aggregation, and soil organic carbon stabilization in sustainable cropping systems, Soil Sci. Soc. Am. J., 69, 1078–1085, 2005.
Kovács, B., Gyori, Z., Prokisch, J., Loch, J., and Daniel, P.: A study of plant sample prepration and inductively coupled plasma emission spectrometry parameters, Commun. Soil Sci. Plan., 27, 1177–1198, 1996.
McKenzie, D. C.: SOILpak for cotton growers, third edition, Orange, New South Wales Agriculture, https://nla.gov.au/nla.cat-vn2076648, 1998.
Menzies, N. and Guppy, C.: In-situ soil solution extraction with polyacrylonitrile hollow-fibers, Commun. Soil Sci. Plan., 31, 1875–1886, 2000.
Monnier, G.: Action des matieres organiques sur la stabilite structurale du sol, Sols Africains, 1, 5–42, 1965.
Mustafa, M. A. and Letey, J.: The effect of two nonionic surfactants on aggregate stability of soils, Soil Sci., 107, 343–347, 1969.
Nelson, P. N. and Oades, J. M.: Organic matter, sodicity, and soil structure, in: Sodic soils: Distribution, management and environmental consequences, Oxford University Press, New York, ISBN 019509655X, 1998.
Nelson, D. W. and Sommers, L. E.: Total carbon, organic carbon and organic matter, in: Methods of Soil Analysis, Part 3. Chemical Methods, edited by: Sparks, D. L., Page, A. L., Helmke, P. A., Loeppert, R. H., Soltanpour, P. N., Tabatabai, M. A., and Johnston, C. T.: ME Sumner, Soil Science Society of America/American Society of Agronomy: Madison, WI, 961–1010, https://doi.org/10.2136/sssabookser5.3.c34, 1996.
Nemati, M., Caron, J., and Gallichand, J.: Predicting hydraulic conductivity changes from aggregate mean weight diameter, Water Resour. Res., 38, 9–1, 2002.
Niaz, S. and Wehr, B.: Wetting and drying cycles, organic amendments and gypsum play a key role in structure formation and stability of sodic Vertisols, Zenodo [data set], https://doi.org/10.5281/zenodo.7646256, last access: 16 February 2023.
Niaz, S., Wehr, J. B., Dalal, R. C., Kopittke, P. M., and Menzies, N. W.: Organic amendments and gypsum reduce dispersion and increase aggregation of two sodic Vertisols, Geoderma, 425, 116047, https://doi.org/10.1016/j.geoderma.2022.116047, 2022.
Oades, J. M.: Soil organic matter and structural stability: mechanisms and implications for management, Plant Soil, 76, 319–337, 1984.
Oades, J. M.: The role of biology in the formation, stabilization and degradation of soil structure, in: Soil structure/soil biota interrelationships, Elsevier, 377–400, ISBN 978-0-444-81490-6, 1993.
Oster, J. D. and Jayawardane, N.: Agricultural management of sodic soils, in: Sodic soil: Distribution, properties, management and environmental consequences, edited by: Sumner, M. E. and Naidu, R., Oxford University Press, New York, 126–147, ISBN 019509655X, 1998.
Pal, D., Wani, S., and Sahrawat, K.: Vertisols of tropical Indian environments: pedology and edaphology, Geoderma, 189, 28–49, https://doi.org/10.1016/j.geoderma.2012.04.021, 2012.
Peng, X., Hallett, P. D., Zhang, B., and Horn, R.: Physical response of rigid and non-rigid soils to analogues of biological exudates, Eur. J. Soil Sci., 62, 676–684, 2011.
Perfect, E., Kay, B. D., Van Loon, W. K. P., Sheard, R. W., and Pojasok, T.: Factors influencing soil structural stability within a growing season, Soil Sci. Soc. Am. J., 54, 173–179, 1999.
