the Creative Commons Attribution 4.0 License.
the Creative Commons Attribution 4.0 License.
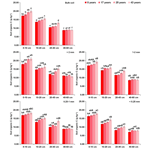
Soil nutrient contents and stoichiometry within aggregate size classes varied with tea plantation age and soil depth in southern Guangxi in China
Ling Mao
Shaoming Ye
Shengqiang Wang
Soil ecological stoichiometry offers a tool to explore the distribution, cycling, limitation, and balance of chemical elements in tea plantation ecosystems. This study aimed to explore how soil organic C (OC) and nutrient contents (total N (TN), total P (TP), Ca2+, Mg2+, Fe2+, and Mn2+) as well as their stoichiometric ratios (, , , , and ) varied with tea plantation age (8, 17, 25, and 43 years) and soil depth (0–10, 10–20, 20–40, and 40–60 cm) within aggregates in southern Guangxi in China. Our results showed that tea plantation age and soil depth significantly affected soil nutrient stoichiometry in different sizes of aggregates. Among different ages of tea plantations, soil OC, TN, and TP contents as well as , , and ratios significantly decreased as the soil depth increased. In addition, soil Ca2+ and Mg2+ contents were significantly lower in the surface soil layer than the deeper soil layer, whereas soil Fe2+ and Mn2+ contents showed opposite trends, and no significant differences were detected in and ratios among different soil depths. At the 0–40 cm soil depth, continuous planting of tea corresponded to increases in soil OC, TN, Fe2+, and Mn2+ contents, whereas soil Ca2+ and Mg2+ contents significantly decreased over time. During the process of tea growth, the losses of soil Ca2+ and Mg2+, especially Ca2+ (as indicated by the decrease in the soil ratio), led to soil acidification, which reduced Fe2+ absorption and enhanced Mn2+ uptake by tea plants (as indicated by the increase in the soil ratio). In general, tea plantation age affected the variations of soil nutrient contents and stoichiometry, and such effects were more obvious at the 0–40 cm soil depth, in contrast to the 40–60 cm soil depth.
- Article
(1816 KB) - Full-text XML
-
Supplement
(565 KB) - BibTeX
- EndNote
In the past century, under the remarkable increase in population pressure, continuous tillage and deforestation resulted in the dramatic decrease in soil fertility level in southern Guangxi in China (Jiang et al., 2018). To overcome these existing challenges, the Chinese government has rolled out the Grain for Green program in the hope of alleviating land deterioration by converting farmlands to forest lands or grasslands (Zeng et al., 2020). Since the initiation of this program, the southern part of Guangxi has begun transforming farmlands into tea (Camellia sinensis L.) plantations as per the local geography and natural resources (Zhang et al., 2017). Tea, as a pivotal cash crop, is commonly cultivated in the developing nations, particularly in China, India, Kenya, and Sri Lanka. China is the world's largest producer of tea, with the tea-planting area reaching 3.17×106 ha in 2020, and it shows an elevating trend in the future (Chinese Tea Committee, 2020). Guangxi has a subtropical monsoon climate and marks the key tea-planting region in China. According to the statistics from the Chinese Tea Committee (2020), over 80 % of tea plantations of Guangxi are situated in impoverished counties, and the tea-planting industry turns out to be a staple industry on which poor counties depend to throw off poverty.
Ecological stoichiometry offers a tool to explore the distribution, cycling, restriction, and balance of nutrients in terrestrial ecosystems (Yu et al., 2019) and is an invaluable tool for identifying the influencing factors and driving mechanisms in ecological processes (Su et al., 2019). Carbon (C) is the most commonly seen element in plants (Prescott et al., 2020), and nitrogen (N) and phosphorus (P) are critical control factors for the growth of plants (Krouk and Kiba, 2020). The relationships amongst C, N, and P are coupled (Elser et al., 2003), and their stoichiometric ratios (, , and ) reflect the nutrient status during the process of soil genesis, making them important indicators of soil quality (Bai et al., 2020). Additionally, calcium (Ca), magnesium (Mg), iron (Fe), and manganese (Mn) are pivotal metallic nutritive elements for the development of plants (H. Liu et al., 2021). The contents of soil total Ca, Mg, Fe, and Mn may exceed the demand of a single plant by more than 1000-fold and cannot sensitively reflect the needs of plants (Miner et al., 2018), but the contents of these nutrients' available fractions may be insufficient or redundant, resulting in the deficiencies or abundances of plant nutrients (Otero et al., 2013). Thus, soil exchangeable Ca and Mg, together with the available Fe and Mn, significantly affect the development of plants.
