the Creative Commons Attribution 4.0 License.
the Creative Commons Attribution 4.0 License.
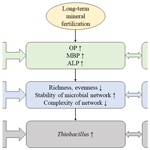
The role of long-term mineral and manure fertilization on P species accumulation and phosphate-solubilizing microorganisms in paddy red soils
Shuiqing Chen
Jusheng Gao
Huaihai Chen
Zeyuan Zhang
Jing Huang
Lefu Lv
Jinfang Tan
Xiaoqian Jiang
Understanding soil phosphorus (P) transformation and turnover under various fertilization managements is important for evaluating sustainable P fertility and potential bioavailability in agriculture managements. Thus, long-term fertilization experiments (∼ 38 years) with the application of different inorganic and organic fertilizers in paddy red soils were conducted to determine the effect of different fertilizer applications on P pool accumulation and microbial communities, especially for phosphate-solubilizing microorganisms (PSMs). Long-term inorganic P (IP) fertilization increased the concentrations of total P (TP) (∼ 479 mg kg−1), available P (AP) (∼ 417 mg kg−1) and inorganic P (∼ 18 mg kg−1), but manure fertilization accelerated the accumulation of organic P, especially for orthophosphate monoesters (e.g., myo-IHP, ∼ 12 mg kg−1). Long-term mineral fertilization decreased bacterial richness, evenness and complexation of bacterial networks. In contrast, long-term manure fertilization and rhizosphere accumulated more amounts of total carbon, total nitrogen, and organic carbon, as well as regulated the soil pH, thus improving the separation of bacterial communities. Furthermore, PSM compositions were greatly influenced by fertilization managements and rhizosphere. For example, inorganic P fertilization increased the abundance of Thiobacillus (i.e., the most abundant phosphate-solubilizing bacteria (PSB) in this study) and shifted the community structure of PSB. Correspondingly, the concentrations of inorganic and total P were the key factors for the variation of the PSB community structure. These findings are beneficial for understanding the variation of inorganic and organic P pools and the microbial community, especially for PSMs under long-term inorganic and/or organic fertilization.
- Article
(4176 KB) - Full-text XML
-
Supplement
(623 KB) - BibTeX
- EndNote
Phosphorus (P) as an essential nutrient for crop growth has been widely applied to soil through mineral and/or organic fertilization (Grant et al., 2005). Manures have been frequently used as organic fertilizers in agriculture production (Braos et al., 2020). The P from manures exists in forms of various inorganic and organic species, whereas mineral fertilizers usually only contain highly soluble Ca(H2PO4)2 (Sharpley and Moyer, 2000). Fertilization managements are important factors for P species transformation and bioavailability. For example, mineral fertilization results in an initial high P availability but follows a decrease of P concentration over time by adsorption, complexation and precipitation with soil particles. On the other hand, the application of manure usually leads to an accumulation in labile organic P pools with potential supply to plants (Schneider et al., 2016). Additionally, the application of mineral fertilizer and manure brought different changes in the physical, chemical and biological attributes of soil, such as soil pH, organic carbon, microbial communities and so on, which also induce different P transformation processes and potential availability (Yue et al., 2016; Tao et al., 2021).
Soil microorganisms are usually involved in a wide range of biological processes including the transformation of insoluble soil nutrients (Babalola and Glick, 2012). After long-term fertilization, insoluble or soluble organic matter in soil may increase, thus leading to the increases of microbial biomass and activity (Marschner et al., 2003). Among them, phosphate-solubilizing microorganisms (PSMs) could solubilize insoluble inorganic P (IP), mineralize organic P, and play an important role in P transformation and availability (Sharma et al., 2013). The response of PSMs in soil is strongly related to the availability of P which is greatly different under various fertilization managements (Sánchez-Esteva et al., 2016; Gómez-Muñoz et al., 2018; Raymond et al., 2021).
Currently, the information about how long-term various inorganic and organic fertilization managements affect the evolution characteristics of different P pools remains scarce. Furthermore, the responses of microbial communities are still unclear, especially PSMs that shift in bulk and rhizosphere soils to the different P pool evolution under various fertilization managements, especially for PSMs. This information plays a pivotal role for understanding soil P transformation mechanisms and evaluating sustainable P fertility and potential bioavailability in agriculture managements. The accumulation, turnover and bioavailability of soil P pools under different fertilization managements could be well evaluated by long-term fertilization experiences. Currently, numerous long-term fertilization experiences have been established to evaluate the impact of different fertilizer amendments on crop production and simultaneously provide valuable information on soil fertility by investigating changes in soil process over time (Wen et al., 2019). Thus, in this study, long-term fertilization experiments (∼ 38 years) under inorganic fertilizer and/or manure amendments were conducted to determine their effects on P pool accumulation, soil microbial communities, and PSMs in paddy red soils. We hypothesized that (1) long-term input of inorganic fertilizers accumulates more inorganic P, but the manure application and rhizosphere may accelerate the accumulation of organic P and (2) the long-term manure fertilization and rhizosphere could accumulate more organic nutrients, thus driving the separation of bacterial communities compared to the mineral fertilizer application.
2.1 Field design and sampling
Long-term fertilization experiments were conducted since 1982 in a national farmland ecosystem observation and research station (26∘45′ N, 111∘52′ E), Qiyang, Hunan Province, China. The soil was classified as Ferralic Cambisol according to the World Reference Base for Soil Resources (WRB, 2014), and classified as red soil according to the Chinese soil classification (Baxter, 2007). Rice (Oryza sativa) is the major crop in this region. The early rice was transplanted at the end of April and harvested in July, and the late rice was transplanted at the end of July and harvested in October. All straw (except the rice stubble) was removed from the fields after each seasonal rice harvest (Zhang et al., 2017; Yang et al., 2012). The experimental field was disposed with five different fertilizer treatments: CK (control without fertilizer), NPK (mineral N, P and K fertilizers), M (cattle manure), NPKM and NKM (Qaswar et al., 2020; Gao et al., 2011). Mineral fertilizers were applied in the forms of urea for N, calcium superphosphate for P and potassium chloride for K with the amounts of 145 kg ha−1 of N, 49 kg ha−1 of P and 56 kg ha−1 of K, respectively. Additionally, the manure was added with the average nutrient contents including 18 000 kg ha−1 of C, 145 kg ha−1 of N, 49 kg ha−1 of P and 56 kg ha−1 of K. All the mineral fertilizers and manure were applied as basal application. The collection of bulk soil samples with five different fertilizer treatments were conducted before the harvest of late rice in October 2020 with field replications. In each field, three soil cores (0–20 cm topsoil) were collected and then pooled to form a composite sample. Besides, before the rhizosphere soil collection, the bulk soil was manually removed, and approximately 1 mm of soil on the rice roots was collected as rhizosphere soil (Shao et al., 2021). Soil samples used for physical and chemical analyses were four replications (2 field replication × 2 replication of each field, n=4) and those for DNA extraction were six replications (2 field replication × 3 replication of each field, n=6).
2.2 Soil physical and chemical properties
Soil pH was measured by pH meter in the mixed solution (the mass ratio of soil and water is 1 : 2.5). Soil moist content was measured by drying moist soil at 105∘ for 16 h until it became a constant mass. Total carbon (TC), organic carbon (OC), and total nitrogen (TN) were determined by the CHNS elemental analyzer (Vario EL Cube manufactured by Elementar, Germany) (Schumacher, 2002). The soil was pretreated by 1 M HCl with a soil–liquid ratio of 1 : 1 before OC determination; 1 g soil was extracted with 5mL KCl (2 M) to determine for ammonia-N (NH) by an indophenol blue colorimetric method (Dorich and Nelson, 1983) and for nitrate-N (NO) by dual-wavelength ultraviolet spectrophotometry (Norman et al., 1985). After potassium persulfate and H2SO4 predigestion (Bowman, 1989), soil samples were determined for total P (TP) by a colorimetric method (Murphy and Riley, 1962). The extraction of available phosphorus (AP) was referred to the method described by Olsen (1954), and the concentration was measured using a colorimetric method (Murphy and Riley, 1962).
The extracted P with 0.5 M NaHCO3 before and after 24 h of CHCl3 fumigation was determined using inductively coupled plasma optical emission spectroscopy (ICP–OES; PerkinElmer, Avio 500, USA). A kEC factor of 0.4 was used for the calculation of soil microbial biomass P. Soil microbial biomass P was measured using a chloroform fumigation–extraction technique (Brookes et al., 1982). Additionally, phosphatases could mediate soil P transformation and recycling. The alkaline phosphatase (ALP) in soil is released by bacteria, whereas acidic phosphatase (ACP) can be derived from plants, fungi and bacteria (Nannipieri et al., 2011; Acosta-Martínez and Ali Tabatabai, 2011). The activities of acid and alkaline phosphatases were indicators to reflect the microbial activity and P cycling ability in soil and were assayed by the method described by Tabatabai and Bremner (1969) using p-nitrophenyl phosphate as substrate at 37 ∘C.