Piccolo, A. and Mbagwu, J. S.: Role of hydrophobic components of soil organic matter in soil aggregate stability, Soil Sci. Soc. Am. J., 63, 1801–1810, 1999.
R Core Team: R: A language and environment for statistical computing, R Foundation for Statistical Computing, Vienna, Austria, https://www.R-project.org/, last access: 12 February 2020.
Rahman, M. T, Zhu, Q., Zhang, Z. B., Zhou, H., and Peng, X. H.: The roles of organic amendments and microbial community in the improvement of soil structure of a Vertisol, Appl. Soil Ecol., 111, 84–93, 2017.
Rahman, M. T., Guo, Z. C., Zhang, Z. B., Zhou, H., and Peng, X. H.: Wetting and drying cycles improving aggregation and associated C stabilization differently after straw or biochar incorporated into a Vertisol, Soil Till. Res., 100, 28–36, 2018.
Rengasamy, P. and Olsson, K. A.: Sodicity and soil structure, Aus. J. Soil Res., 29, 935–952, 1991.
Rice, E. W., Baird, R. B., and Eaton, A. D.: Standard Methods of the Examination of Water and Wastewater, 23rd Edition. American Public Health Association, American Water Works Association, Water Environment Federation, Denver, CO, ISBN 1375770535, 2017.
SigmaPlot Version 12.3: Systat Software, Inc., San Jose, California, 2017.
Six, J., Bossuyt, H., Degryze, S., and Denef, K.: A history of research on the link between (micro) aggregates, soil biota, and soil organic matter dynamics, Soil Till. Res., 79, 7–31, 2004.
Six, J., Elliott, E. T., and Paustian, K.: Soil macroaggregate turnover and microaggregate formation: a mechanism for C sequestration under no-tillage agriculture, Soil Biol. Biochem., 32, 2099–2103, 2000a.
Six, J., Elliott, E. T., and Paustian, K.: Soil structure and soil organic matter, II. A normalized stability index and the effect of mineralogy, Soil Sci. Soc. Am. J., 64, 1042–1049, 2000b.
Tisdall, J. M. and Oades, J. M.: Organic matter and water-stable aggregates in soils, J. Soil Sci., 33, 141–163, 1982.
Tucker, B. M.: Active and exchangeable cations in soils, Aus. J. Soil Res., 23, 195–209, 1985.
Utomo, W. and Dexter, A. J.: Changes in soil aggregate water stability induced by wetting and drying cycles in non-saturated soil, J. Soil Sci., 33, 623–637, 1982.
van Olphen, H.: An Introduction to Clay Colloid Chemistry, Interscience Publishers, New York, ISBN 0894645544, 1997.
WRB: World reference base for soil resources 2014, update 2015, World Soil no. 106, FAO, Rome, 2014.
Wu, J. and Brookes, P. C.: The proportional mineralisation of microbial biomass and organic matter caused by air-drying and rewetting of a grassland soil, Soil Bio. Biochem., 37, 507–515, 2005.
Wuddivira, M. and Camps-Roach, G.: Effects of organic matter and calcium on soil structural stability, Eur J. of Soil Sci., 58, 722–727, https://doi.org/10.1111/j.1365-2389.2006.00861.x, 2007.
Xiang, S.-R., Doyle, A., Holden, P. A., and Schimel, J. P.: Drying and rewetting effects on C and N mineralization and microbial activity in surface and subsurface California grassland soils, Soil Bio. Biochem., 40, 2281–2289, 2008.
Yu, Z., Wang, G., and Marschner, P.: Drying and rewetting–effect of frequency of cycles and length of moist period on soil respiration and microbial biomass, Eur. J. Soil Biol., 62, 132–137, 2014
Zhang, Z., Wang, D., and Li, M.: Soil respiration, aggregate stability and nutrient availability affected by drying duration and drying-rewetting frequency, Geoderma, 413, 115743, https://doi.org/10.1016/j.geoderma.2022.115743, 2022.