Over the past decade, soil nutrient stoichiometry (mainly C–N–P rather than Ca–Mg or Fe–Mn) has been broadly investigated across the world (Tian et al., 2010; Zhang et al., 2016; Yue et al., 2017; Yu et al., 2018; Qiao et al., 2020). It has been widely acknowledged amongst these studies that soil depth is vital for the regulation of soil nutrient stoichiometry. Several studies have identified the decreasing trend of soil organic C (OC), total N (TN), and total P (TP) contents with increasing soil depth (Yue et al., 2017; Yu et al., 2018; Qiao et al., 2020), whereas conflicting vertical patterns have been observed for soil , , and ratios. For instance, a decreasing trend of the and ratios was observed with increasing soil depth, according to the data of the second soil investigation in China (Tian et al., 2010). Nevertheless, a larger ratio in the deeper soil layer, not the surface soil layer, was identified in a Mollisol plain in northeastern China, because soil total C was measured and carbonates were observed in the deeper soil layer (Zhang et al., 2016). Moreover, the ratio showed no remarkable change with soil depth in an investigation of alpine grassland on the Qingzang Plateau (Yang et al., 2010). As shown above, inconsistent vertical patterns have been reported for the C–N–P stoichiometric ratios in different soil ecosystems.
As the basic unit of soil structure, soil aggregates are complex ensembles composed of primary particles and organic matter (OM) (Tisdall and Oades, 1982). According to the differences in binding agents, soil aggregates can be classified into microaggregates (<0.25 mm) and macroaggregates (>0.25 mm) (Tisdall and Oades, 1982). In general, persistent binding agents (including chemically stable OM and polyvalent metal cation complexes) contribute to the binding of primary particles into microaggregates (Six et al., 2004). In contrast, temporary binding agents (including fungal hyphae, plant roots, and polysaccharides) aggregate with microaggregates, which facilitates the formation of macroaggregates (Six et al., 2004). Soil aggregates with various sizes have different abilities in the supply and reserve of soil OC and nutrients. Thus, to improve the comprehension of the structure and function of soil ecosystems, more efforts should be made to observe the soil nutrient stoichiometry within aggregates (Xu et al., 2019; Cui et al., 2021). Recently, although lots of studies have reported the OC, TN, and TP distribution in different sizes of aggregates, these studies have shown different results. To be specific, some studies reveal the significant increases in the OC, TN, and TP contents with decreasing aggregate size (Sarker et al., 2018; Piazza et al., 2020), while other studies observe opposite trends (Lu et al., 2019; D. Liu et al., 2021). Further, the changes in soil OC, TN, and TP contents within aggregates have received great attention, while soil exchangeable alkaline-earth metals (i.e., Ca2+ and Mg2+) and available micronutrients (i.e., Fe2+ and Mn2+) are less often investigated.
Our previous studies indicated that the land-use shift from farmlands to tea plantations ameliorated the soil fertility level (Zheng et al., 2011). However, during the process of tea growth, the variation of soil nutrient stoichiometry remains unclear. Meanwhile, since tea serves as a deep-rooted plant, it is vital to understand how nutrient stoichiometry changes with the increasing soil depth in tea plantation ecosystems. Thus, this study was carried out to investigate how soil OC and nutrient contents as well as their stoichiometric ratios varied with tea plantation age (8, 17, 25, and 43 years) and soil depth (0–10, 10–20, 20–40, and 40–60 cm) within aggregates (<0.25, 0.25–1, 1–2, and >2 mm). We hypothesized that (i) soil OC and TN contents would increase with tea plantation age due to the annual fertilization and that (ii) decreases in soil Ca2+ and Mg2+ contents would be accompanied by increases in soil Fe2+ and Mn2+ contents because of soil acidification during the process of tea growth.