2.3 Organic P analyses
Soil organic P was extracted with an NaOH–EDTA solution according to the method described by Jiang et al. (2017). In short, 4 g air-dried soil was extracted for 4 h using a 40 mL solution containing 0.25 M NaOH and 0.05 M Na2EDTA. After centrifuging at 13 000 g for 20 min, 2 mL aliquot of each supernatant was used to determine Fe, Mn and P by ICP–OES. The remaining supernatants were freeze-dried and prepared for solution 31P-NMR spectroscopy. Each freeze-dried extract (∼ 100 mg) was redissolved in 0.1 mL of deuterium oxide and 0.9 mL of a solution containing 1.0 M NaOH and 0.1 M Na2EDTA, then immediately determined with solution 31P-NMR spectra using a Bruker 500 MHz spectrometer. The NMR parameters were as follows: 28 K data points, 0.68 s acquisition time, 90∘ pulse width and 8000 scans. The repetition delay time was calculated based on the concentration ratio of P to (Fe + Mn) according to the research by McDowell et al. (2006). Peak areas were calculated by integration on spectra processed with 2 and 7 Hz line broadening using MestReNova software. Phosphorus species were identified based on their chemical shifts, including orthophosphate (6 ppm), pyrophosphate (∼ −5 ppm), polyphosphate (−4 to −5, −5 to −50 ppm), orthophosphate monoesters (3 to 6, 6 to 7 ppm), orthophosphate diesters (3 to −4 ppm), and phosphonates (7 to 50 ppm). The orthophosphate peak was standardized to 6 ppm during processing (Cade-Menun et al., 2010; Young et al., 2013). Individual P compounds were identified based on their chemical shifts from the study by Cade-Menun (2015) and by spiking selected samples with myo-Inositol hexakisphosphate (myo-IHP), α- and β-glycerophosphates (Figs. S1 and S2 in the Supplement).
The concentrations of individual P species were calculated by multiplying 31P-NMR proportions by the total NaOH–Na2EDTA extractable P concentration. The α- and β-glycerophosphates and mononucleotides were considered as degradation of orthophosphate diesters, though they were detected in the orthophosphate monoester region (Young et al., 2013; Liu et al., 2015).
2.4 Soil DNA extraction, PCR amplification, Illumina Miseq sequencing and bioinformatics analyses
The DNA was extracted from 0.25 g soil using the FastDNA® Spin Kit (MP Biomedicals, USA). The purity and concentration of DNA were measured by Nanodrop 2000 (Thermo Fisher Scientific, USA). For bacteria, the V3–V4 region of the 16S rRNA gene was amplified with the primer pair 338F (5′-ACTCCTACGGGAGGCAGCAG-3′) and 806R (5′-GGACTACHVGGGTWTCTAAT-3′) (Caporaso et al., 2012; Dennis et al., 2013). For fungi, the primer pair ITS1F (CTTGGTCATTTAGAGGAAGTAA) and ITS2 (GCTGCGTTCTTCATCGATGC) were used to target the ITS1 region (Blaalid et al., 2013). After sequencing, the raw sequences of each sample were assembled by QIIME 2 according to the unique bar code after removing the adaptors and primer sequences (Bolyen et al., 2019). Demultiplexed sequences were quality filtered, trimmed, denoised and merged, then the QIIME2 dada2 plugin was used to identify and remove chimeric sequences to obtain the feature table of the amplicon sequence variant (ASV; Callahan et al., 2016). The ASV sequences were aligned to the GREENGENES database and UNITE database separately to generate the taxonomy table for bacteria and fungi (Bokulich et al., 2018). Besides, phosphate-solubilizing microorganisms (PSMs) were collected according to the research by Rodríguez and Fraga (1999) as well as Alori et al. (2017) (see Table S1 in the Supplement). The raw reads of bacteria and fungi were deposited in the Sequence Read Archive (SRA) database of the National Center for Biotechnology Information (NCBI) under accession numbers PRJNA804681 and PRJNA805018, respectively.
2.5 Statistical analyses
All statistical analyses were conducted using SPSS 25.0. All indicators between different fertilizer treatments (i.e., CK, NPK, M, NPKM and NKM) were tested for significant differences (set to p<0.05) by one-way ANOVA. The least significant difference (LSD) method was used to test significant differences of all indicators between bulk and rhizosphere soils. Alpha (α) diversity indices, such as Chao1 richness estimator and Shannon diversity index, were calculated using the core-diversity plugin within QIIME2. Nonmetric multidimensional scaling (NMDS) based on Bray–Curtis distance was measured by R package “vegan” and visualized via R package “ggplot 2”. Co-occurrence network analysis was performed by using R package “psych” to calculate Spearman's rank correlations for taxa among six repetitions of each treatment group, and then Gephi 0.9.2 software was used to draw networks. Redundancy analysis (RDA) was performed by Monte Carlo analysis using Canoco 5 to reveal the association of microbial communities and soil environmental factors.
3.1 Soil physicochemical properties
In this study, we found that TC, TN and OC increased significantly after the long-term application of fertilizers, especially for manure fertilization (Table 1). The concentrations of microbial biomass P increased under long-term fertilization (Table 1). Additionally, the activities of acidic phosphatase (ACP) were higher than alkaline phosphatase (ALP) activities for all the treatments (Fig. 1h and i). On the other hand, soil pH value, gravimetric moisture, NO-N and NH-N contents were not significantly affected by the long-term fertilizer treatments (Table 1).
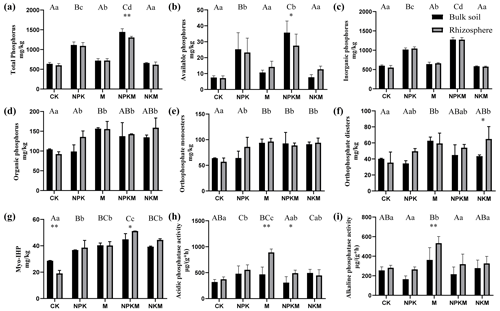
Figure 1Different phosphorus forms and phosphatase activities in five treatments (CK, NPK, M, NPKM, NKM) and two sample types (rhizosphere and bulk soil): (a) total phosphorus, (b) available phosphorus, (c) inorganic phosphorus, (d) organic phosphorus, (e) orthophosphate monoesters, (f) orthophosphate diesters, (g) Myo-IHP, (h) acidic phosphatase activity, (i) alkaline phosphatase activity. Significant differences between treatments in bulk soil are indicated by capital letters (p<0.05, n=4). Significant differences between treatments in rhizosphere are indicated by lowercase letters (p<0.05, n=4). Significant differences between rhizosphere and bulk soil are indicated by asterisks, where * p<0.05, p<0.01 (Duncan's test, n=4).
Table 1The soil properties in five treatments (CK, NPK, M, NPKM, NKM) and two sample types (bulk and rhizosphere soils).
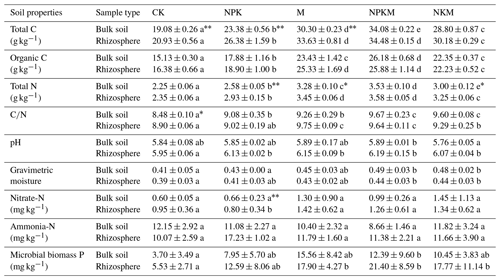
Values are means ± standard error. Significant differences between treatments are indicated by lowercase letters (p<0.05, n=4). Significant differences between rhizosphere and bulk soil are indicated by asterisks, where * p<0.05, p<0.01 (Duncan's test, n=4).
3.2 Soil P species
Long-term application of inorganic P fertilizer (NPK and NPKM) could significantly increase total P (TP), available P (AP) and inorganic P (IP) concentrations in both bulk and rhizosphere soils (Fig. 1a, b, and c). The concentrations of P extracted by NaOH–Na2EDTA in the soils were ∼ 243–739 mg kg−1, accounting for ∼ 38 %–66 % of total P (Table 2). Orthophosphate, pyrophosphate, orthophosphate monoesters (e.g., myo-IHP, scyllo-IHP) and orthophosphate diesters (e.g., DNA) were found in the soils (Table 2). The amounts of soil organic P (i.e., sum of orthophosphate monoesters and diesters) were not much and accounted for 8 %–30 % of total P (data not shown). Generally, the concentrations of organic P were higher with long-term manure fertilization compared to those of CK and NPK (Fig. 1d). Among the OP, the amounts of orthophosphate monoesters (57–96 mg kg−1) were higher than those of orthophosphate diesters (34–65 mg kg−1) (Table 2). The long-term manure amendments had an obvious effect on the accumulation of orthophosphate monoesters: the concentrations of orthophosphate monoesters and myo-IHP were significantly higher with manure fertilization (i.e., M, NPKM, NKM) than those with other treatments (i.e., CK, NPK) (Fig. 1e and g). The concentrations of orthophosphate diesters were also higher with manure treatments compared to CK and NPK although the tendency was not significant (Fig. 1f).
Table 2The phosphorus species in five treatments (CK, NPK, M, NPKM, and NKM) and two sample types (bulk and rhizosphere soils).
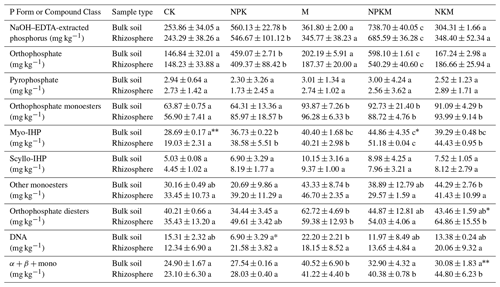
Myo-IHP: myo-Inositol hexakisphosphate; scyllo-IHP: scyllo-Inositol hexakisphosphate; α + β + mono, α- and β-glycerophosphates and mononucleotides. Values are means ± standard error. Significant differences between treatments are indicated by lowercase letters (p<0.05, n=2). Significant differences between rhizosphere and bulk soil are indicated by asterisks, where * p<0.05, p<0.01 (Duncan's test, n=2).
3.3 Long-term fertilization and rhizosphere effect on the composition of microbial community
The dominant bacteria for different treatments at the phylum level were Proteobacteria, Acidobacteria, Chloroflexi, and Nitrospirae and the dominant fungi were Ascomycota and Basidiomycota (Fig. 2). As the most abundant phylum of bacteria, Proteobacteria were further classified into Alphaproteobacteria, Betaproteobacteria, Gammaproteobacteria, Deltaproteobacteria, Epsilonproteobacteria and unclassed groups at the class level. Gammaproteobacteria were significantly more abundant for manure treatments than for CK and NPK treatments. The abundance of Epsilonproteobacteria increased after mineral fertilization. Both inorganic and organic fertilization could increase the abundance of Alphaproteobacteria (Fig. S3). On the other hand, certain bacteria and fungi at the phylum level affected by fertilization were different in rhizosphere and non-rhizosphere soils. For example, the long-term manure fertilization accumulated more Spirochaetes but less Actinobacteria and Saccharibacteria (TM7) in non-rhizosphere soils (Fig. 2A and C). The relative abundance of Ascomycota increased significantly with fertilization in non-rhizosphere soils but this was not the case in the rhizosphere soils (Fig. 2B and D). These results suggested that both long-term fertilization and rhizosphere affected the composition of the microbial community.
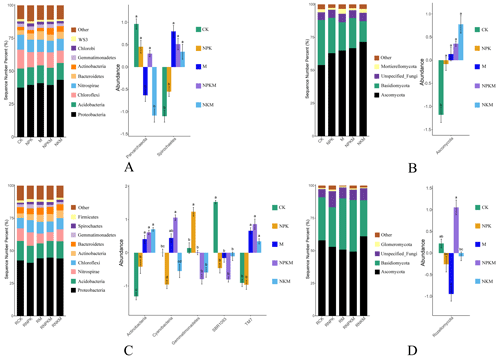
Figure 2The microbial relative abundance (left) and the features with significant differences (Anova + Duncan; p<0.05; n=6) between groups (right) at the phylum level in five treatments (CK, NPK, M, NPKM, and NKM). Capital letters shown with the bar graphs refer to different classifications (A – bacteria in bulk soil; B – fungi in bulk soil; C – bacteria in rhizosphere soil; D – fungi in rhizosphere soil).
The relative abundances of phosphate-solubilizing bacteria (PSB) were also greatly influenced by fertilization and rhizosphere. The Thiobacillus was the most abundant bacterium at genus level and increased with long-term input of inorganic P in both bulk and rhizosphere soils (Fig. 3A and C). Additionally, the long-term manure fertilization increased the abundance of Flavobacterium in bulk soil. On the other hand, the Fusarium was the most abundant fungus at genus level (Fig. 3B and D). The influence of fertilization on the phosphate-solubilizing fungi (PSF) in bulk soil was not obvious. However, manure fertilization increased the abundance of Aspergillus and Trichoderma in rhizosphere soils.
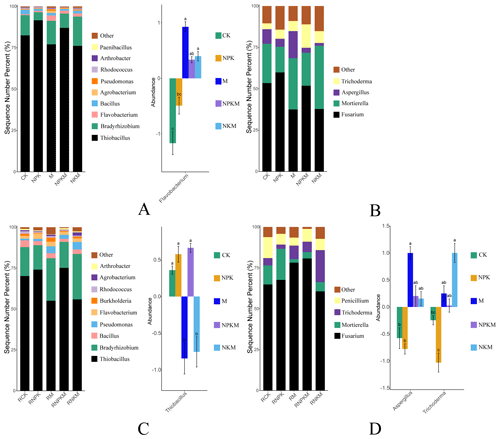
Figure 3The relative abundance of phosphate-solubilizing microorganisms (left) and features with significant differences (Anova + Duncan; p<0.05; n=6) between groups (right) at the genus level in five treatments (CK, NPK, M, NPKM, and NKM). Capital letters mean different classification (A – bacteria in bulk soil; B – fungi in bulk soil; C – bacteria in rhizosphere soil; D – fungi in rhizosphere soil).
3.4 Microbial community diversity
Soil with long-term mineral fertilization (NPK) presented a lower bacterial richness and evenness (i.e., Chao1 and Shannon index) than those with manure fertilization (M/NPKM/NKM) and even lower than control soil (CK), indicating that bacterial α diversity decreased after long-term mineral fertilizer regimes but was not changed under manure fertilization (Fig. 4). On the other hand, the rhizosphere effect was clearly observed in the bacterial diversity: the richness and evenness of the bacterial community in rhizosphere soil were significantly higher than those in non-rhizosphere soil (p<0.001). It is worth noting that fertilization and rhizosphere effect have no obvious influence on fungal richness and evenness. It suggested that long-term fertilization and rhizosphere affected the richness and evenness of bacterial and fungal communities differently.
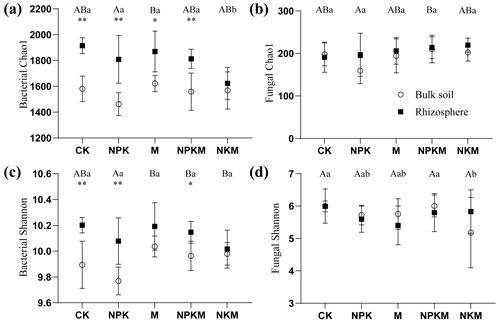
Figure 4Mean ± SE values for microbial α diversity: (a) bacterial Chao1 index, (b) fungal Chao1 index, (c) bacterial Shannon index and (d) fungal Shannon index in five treatments (CK, NPK, M, NPKM, and NKM) and two sample types (rhizosphere and bulk soil). Significant differences between treatments in bulk soil are indicated by capital letters (p<0.05; n=6). Significant differences between treatments in rhizosphere are indicated by lowercase letters (p<0.05, n=6). Significant differences between rhizosphere and bulk soils are indicated by asterisks, where * p<0.05, p<0.01 (Duncan's test; n=6).
The plot of NMDS identified the variations in microbial β diversity between different sites, with the response of bacterial β diversity being greater than that of fungal β diversity (Fig. 5). Specifically, the profiles of bacterial β diversity with manure fertilizations (M, NKPM, NKM) were clearly separated from that for CK soil (Fig. 5a and c). The analysis of similarities (ANOSIM) revealed that R values for rhizosphere soils between different fertilization treatments were higher than those for bulk soils (Table S2). Accordingly, the variations in bacterial β diversity of rhizosphere soils with manure fertilization were greater than that of bulk soils (Fig. 5a and c). These results indicate that manure fertilization and rhizosphere effect exacerbated the variation of bacterial β diversity.
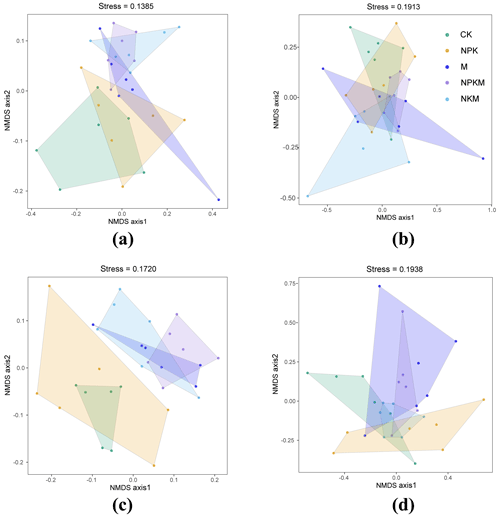
Figure 5Nonmetric multidimensional scaling (NMDS) ordination of the microbial community by comparing with Bray–Curtis distance similarities based on the abundance of OTUs. Lowercase letters mean different classification (a – bacteria in bulk soil; b – fungi in bulk soil; c – bacteria in rhizosphere soil; d – fungi in rhizosphere soil).