2.1 Experiment site
In January 2019, this study was completed at the Hengxian Agriculture Experiment Center of Guangxi University (altitude of 557–563 m and slope degree of 13–15∘). The climate at the experiment site is dominated by a subtropical monsoon climate, with the yearly average rainfall and temperature being 1304 mm and 21.6 ∘C, respectively. An exposed soil horizon occurs early in the Mesozoic, which gradually forms the Ultisols agrotype (IUSS Working Group, 2014). As early as the 1960s, due to the high economic value of tea, massive hectares of farmlands were developed into tea plantations in this region.
“Baimao tea” refers to a major cultivar in this area, and the ages of these tea plantations are distinct. Managed by different owners, tea plantations were both experimental trials (Guangxi University) and commercial plantings. In the tea-planting course, the tillage method was no tillage and tea-planting density was almost 6×104 plants ha−1. Herbicides were not applied, and yellow sticky boards were used to prohibit pests, because this color might attract pests and get them stuck on the boards. In addition, all the tea plants were subject to slight pruning in September each year.
An annual fertilizer regime in tea plantations was shown below. Both 0.65 Mg ha−1 complex fertilizer (granule, N–P2O5–K2O: 18 %-6 %-6 %) and 12 Mg ha−1 swine manure (slurry, N–P2O5–K2O: 0.54 %-0.48 %-0.36 %) were applied vertically below tree crown yearly in mid-November as the basal fertilizer at the surrounding region. Subsequently, the top dressing, applied to the site treated with replenished basal fertilizer, was replenished thrice per year. Both 1.2 Mg ha−1 complex fertilizer and 0.5 Mg ha−1 urea were applied onto the soil surface in mid-March, while 0.65 Mg ha−1 complex fertilizer and 0.3 Mg ha−1 urea were applied in late June and in early September.
2.2 Experiment design
In general, examining the same location persistently has been considered an effective approach to monitor the variations of soil with time (Sparling et al., 2003). Nevertheless, the challenges in long-time soil monitoring have made it urgent to develop the substitutional approaches to investigate the changes in soil over time, amongst which the most common approach is the “space-for-time” alternative (Zanella et al., 2018).
In this study, this approach was used to explore the variation of soil nutrient stoichiometry in a chronological sequence of tea plantations. In general, confounding factors existed in the spatial variations of soil, and hence the present study managed to mitigate such effects by choosing tea plantations, which were cultured with the same tea variety (Baimao tea) with different planting ages (8, 17, 25, and 43 years) and were located at the same unit associated with geomorphological status.
Each of the 4 tea plantation age groups was replicated in 5 locations for a total of 20 experimental units (Fig. 1). Separation amongst these units was completed with distances of >800 m between each other, thereby decreasing the spatial autocorrelation and avoiding the pseudo-replication. For every unit ( m2), a plot () was randomly established with a distance of >50 m away from the unit margin.
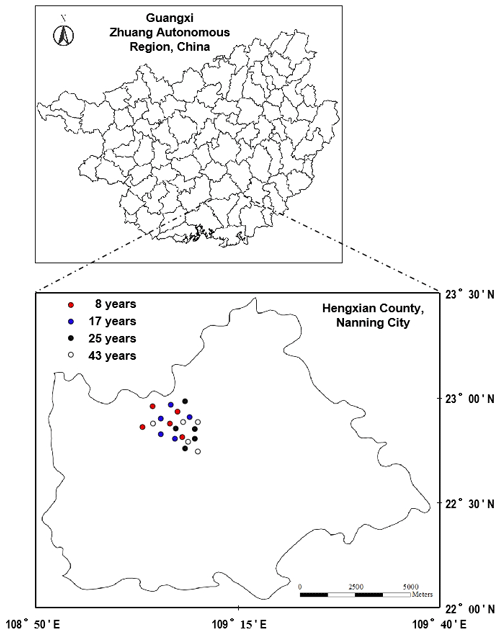
Figure 1Location of the experiment site. Tea plantations were cultured with the same tea variety with different planting ages (8, 17, 25, and 43 years) and were located at the same unit associated with the geomorphological status. Each of the 4 tea plantation age groups was replicated in 5 locations for a total of 20 experimental units.