3.5 Co-occurrence networks
The co-occurrence network was used to analyze the ecological relationship of both bacterial and fungal communities under five fertilization treatments. After long-term mineral fertilization (NPK), total edges, average degree, positive edges and positive/negative edges ratio (i.e., ratio) of bacteria and fungi networks decreased (Fig. 6 and Table S3). Additionally, the high P input (NPKM vs. NKM) brought a larger and more complex but less stable bacterial network (e.g., more total nodes, edges, average degree, average clustering coefficient, average path length and less modularity). However, the opposite tendency was shown for fungus network (Fig. 6 and Table S3), indicating that the response of bacteria and fungi to the input of inorganic P was different.
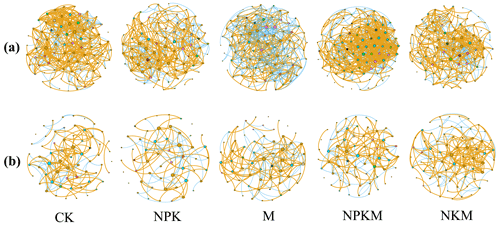
Figure 6Network of co-occurring (a) bacterial and (b) fungal OTUs across five fertilizer treatments. Only Spearman's correlation coefficient r>0.6 or significant at P<0.01 is shown. The nodes are colored according to phylum. Orange edges represent positive correlations and blue edges represent negative correlations. Node size presents the connecting numbers of each OUT.
3.6 Factors correlating with microbial community diversity
Redundancy analysis (RDA) was conducted to determine the correlation of soil properties with microbial community diversity in bulk and rhizosphere soils. The results showed that TC (10.4 %, F = 4.4, p = 0.03), soil pH value (10.3 %, F =4.4, p = 0.03), TN (10.1 %, F = 4.3, p = 0.03) and OC (9.2 %, F = 3.9, p = 0.03) were significantly correlated with bacterial community diversity (Fig. 7a, Table S4). On the other hand, for the fungus, the soil properties had extremely small explanations of <4.4 % for the variation for the fungal community (Table S4).
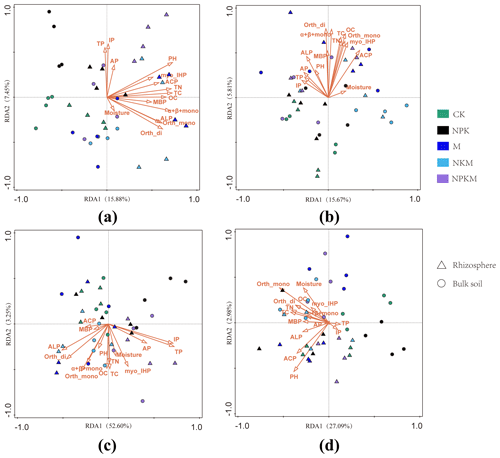
Figure 7Correlations between soil properties and the community structure of (a) total bacteria, (b) total fungi, (c) phosphorus-solubilizing bacteria and (d) phosphorus-solubilizing fungi as determined by redundancy analysis (RDA). MBP – microbial biomass phosphorus; TP – total phosphorus; IP – inorganic phosphorus; AP – available phosphorus; Orth-mono –orthophosphate monoester; Orth-di – orthophosphate diesters; Myo-IHP – myo-Inositol hexakisphosphate; α + β + mono – α- and β-glycerophosphates and mononucleotides; ACP – activity of acidic phosphatase; ALP – activity of alkaline phosphatase.
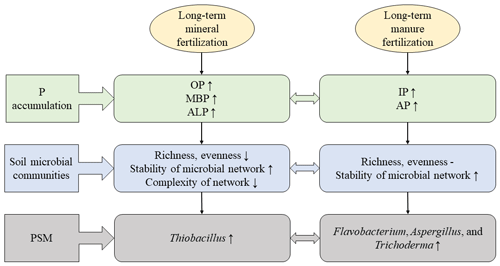
Figure 8A diagrammatic sketch showing different responses of P accumulation, soil microbial communities and the PSMs after long-term mineral or manure fertilization. The ↑ indicates an increase; the – indicates no effect; the ↓ indicates a decrease.
The RDA was also performed to establish the linkages of soil properties with community diversity of PSMs. The soil properties together explained more than 55 % of the variation in PSB community structure and those correlated with PSB contained TP (27.5%, F = 14.4, p = 0.03) and IP (26.6 %, F = 13.7, p = 0.03) (Fig. 7c and Table S5). The PSB were well separated by RDA1 (52.60 %) between the samples with inorganic P application (i.e., NPK, NPKM) and without inorganic P application (i.e., CK, M, NKM) (Fig. 7c). The 30.08 % of the total variance in the PSF community could be explained by the first and second axes (Fig. 7d).
4.1 Long-term fertilization on soil P accumulation
Long-term organic P fertilization increased the utilization of P for crops compared to inorganic P fertilization. The same amount of P was added to soil, whether with inorganic or organic fertilization, but the total P of soil was significantly higher with mineral fertilization compared to manure treatment, suggesting more P was retained in soil and less P was utilized by crops under long-term mineral fertilization (Fig. 1a). Several researchers have already reported that inorganic P was easily immobilized by clay minerals and was dominantly associated with amorphous oxides compared to crystalline oxide fractions in many soil types such as Sandy soils, Ultisols, Luvisol, Ferralic Cambisol and so on (Arai et al., 2005; Rick and Arai, 2011; Jiang et al., 2015; Ahmed et al., 2019). On the other hand, it has been confirmed that the application of manure usually leads to an increase in labile organic P pools, which are protected from the process of adsorption on clay minerals and are readily available to plants (Braos et al., 2020; Kashem et al., 2004). In this study, manure fertilization increased microbial biomass P concentration and alkaline phosphatase activity compared to mineral fertilization (Figs. 1i and 8; Table 1). It is possible that the mineralization of organic P such as orthophosphate diesters from microbes by alkaline phosphatase increased under organic fertilization, thus improving the P availability for crops.
The application of inorganic P fertilizer mainly increased the concentration of inorganic P but manure fertilization accelerated the accumulation of organic P in soil, which was consistent with our hypotheses (Figs. 1c, d and 8). Phosphorus speciation is usually regulated by the changes in soil mineralogy, mineral and organic P inputs, biological production and the utilization of various P species (Turner et al., 2007; Jiang et al., 2017). Fertilization, especially for manure, accelerated the accrual of organic carbon (Table 1) significantly, which also co-accumulated organic P. Generally, the content of organic phosphorus (OP) from manures accounts for a large proportion of total P, among which inositol phosphate (IHP) was the most abundant OP (Maguire et al., 2004). Therefore, long-term manure fertilization also increased the input of OP in the field. The organic P could be effectively mineralized by microorganisms and thus transferred into various inorganic P fractions (Song et al., 2007).
The application of manure increased the accumulation of orthophosphate monoesters significantly, especially for myo-inositol phosphates (myo-IHP) (Fig. 1e and g). Normally, phosphate monoesters were the main group of organic P compounds and existed as IHP mainly in most soils (Turner et al., 2005). Those orthophosphate monoesters were commonly stabilized by association with soil minerals such as oxides (Celi and Barberis, 2007; Turner and Engelbrecht, 2011; Jiang et al., 2015). Therefore, the stability and immobilization of orthophosphate monoesters promoted their accumulation in soil, regardless of the input of manure or the P transformation. On the other hand, separate manure fertilization (M) also increased the contents of orthophosphate diesters significantly (Fig. 1f). Long-term manure fertilization accumulated more microbial biomass P significantly (Table 1) that were rich in orthophosphate diesters (Turner et al., 2007). The accumulation of orthophosphate diesters under manure fertilization was probably due to the reduced decomposition of plant residues and manure or increased microbial synthesis under anaerobic paddy-rice management (Jiang et al., 2017).
4.2 Long-term fertilization and rhizosphere effect on soil microbial communities
Our results indicated that long-term mineral fertilization decreased bacterial richness, evenness and the decrease of complexation of bacterial networks, indicating that long-term mineral fertilization increased the stability of microbial network (e.g., lower ratio) but decreased the complexity of network (e.g., less total edges and lower average degree; Fig. 8; Tu et al., 2020; Olesen et al., 2007; Hernandez et al., 2021). On the other hand, long-term organic fertilization did not change the bacterial richness and evenness; it even promoted the separation of bacterial communities (Fig. 8). This conclusion was also expected in our hypothesis. Meanwhile, long-term manure treatments (M, NPKM, NKM) increased the negative connections of microorganisms and also promoted the stability of network (Zhou et al., 2020). Previous studies have reported that long-term mineral fertilization changed soil properties and these perturbations may have an adverse effect on soil microbes (Marschner et al., 2003; Geisseler and Scow, 2014; Liang et al., 2020). In contrast, organic fertilizers contain a large amount of organic matter which could be utilized by soil bacteria (Wu et al., 2020, 2021).
Additionally, there were significant increases for diversity of bacterial communities in rhizosphere soil compared to bulk soil. Generally, microbes concentrated in the rhizosphere where organic compounds were released by plant roots (Achat et al., 2010), and plants tend to recruit bacteria as symbiotic microbes by releasing phenolic compounds (Gkarmiri et al., 2017; Badri et al., 2013).
Accordingly, redundancy analysis showed that the key factors related to the shift of bacterial communities included pH, TC, TN and OC. The previous study showed that the soil bacterial community was indirectly impacted by pH via the alteration of metals and nutrient availability (Xiao et al., 2021) and directly modulated by the abundance and mineralization of carbon in soil (Chen et al., 2019) as well as soil nitrogen deposition (Zeng et al., 2016). In this study, the long-term organic fertilization and rhizosphere soil accumulated more TC, TN and OC, which provided more nutrients, changed the soil pH, and thus drove the shift of bacterial communities (Ingwersen et al., 2008; Liu et al., 2019).
Additionally, the application of both mineral and organic fertilizers increased the stability of bacterial networks (i.e., increasing negative correlations). Compared to CK, long-term fertilization provided more nutrient elements, stimulated the growth and competition of bacteria, and finally facilitated the stability of ecological networks (Faust and Raes, 2012; Simard et al., 2012).
It is worth noting that fertilization and rhizosphere effect had no obvious influence on the fungal community structure. Redundancy analysis showed that the explanations of soil properties were extremely small for the variation for the fungal community. It has been found that fungi were less sensitive to soil substrates and environmental conditions whereas bacteria were more sensitive (Dong et al., 2014). The high TOC provided by the long-term fertilization and rhizosphere soil gave an advantage for bacteria to compete with fungi for resources, thus decreasing influences of long-term fertilization and rhizosphere on fungi (Zelezniak et al., 2015).
4.3 Response of PSMs
Thiobacillus was the most abundant PSB at genus level and increased with the input of inorganic P fertilizers in bulk and rhizosphere soils (Figs. 3A, C and 8). It was involved in sulfur oxidation, and acidity that resulted from sulfur oxidation could solubilize mineral P (Aria et al., 2010). Acidic and anaerobic conditions provided by paddy-rice management of red soil in this study were beneficial for the growth of Thiobacillus considering that it belongs to acidophilic bacteria (Monachon et al., 2019; Kumar et al., 2020). The applied calcium superphosphate as inorganic P fertilizer in this study contained a certain amount of CaSO4, therefore the input of inorganic P fertilizer also provided S source for the growth of Thiobacillus. On the other hand, Fusarium was the most abundant PSF at genus level (Fig. 3B and D) and proved to produce organic acid to solute the mineral P (Elias et al., 2016). It was known that Fusarium was widely distributed in soil around the world and acted as a saprophyte (Deacon, 1997), among which many species were also found as phytopathogens (Suga and Hyakumachi, 2004).
Besides, the long-term organic fertilization increased the abundance of Flavobacterium, Aspergillus and Trichoderma (Fig. 8). Flavobacterium was associated with the degradation of phosphotriester (Brown, 1980) and proved to grow under nutrient-rich conditions (Kraut-Cohen et al., 2021). Aspergillus, as a saprophytic fungus, could produce organic acid to dissolve mineral phosphorus (Li et al., 2016) and also preferred nutrient-rich conditions (Martins et al., 2014). Additionally, Trichoderma as a biological control fungus (Zin and Badaluddin, 2020) was colonized in the root epidermis and outer cortical layers (Harman, 2006). Long-term organic fertilization provided more organic matter for these microbes.
The PSMs could solubilize mineral P and mineralize organic P (Sharma et al., 2013). The PSB of samples with inorganic P input (i.e., NPK, NPKM) and non-mineral P application (i.e., CK, M, NKM) could be well separated, indicating that mineral P had a strong effect on the community diversity of PSB. Correspondingly, TP and IP were key factors driving the diversity of the soil PSB community and those indicators were all significantly higher with inorganic P amendments (Fig. 1a, and c). As discussed before, Thiobacillus as the most abundant PSB at genus level in this study increased with the input of mineral P because the mineral P could provide additional S source for the growth of Thiobacillus. Furthermore, the availability of P in soil was considered a key condition for PSMs to express P-solubilization traits. Low availability of P in soil is widely considered as a favorable condition for PSMs, whereas recent studies suggested that a minimum P threshold is required to achieve a response by plants (Sánchez-Esteva et al., 2016; Gómez-Muñoz et al., 2018; Raymond et al., 2021).
Long-term inorganic and organic fertilization managements brought different effects on P accumulation, microbial community and PSB. Long-term mineral fertilization increased inorganic and available P concentrations, while manure fertilization increased soil organic P concentrations, microbial biomass P contents and potential organic P mineralization. The turnover of P by bacteria seems strong under long-term organic fertilization and rhizosphere soil considering that more organic nutrients were provided for bacteria and the bacterial community diversity increased. Furthermore, inorganic P fertilization increased the abundance of Thiobacillus whereas organic fertilization increased the abundance of Flavobacterium, Aspergillus and Trichoderma. The concentrations of TP and IP strongly influenced by inorganic P fertilization were key factors driving the diversity of the soil PSB community. These findings provide useful insights into P accumulation, turnover and soil P sustainable fertility under different fertilization strategies.
The R code generated during this current study is available from the corresponding author upon reasonable request.
No data sets were used in this article.
The supplement related to this article is available online at: https://doi.org/10.5194/soil-9-101-2023-supplement.
SC: investigation, data curation, formal analysis, original draft preparation and visualization. JG: resources and data curation. HC: formal analysis, data curation and visualization. ZZ: investigation and resources. JH: resources. LL: investigation. JT: Conceptualization, supervision and project administration. XJ: methodology, reviewing and editing, project administration and funding acquisition.
The contact author has declared that none of the authors has any competing interests.
Publisher’s note: Copernicus Publications remains neutral with regard to jurisdictional claims in published maps and institutional affiliations.
This study was financially supported by the National Natural Science Foundation of China (grant no. 41907063) and Special Project of Hunan Innovative Province Construction (grant no. 2022JJ30648).
This paper was edited by Ping He and reviewed by two anonymous referees.
Achat, D., Bakker, M., Zeller, B. O. B., Pellerin, S., Bienaimé, S., and Morel, C.: Long-term organic phosphorus mineralization in Spodosols under forests and its relation to carbon and nitrogen mineralization, Soil Biol. Biochem., 42, 1479–1490, https://doi.org/10.1016/j.soilbio.2010.05.020, 2010.
Acosta-Martínez, V. and Ali Tabatabai, M.: Phosphorus Cycle Enzymes, in: Methods of Soil Enzymology, edited by: Dick, R. P., SSSA Book Series, 161–183, https://doi.org/10.2136/sssabookser9.c8, 2011.
Ahmed, W., Liu, K., Qaswar, M., Huang, J., Huang, Q., Xu, Y., Ali, S., Mehmood, S., Ammar Asghar, R. M., Mahmood, M., and Zhang, H.: Long-term mineral fertilization improved the grain yield and phosphorus use efficiency by changing soil P fractions in ferralic cambisol, Agronomy, 9, 784, https://doi.org/10.3390/agronomy9120784, 2019.
Alori, E. T., Glick, B. R., and Babalola, O. O.: Microbial phosphorus solubilization and its potential for use in sustainable agriculture, Front Microbiol., 8, 971, https://doi.org/10.3389/fmicb.2017.00971, 2017.
Arai, Y., Livi, K. J. T., and Sparks, D. L.: Phosphate reactivity in long-term poultry litter-amended southern delaware sandy soils, Soil Sci. Soc. Am. J., 69, 616–629, https://doi.org/10.2136/sssaj2004.0218, 2005.
Aria, M. M., Lakzian, A., Haghnia, G. H., Berenji, A. R., Besharati, H., and Fotovat, A.: Effect of Thiobacillus, sulfur, and vermicompost on the water-soluble phosphorus of hard rock phosphate, Bioresource Technol., 101, 551–554, https://doi.org/10.1016/j.biortech.2009.07.093, 2010.
Babalola, O. and Glick, B.: Indigenous African agriculture and plant associated microbes: Current practice and future transgenic prospects, Sci. Res. Essays, 7, 2431–2439, https://doi.org/10.5897/SRE11.1714, 2012.
Badri, D. V., Chaparro, J. M., Zhang, R., Shen, Q., and Vivanco, J. M.: Application of natural blends of phytochemicals derived from the root exudates of Arabidopsis to the soil reveal that phenolic-related compounds predominantly modulate the soil microbiome, J. Biol. Chem., 288, 4502–4512, https://doi.org/10.1074/jbc.M112.433300, 2013.