2.3 Litter and soil sampling
For every plot, five surface litter (a stock) specimens were acquired from the surface of the soil in the five randomly chosen subplots (), which were afterwards integrated into a composite litter specimen. In total, 20 (4 tea plantation ages × 5 replicates) composite litter specimens were desiccated at 80 ∘C until the weight became constant. Then, the weights of these desiccated litter specimens were measured, and the litter C (Nelson and Sommers, 1996) and N (Bremner, 1996) contents were measured. The amounts of litter were 821, 974, 786, and 648 g m−2 in the 8, 17, 25, and 43 years of tea plantations, respectively, and the ratios of litter were 14.23, 12.68, 17.32, and 21.37, respectively.
Soil sampling was completed at the same sites of litter sampling. For every plot, five soil specimens were acquired by a spade from every soil layer (i.e., 0–10, 10–20, 20–40, and 40–60 cm) in the five subplots (), which were afterward integrated into a composite soil specimen. In total, 80 (4 tea plantation ages × 4 soil layers × 5 replicates) composite soil specimens were gently separated into the naturally formed aggregates and were then sieved by a 5 mm sifter to remove small stones, coarse roots, and macrofauna. Afterwards, soil specimens were used for the aggregate separation. For every plot, another five soil specimens were randomly chosen via cutting rings (, diameter=50.46 mm, and depth=50 mm) from every soil layer to measure the bulk density, clay (<0.002 mm), pH, OC, and nutrients of bulk soil.
2.4 Soil aggregate separation
According to the wet screening process, 250 g of every composite soil specimen was sieved via the 2, 1, and 0.25 mm sieves successively (Kemper and Chepil, 1965). To be specific, the composite soil specimens were soaked in distilled water for 15 min and were then shaken in the vertical direction for 15 min at the 1 s−1 oscillating rate and 5 cm amplitude. Consequently, we obtained four different sizes of aggregates, covering microaggregates (<0.25 mm) and fine (0.25–1 mm), medium (1–2 mm), and coarse (>2 mm) macroaggregates. All the aggregates were desiccated and weighed, and later the aggregate-related OC and nutrients were measured.
2.5 Soil property analyses
Prior to the analyses of soil physical–chemical properties, soil specimens were subject to atmospheric drying under indoor temperature conditions. According to the cutting ring method (Lu, 2000), soil specimens were oven-dried at 105 ∘C until constant weight so as to measure the bulk density. Soil clay was measured by the hydrometer (TM-85, Veichi, China) (Lu, 2000). Soil pH was measured by the glassy electrode (MT-5000, Ehsy, China), with the ratio of soil : water (mass : volume) being 1 : 2.5 (Lu, 2000). Soil OC and TN were measured via the acid dichromate wet oxidation method (Nelson and Sommers, 1996) and the micro-Kjeldahl method (Bremner, 1996), respectively. Soil TP was measured via the molybdate blue colorimetry method (Bray and Kurtz, 1945). Soil exchangeable alkaline-earth metals (i.e., Ca2+ and Mg2+) were measured by ammonium acetate (CH3COONH4) (Thomas, 1982). Briefly, 2.5 g of every aggregate fraction was weighed into the Erlenmeyer flask to blend with 50 mL 1 M CH3COONH4 (pH=7.0). The extract liquid was agitated for 30 min under 150 rpm and then sieved via Whatman No. 2 V filtration paper (quantitative and ash-free). Soil available micronutrients (i.e., Fe2+ and Mn2+) were measured by diethylenetriamine pentaacetic acid (DTPA) (Lindsay and Norvell, 1978). Briefly, 10 g of every aggregate fraction was weighed into the Erlenmeyer flask to blend with 20 mL 0.005 M DTPA + 0.01 M CaCl2 + 0.1 M TEA (triethanolamine) (pH=7.0). The extract liquid was agitated for 2 h under 180 rpm and then sieved. All the extractable metallic cations were measured by the atomic absorption spectrometer (AAS, Shimadzu, Japan). In this study, 5 standard specimens (GBW-07401), 5 blank specimens, and 80 analytical replicates (accounting for 20 % of the total soil specimens) were used to control quality. The difference between analytical replicates was consistently less than 5 %.