Baxter, S.: World Reference Base for Soil Resources. World Soil Resources Report 103, Food and Agriculture Organization of the United Nations, Rome (2006), 132 pp., ISBN 92-5-10511-4, 2007.
Blaalid, R., Kumar, S., Nilsson, R. H., Abarenkov, K., Kirk, P. M., and Kauserud, H.: ITS1 versus ITS2 as DNA metabarcodes for fungi, Mol. Ecol. Resour., 13, 218–224, https://doi.org/10.1111/1755-0998.12065, 2013.
Bokulich, N. A., Kaehler, B. D., Rideout, J. R., Dillon, M., Bolyen, E., Knight, R., Huttley, G. A., and Gregory Caporaso, J.: Optimizing taxonomic classification of marker-gene amplicon sequences with QIIME 2's q2-feature-classifier plugin, Microbiome, 6, 90, https://doi.org/10.1186/s40168-018-0470-z, 2018.
Bolyen, E., Rideout, J. R., Dillon, M. R., Bokulich, N. A., Abnet, C. C., Al-Ghalith, G. A., Alexander, H., Alm, E. J., Arumugam, M., Asnicar, F., Bai, Y., Bisanz, J. E., Bittinger, K., Brejnrod, A., Brislawn, C. J., Brown, C. T., Callahan, B. J., Caraballo-Rodríguez, A. M., Chase, J., Cope, E. K., Da Silva, R., Diener, C., Dorrestein, P. C., Douglas, G. M., Durall, D. M., Duvallet, C., Edwardson, C. F., Ernst, M., Estaki, M., Fouquier, J., Gauglitz, J. M., Gibbons, S. M., Gibson, D. L., Gonzalez, A., Gorlick, K., Guo, J., Hillmann, B., Holmes, S., Holste, H., Huttenhower, C., Huttley, G. A., Janssen, S., Jarmusch, A. K., Jiang, L., Kaehler, B. D., Kang, K. B., Keefe, C. R., Keim, P., Kelley, S. T., Knights, D., Koester, I., Kosciolek, T., Kreps, J., Langille, M. G. I., Lee, J., Ley, R., Liu, Y.-X., Loftfield, E., Lozupone, C., Maher, M., Marotz, C., Martin, B. D., McDonald, D., McIver, L. J., Melnik, A. V., Metcalf, J. L., Morgan, S. C., Morton, J. T., Naimey, A. T., Navas-Molina, J. A., Nothias, L. F., Orchanian, S. B., Pearson, T., Peoples, S. L., Petras, D., Preuss, M. L., Pruesse, E., Rasmussen, L. B., Rivers, A., Robeson, M. S., Rosenthal, P., Segata, N., Shaffer, M., Shiffer, A., Sinha, R., Song, S. J., Spear, J. R., Swafford, A. D., Thompson, L. R., Torres, P. J., Trinh, P., Tripathi, A., Turnbaugh, P. J., Ul-Hasan, S., van der Hooft, J. J. J., Vargas, F., Vázquez-Baeza, Y., Vogtmann, E., von Hippel, M., Walters, W., Wan, Y., Wang, M., Warren, J., Weber, K. C., Williamson, C. H. D., Willis, A. D., Xu, Z. Z., Zaneveld, J. R., Zhang, Y., Zhu, Q., Knight, R., and Caporaso, J. G.: Reproducible, interactive, scalable and extensible microbiome data science using QIIME 2, Nat. Biotechnol., 37, 852–857, https://doi.org/10.1038/s41587-019-0209-9, 2019.
Bowman, R. A.: A Sequential Extraction Procedure with Concentrated Sulfuric Acid and Dilute Base for Soil Organic Phosphorus, Soil Sci. Soc. Am. J., 53, 362–366, https://doi.org/10.2136/sssaj1989.03615995005300020008x, 1989.
Braos, L. B., Bettiol, A. C. T., Di Santo, L. G., Ferreira, M. E., and Cruz, M. C. P.: Dynamics of phosphorus fractions in soils treated with dairy manure, Soil Res., 58, 289–298, https://doi.org/10.1071/SR18325, 2020.
Brookes, P. C., Powlson, D. S., and Jenkinson, D. S.: Measurement of microbial biomass phosphorus in soil, Soil Biol. Biochem., 14, 319–329, https://doi.org/10.1016/0038-0717(82)90001-3, 1982.
Brown, K. A.: Phosphotriesterases of flavobacterium sp., Soil Biol. Biochem., 12, 105–112, https://doi.org/10.1016/0038-0717(80)90044-9, 1980.
Cade-Menun, B. J.: Improved peak identification in 31P-NMR spectra of environmental samples with a standardized method and peak library, Geoderma, 257–258, 102–114, https://doi.org/10.1016/j.geoderma.2014.12.016, 2015.
Cade-Menun, B. J., Carter, M. R., James, D. C., and Liu, C. W.: Phosphorus forms and chemistry in the soil profile under long-term conservation tillage: A phosphorus-31 nuclear magnetic resonance study, J. Environ. Qual., 39, 1647–1656, https://doi.org/10.2134/jeq2009.0491, 2010.
Callahan, B. J., McMurdie, P. J., Rosen, M. J., Han, A. W., Johnson, A. J. A., and Holmes, S. P.: DADA2: High-resolution sample inference from Illumina amplicon data, Nat. Methods, 13, 581–583, https://doi.org/10.1038/nmeth.3869, 2016.
Caporaso, J. G., Lauber, C. L., Walters, W. A., Berg-Lyons, D., Huntley, J., Fierer, N., Owens, S. M., Betley, J., Fraser, L., Bauer, M., Gormley, N., Gilbert, J. A., Smith, G., and Knight, R.: Ultra-high-throughput microbial community analysis on the Illumina HiSeq and MiSeq platforms, ISME J., 6, 1621–1624, https://doi.org/10.1038/ismej.2012.8, 2012.
Celi, L. and Barberis, E.: Abiotic reactions of inositol phosphates in soil. Inositol phosphates, in: Inositol phosphates: Linking agriculture and the environment, 1st Edn., edited by: Turner, B. L., Richardson, A. E., and Mullaney, E. J., CABI Publishing, Wallingford, Oxfordshire, England, 207–220, https://doi.org/10.1079/9781845931520.0207, 2007.
Chen, H., Zhao, X., Lin, Q., Li, G., and Kong, W.: Using a combination of PLFA and DNA-based sequencing analyses to detect shifts in the soil microbial community composition after a simulated spring precipitation in a semi-arid grassland in China, Sci. Total Environ., 657, 1237–1245, https://doi.org/10.1016/j.scitotenv.2018.12.126, 2019.
Deacon, J. W.: Modern mycology, 3rd Edn., Blackwell Science Oxford, Hoboken, New Jersey, ISBN-10 0632030771, 1997.
Dennis, K. L., Wang, Y., Blatner, N. R., Wang, S., Saadalla, A., Trudeau, E., Roers, A., Weaver, C. T., Lee, J. J., Gilbert, J. A., Chang, E. B., and Khazaie, K.: Adenomatous polyps are driven by microbe-instigated focal inflammation and are controlled by IL-10–Producing T cells, Cancer Res., 73, 5905–5913, https://doi.org/10.1158/0008-5472.CAN-13-1511, 2013.
Dong, W.-Y., Zhang, X., Dai, X.-Q., Fu, X., Yang, F.-T., Liu, X.-Y., Sun, X., Wen, X., and Schaeffer, S.: Changes in soil microbial community composition in response to fertilization of paddy soils in subtropical China, Appl. Soil Ecol., 84, 140–147, https://doi.org/10.1016/j.apsoil.2014.06.007, 2014.
Dorich, R. A. and Nelson, D. W.: Direct colorimetric measurement of ammonium in potassium chloride extracts of soils, Soil Sci. Soc. Am. J., 47, 833–836, https://doi.org/10.2136/sssaj1983.03615995004700040042x, 1983.
Elias, F., Woyessa, D., and Muleta, D.: Phosphate solubilization potential of rhizosphere Fungi isolated from plants in Jimma Zone, Southwest Ethiopia, Int. J. Microbiol., 2016, 5472601, https://doi.org/10.1155/2016/5472601, 2016.
Faust, K. and Raes, J.: Microbial interactions: from networks to models, Nat. Rev. Microbiol., 10, 538–550, https://doi.org/10.1038/nrmicro2832, 2012.
Gao, J., Cao, W., Li, D., Xu, M., Zeng, X., Nie, J., and Zhang, W.: Effects of long-term double-rice and green manure rotation on rice yield and soil organic matter in paddy field, Acta Ecol. Sin., 31, 4542–4548, 2011.
Geisseler, D. and Scow, K. M.: Long-term effects of mineral fertilizers on soil microorganisms – A review, Soil Biol. Biochem., 75, 54–63, https://doi.org/10.1016/j.soilbio.2014.03.023, 2014.
Gkarmiri, K., Mahmood, S., Ekblad, A., Alström, S., Högberg, N., Finlay, R., and Loeffler Frank, E.: Identifying the Active Microbiome Associated with Roots and Rhizosphere Soil of Oilseed Rape, Appl. Environ. Microb., 83, e01938-17, https://doi.org/10.1128/AEM.01938-17, 2017.
Gómez-Muñoz, B., Jensen, L. S., de Neergaard, A., Richardson, A. E., and Magid, J.: Effects of Penicillium bilaii on maize growth are mediated by available phosphorus, Plant Soil, 431, 159–173, https://doi.org/10.1007/s11104-018-3756-9, 2018.
Grant, C., Bittman, S., Montreal, M., Plenchette, C., and Morel, C.: Soil and fertilizer phosphorus: Effects on plant P supply and mycorrhizal development, Can. J. Plant Sci., 85, 3–14, https://doi.org/10.4141/p03-182, 2005.
Harman, G. E.: Overview of mechanisms and uses of Trichoderma spp., Phytopathology®, 96, 190–194, https://doi.org/10.1094/PHYTO-96-0190, 2006.
Hernandez, D. J., David, A. S., Menges, E. S., Searcy, C. A., and Afkhami, M. E.: Environmental stress destabilizes microbial networks, ISME J., 15, 1722–1734, https://doi.org/10.1038/s41396-020-00882-x, 2021.
Ingwersen, J., Poll, C., Streck, T., and Kandeler, E.: Micro-scale modelling of carbon turnover driven by microbial succession at a biogeochemical interface, Soil Biol. Biochem., 40, 864–878, https://doi.org/10.1016/j.soilbio.2007.10.018, 2008.
Jiang, X., Bol, R., Willbold, S., Vereecken, H., and Klumpp, E.: Speciation and distribution of P associated with Fe and Al oxides in aggregate-sized fraction of an arable soil, Biogeosciences, 12, 6443–6452, https://doi.org/10.5194/bg-12-6443-2015, 2015.
Jiang, X., Amelung, W., Cade-Menun, B. J., Bol, R., Willbold, S., Cao, Z., and Klumpp, E.: Soil organic phosphorus transformations during 2000 years of paddy-rice and non-paddy management in the Yangtze River Delta, China, Sci. Rep.-UK, 7, 10818, https://doi.org/10.1038/s41598-017-10071-0, 2017.
Kashem, M. A., Akinremi, O. O., and Racz, G. J.: Phosphorus fractions in soil amended with organic and inorganic phosphorus sources, Can. J. Soil. Sci., 84, 83–90, https://doi.org/10.4141/S03-018, 2004.
Kraut-Cohen, J., Shapiro, O. H., Dror, B., and Cytryn, E.: Pectin induced colony expansion of soil-derived Flavobacterium strains, Front. Microbiol., 12, 651891, https://doi.org/10.3389/fmicb.2021.651891, 2021.
Kumar, M., Zeyad, M. T., Choudhary, P., Paul, S., Chakdar, H., and Singh Rajawat, M. V.: Thiobacillus, in: Beneficial Microbes in Agro-Ecology, 1st Edn., edited by: Amaresan, N., Senthil Kumar, M., Annapurna, K., Kumar, K., and Sankaranarayanan, A., Academic Press, 545–557, https://doi.org/10.1016/B978-0-12-823414-3.00026-5, 2020.
Li, Z., Bai, T., Dai, L., Wang, F., Tao, J., Meng, S., Hu, Y., Wang, S., and Hu, S.: A study of organic acid production in contrasts between two phosphate solubilizing fungi: Penicillium oxalicum and Aspergillus niger, Sci. Rep.-UK, 6, 25313, https://doi.org/10.1038/srep25313, 2016.
Liang, R., Hou, R., Li, J., Lyu, Y., Hang, S., Gong, H., and Ouyang, Z.: Effects of different fertilizers on rhizosphere bacterial communities of winter wheat in the North China plain, Agronomy, 10, 93, https://doi.org/10.3390/agronomy10010093, 2020.
Liu, J., Hu, Y., Yang, J., Abdi, D., and Cade-Menun, B. J.: Investigation of soil legacy phosphorus transformation in long-term agricultural fields using sequential fractionation, P K-edge XANES and solution P NMR spectroscopy, Environ. Sci. Technol., 49, 168–176, https://doi.org/10.1021/es504420n, 2015.
Liu, M., Sui, X., Hu, Y., and Feng, F.: Microbial community structure and the relationship with soil carbon and nitrogen in an original Korean pine forest of Changbai Mountain, China, BMC Microbiol., 19, 218, https://doi.org/10.1186/s12866-019-1584-6, 2019.
Maguire, R. O., Sims, J. T., Saylor, W. W., Turner, B. L., Angel, R., and Applegate, T. J.: Influence of phytase addition to poultry diets on phosphorus forms and solubility in litters and amended soils, J. Environ. Qual., 33, 2306–2316, https://doi.org/10.2134/jeq2004.2306, 2004.
Marschner, P., Kandeler, E., and Marschner, B.: Structure and function of the soil microbial community in a long-term fertilizer experiment, Soil Biol. Biochem., 35, 453–461, https://doi.org/10.1016/S0038-0717(02)00297-3, 2003.
Martins, I., Hartmann, D. O., Alves, P. C., Martins, C., Garcia, H., Leclercq, C. C., Ferreira, R., He, J., Renaut, J., Becker, J. D., and Silva Pereira, C.: Elucidating how the saprophytic fungus Aspergillus nidulans uses the plant polyester suberin as carbon source, BMC Genomics., 15, 613, https://doi.org/10.1186/1471-2164-15-613, 2014.
McDowell, R. W., Stewart, I., and Cade-Menun, B. J.: An examination of spin–lattice relaxation times for analysis of soil and manure extracts by liquid state phosphorus-31 nuclear magnetic resonance spectroscopy, J Environ. Qual., 35, 293–302, https://doi.org/10.2134/jeq2005.0285, 2006.
Monachon, M., Albelda-Berenguer, M., and Joseph, E.: Biological oxidation of iron sulfides, in: Advances in Applied Microbiology, 1st Edn., edited by: Gadd, G. M. and Sariaslani, S., Academic Press, Cambridge, Massachusetts, USA, 1–27, https://doi.org/10.1016/bs.aambs.2018.12.002, 2019.
Murphy, J. and Riley, J. P.: A modified single solution method for the determination of phosphate in natural waters, Anal. Chim. Acta, 27, 31–36, https://doi.org/10.1016/S0003-2670(00)88444-5, 1962.
Nannipieri, P., Giagnoni, L., Landi, L., and Renella, G.: Role of Phosphatase Enzymes in Soil, in: Soil Biology, edited by: Bünemann, E., Oberson, A., and Frossard, E., Springer, 215–243, https://doi.org/10.1007/978-3-642-15271-9_9, 2011.
Norman, R. J., Edberg, J. C., and Stucki, J. W.: Determination of nitrate in soil extracts by dual-wavelength ultraviolet spectrophotometry, Soil Sci. Soc. Am. J., 49, 1182–1185, https://doi.org/10.2136/sssaj1985.03615995004900050022x, 1985.
Olesen, J. M., Bascompte, J., Dupont, Y. L., and Jordano, P.: The modularity of pollination networks, P. Natl. Acad. Sci., 104, 19891–19896, https://doi.org/10.1073/pnas.0706375104, 2007.
Olsen, S. R., Cole, C. V., Watanabe, F. S., and Dean, L. A.: Estimation of available phosphorus in soils by extraction with sodium bicarbonate, 1st, Miscellaneous Paper Institute for Agricultural Research, Washington, D.C., U.S. Government Printing Office, USDA Circular no. 939, 1954.
Qaswar, M., Jing, H., Ahmed, W., Dongchu, L., Shujun, L., Lu, Z., Cai, A., Lisheng, L., Yongmei, X., Jusheng, G., and Huimin, Z.: Yield sustainability, soil organic carbon sequestration and nutrients balance under long-term combined application of manure and inorganic fertilizers in acidic paddy soil, Soil Till. Res., 198, 104569, https://doi.org/10.1016/j.still.2019.104569, 2020.
Raymond, N. S., Gómez-Muñoz, B., van der Bom, F. J. T., Nybroe, O., Jensen, L. S., Müller-Stöver, D. S., Oberson, A., and Richardson, A. E.: Phosphate-solubilising microorganisms for improved crop productivity: a critical assessment, New Phytol., 229, 1268–1277, https://doi.org/10.1111/nph.16924, 2021.
Rick, A. R. and Arai, Y.: Role of natural nanoparticles in phosphorus transport processes in ultisols, Soil Sci. Soc. Am. J., 75, 335–347, https://doi.org/10.2136/sssaj2010.0124nps, 2011.