2.6 Calculations and statistics
The mean weight diameter (MWD, mm) was utilized to indicate the stability of soil aggregates. To be specific, the higher MWD value indicated the stronger aggregate stability (Kemper and Chepil, 1965):
In the formula, Xi indicates the ith size aggregates' mean diameter (mm) and Mi indicates the ith size aggregates' proportion (% in weight).
SPSS 22.0 software (SPSS, Inc., Chicago, IL, USA) was used for statistical analysis (Table 1). Means were tested by Tukey's HSD, and the significant level was set at p≤0.05. Two-way analysis of variance (ANOVA) was used for exploring the effects of soil depth, tea plantation age, and their interactions on the physicochemical properties of the bulk soil. Three-way ANOVA was used for exploring the effects of soil depth, tea plantation age, aggregate size, and their interactions on the physicochemical properties of soil aggregates. Besides that, Pearson correlation analysis was utilized to test the relationships between pH and stoichiometric ratios (i.e., and ) in bulk soil during the process of tea growth.
Table 1Three-way ANOVA regarding the effects of soil depth, tea plantation age, aggregate size, and their interactions on the physicochemical properties of soil aggregates and two-way ANOVA regarding the effects of soil depth, tea plantation age, and their interactions on the physicochemical properties of bulk soil.
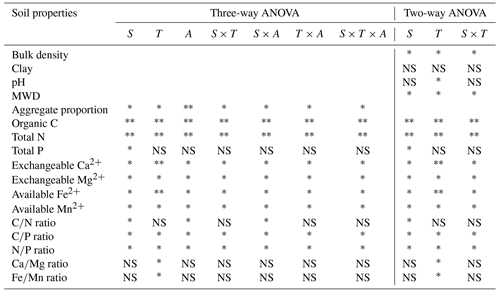
S: soil depth; T: tea plantation age; A: aggregate size. , ∗, and NS indicate significant differences at p<0.01, p≤0.05, and p>0.05 (not significant), respectively.
3.1 Soil bulk density, clay content, and pH
At the 0–10 and 10–20 cm soil depths, bulk density significantly decreased within the first 17 years and afterwards significantly increased, whereas the effect of tea plantation age on the bulk density is limiting at the 20–40 and 40–60 cm soil depths (Tables 1 and 2). Regardless of the tea plantation age, a significant increase in bulk density was observed as the soil depth increased. A two-way ANOVA showed that the effects of soil depth, tea plantation age, and their interactions on the clay content were not significant (Tables 1 and 2). Soil pH significantly decreased during the process of tea growth (Tables 1 and 2). Moreover, no significant variation in soil pH was observed with the increasing soil depth.
Table 2Effects of soil depth and tea plantation age on the bulk density, clay, and pH in bulk soil.
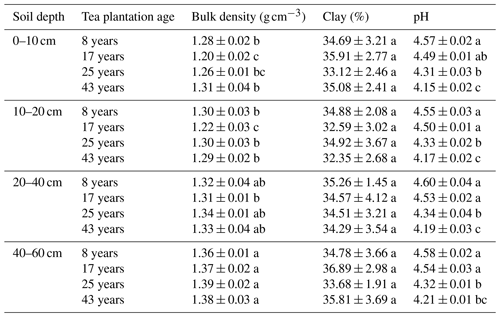
Data represent the mean of five replicates ± standard deviations. Means in the same column with the same lowercase letter are not significantly different (p>0.05) among different soil depths and tea plantation ages.