Rodríguez, H. and Fraga, R.: Phosphate solubilizing bacteria and their role in plant growth promotion, Biotechnol. Adv., 17, 319–339, https://doi.org/10.1016/S0734-9750(99)00014-2, 1999.
Sánchez-Esteva, S., Gómez-Muñoz, B., Jensen, L. S., de Neergaard, A., and Magid, J.: The effect of Penicillium bilaii on wheat growth and phosphorus uptake as affected by soil pH, soil P and application of sewage sludge, Chem. Biochem. Tech. Agr., 3, 21, https://doi.org/10.1186/s40538-016-0075-3, 2016.
Schneider, K. D., Cade-Menun, B. J., Lynch, D. H., and Voroney, R. P.: Soil phosphorus forms from organic and conventional forage fields, Soil Sci. Soc. Am. J., 80, 328–340, https://doi.org/10.2136/sssaj2015.09.0340, 2016.
Schumacher, B. A.: Methods for the determination of total organic carbon (TOC) in soils and sediments, Ecological Risk Assessment Support Center Office of Research and Development, Washington, DC, EPA/600/R-02/069(NTISPB2003-100822), 2002.
Shao, J., Miao, Y., Liu, K., Ren, Y., Xu, Z., Zhang, N., Feng, H., Shen, Q., Zhang, R., and Xun, W.: Rhizosphere microbiome assembly involves seed-borne bacteria in compensatory phosphate solubilization, Soil Biol. Biochem., 159, 108273, https://doi.org/10.1016/j.soilbio.2021.108273, 2021.
Sharma, S. B., Sayyed, R. Z., Trivedi, M. H., and Gobi, T. A.: Phosphate solubilizing microbes: sustainable approach for managing phosphorus deficiency in agricultural soils, SpringerPlus, 2, 587, https://doi.org/10.1186/2193-1801-2-587, 2013.
Sharpley, A. and Moyer, B.: Phosphorus forms in manure and compost and their release during simulated rainfall, J. Environ. Qual., 29, 1462–1469, https://doi.org/10.2134/jeq2000.00472425002900050012x, 2000.
Simard, S. W., Beiler, K. J., Bingham, M. A., Deslippe, J. R., Philip, L. J., and Teste, F. P.: Mycorrhizal networks: Mechanisms, ecology and modelling, Fungal Biol. Rev., 26, 39–60, https://doi.org/10.1016/j.fbr.2012.01.001, 2012.
Song, C., Han, X. Z., and Tang, C.: Changes in phosphorus fractions, sorption and release in udic mollisols under different ecosystems, Biol. Fert. Soils, 44, 37–47, https://doi.org/10.1007/s00374-007-0176-z, 2007.
Suga, H. and Hyakumachi, M.: Genomics of phytopathogenic fusarium, in: Applied Mycology and Biotechnology, 4th Edn., edited by: Arora, D. K. and Khachatourians, G. G., Elsevier, Amsterdam, 161–189, https://doi.org/10.1016/S1874-5334(04)80009-1, 2004.
Tabatabai, M. A. and Bremner, J. M.: Use of p-nitrophenyl phosphate for assay of soil phosphatase activity, Soil Biol. Biochem., 1, 301–307, https://doi.org/10.1016/0038-0717(69)90012-1, 1969.
Tao, L., Wen, X., Li, H., Huang, C., Jiang, Y., Liu, D., and Sun, B.: Influence of manure fertilization on soil phosphorous retention and clay mineral transformation: Evidence from a 16-year long-term fertilization experiment, Appl. Clay Sci., 204, 106021, https://doi.org/10.1016/j.clay.2021.106021, 2021.
Tu, Q., Yan, Q., Deng, Y., Michaletz, S. T., Buzzard, V., Weiser, M. D., Waide, R., Ning, D., Wu, L., He, Z., and Zhou, J.: Biogeographic patterns of microbial co-occurrence ecological networks in six American forests, Soil Biol. Biochem., 148, 107897, https://doi.org/10.1016/j.soilbio.2020.107897, 2020.
Turner, B. L. and Engelbrecht, B. M. J.: Soil organic phosphorus in lowland tropical rain forests, Biogeochemistry, 103, 297–315, https://doi.org/10.1007/s10533-010-9466-x, 2011.
Turner, B. L., Cade-Menun, B. J., Condron, L. M., and Newman, S.: Extraction of soil organic phosphorus, Talanta, 66, 294–306, https://doi.org/10.1016/j.talanta.2004.11.012, 2005.
Turner, B. L., Condron, L. M., Richardson, S. J., Peltzer, D. A., and Allison, V. J.: Soil organic phosphorus transformations during pedogenesis, Ecosystems, 10, 1166–1181, https://doi.org/10.1007/s10021-007-9086-z, 2007.
Wen, Y., Liu, W., Deng, W., He, X., and Yu, G.: Impact of agricultural fertilization practices on organo-mineral associations in four long-term field experiments: Implications for soil C sequestration, Sci. Total Environ., 651, 591–600, https://doi.org/10.1016/j.scitotenv.2018.09.233, 2019.
WRB: World Reference Base for soil resources 2014: international soil classification system for naming soils and creating legends for soil maps, Food and Agriculture Organization of the United Nations, Rome, ISSN0532-0488, 2014.
Wu, J., Sha, C., Wang, M., Ye, C., Li, P., and Huang, S.: Effect of organic fertilizer on soil bacteria in maize fields, Land, 10, 328, https://doi.org/10.3390/land10030328, 2021.
Wu, L., Jiang, Y., Zhao, F., He, X., Liu, H., and Yu, K.: Increased organic fertilizer application and reduced chemical fertilizer application affect the soil properties and bacterial communities of grape rhizosphere soil, Sci. Rep.-UK, 10, 9568, https://doi.org/10.1038/s41598-020-66648-9, 2020.
Xiao, E., Ning, Z., Xiao, T., Sun, W., and Jiang, S.: Soil bacterial community functions and distribution after mining disturbance, Soil Biol. Biochem., 157, 108232, https://doi.org/10.1016/j.soilbio.2021.108232, 2021.
Yang, Z.-P., Xu, M.-G., Zheng, S.-X., Nie, J., Gao, J.-S., Liao, Y.-L., and Xie, J.: Effects of Long-Term Winter Planted Green Manure on Physical Properties of Reddish Paddy Soil Under a Double-Rice Cropping System, J. Integr. Agr., 11, 655–664, https://doi.org/10.1016/S2095-3119(12)60053-7, 2012.
Young, E. O., Ross, D. S., Cade-Menun, B. J., and Liu, C. W.: Phosphorus speciation in riparian soils: a phosphorus-31 nuclear magnetic resonance spectroscopy and enzyme hydrolysis study, Soil Sci. Soc. Am. J., 77, 1636–1647, https://doi.org/10.2136/sssaj2012.0313, 2013.
Yue, X., Zhang, J., Shi, A., Yao, S., and Zhang, B.: Manure substitution of mineral fertilizers increased functional stability through changing structure and physiology of microbial communities, Eur. J. Soil Biol., 77, 34–43, https://doi.org/10.1016/j.ejsobi.2016.10.002, 2016.
Zelezniak, A., Andrejev, S., Ponomarova, O., Mende, D. R., Bork, P., and Patil, K. R.: Metabolic dependencies drive species co-occurrence in diverse microbial communities, P. Natl. Acad. Sci. USA, 112, 6449–6454, https://doi.org/10.1073/pnas.1421834112, 2015.
Zeng, J., Liu, X., Song, L., Lin, X., Zhang, H., Shen, C., and Chu, H.: Nitrogen fertilization directly affects soil bacterial diversity and indirectly affects bacterial community composition, Soil Biol. Biochem., 92, 41–49, https://doi.org/10.1016/j.soilbio.2015.09.018, 2016.
Zhang, X., Zhang, R., Gao, J., Wang, X., Fan, F., Ma, X., Yin, H., Zhang, C., Feng, K., and Deng, Y.: Thirty-one years of rice-rice-green manure rotations shape the rhizosphere microbial community and enrich beneficial bacteria, Soil Biol. Biochem., 104, 208–217, https://doi.org/10.1016/j.soilbio.2016.10.023, 2017.
Zhou, H., Gao, Y., Jia, X., Wang, M., Ding, J., Cheng, L., Bao, F., and Wu, B.: Network analysis reveals the strengthening of microbial interaction in biological soil crust development in the Mu Us Sandy Land, Northwestern China, Soil Biol. Biochem., 144, 107782, https://doi.org/10.1016/j.soilbio.2020.107782, 2020.
Zin, N. A. and Badaluddin, N. A.: Biological functions of Trichoderma spp. for agriculture applications, Ann. Agr. Sci., 65, 168–178, https://doi.org/10.1016/j.aoas.2020.09.003, 2020.