3.2 Composition and stability of soil aggregates
At the 0–10 and 10–20 cm soil depths, continuous planting of tea resulted in significant variations in the proportions of different sizes of aggregates, apart from the medium and fine macroaggregates (Table 3). To be specific, the proportions of coarse macroaggregates significantly rose within the first 17 years and then significantly dropped, whereas the proportions of microaggregates displayed an opposite trend over time. At the same time, the greatest value of soil MWD was identified in the tea plantations of 17 years (Table 3). Notably, the role of tea plantation age in the aggregate composition and stability is limited at the 20–40 and 40–60 cm soil depths. Across the four tea plantation ages, the coarse macroaggregates were dominant at the 0–10 cm soil depth, accounting for 32.60 %–53.18 % of bulk soil. However, at the 10–20, 20–40, and 40–60 cm soil depths, the microaggregates were dominant, accounting for 33.80 %–49.51 %, 42.12 %–48.24 %, and 44.80 %–49.45 %, respectively. According to the obtained results, the coarse macroaggregate proportions significantly decreased, while the microaggregate proportions significantly increased with the increasing soil depth.
Table 3Effects of soil depth and tea plantation age on the aggregate stability and composition.
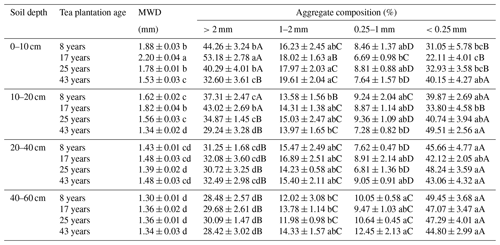
Data represent the mean of five replicates ± standard deviations. Means in the same column with the same lowercase letter are not significantly different (p>0.05) among different soil depths and tea plantation ages. Means in the same row with the same capital letter are not significantly different (p>0.05) among different-sized aggregates.
3.3 Contents of soil C, N, and P
Within aggregate size classes, soil OC (Fig. 2) and TN (Fig. 3) contents significantly increased with the increasing aggregate size, while the distribution of soil TP (Fig. 4) was even in different sizes of aggregates. From 8 to 43 years of tea plantations, the OC and TN contents in soil aggregates were significantly elevated by 22 %–35 % and 14 %–24 %, 11 %–22 % and 9 %–17 %, and 8 %–18 % and 9 %–13 % at the 0–10, 10–20, and 20–40 cm soil depths, respectively. Nevertheless, there existed no significant variation in the aggregate-related TP content. Furthermore, at the 40–60 cm soil depth, the aggregate-related OC, TN, and TP contents did not exhibit significant variations over time. Regardless of the tea plantation age, significant decreases in the aggregate-related OC, TN, and TP contents were observed as the soil depth increased.
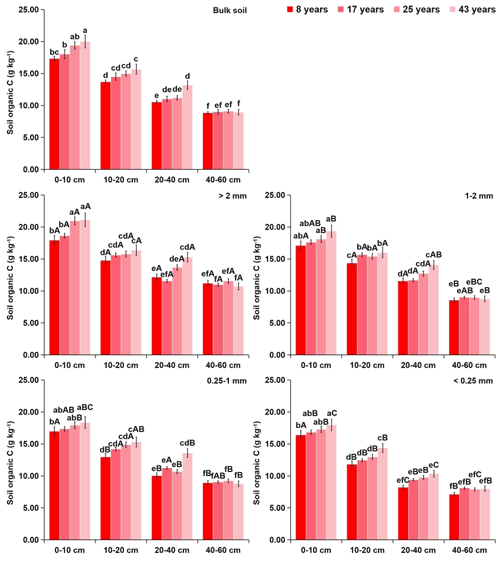
Figure 2Effects of soil depth and tea plantation age on the organic C content in bulk soil and different-sized aggregates. Data represent the mean of five replicates and error bars represent the standard deviations. Means with the same lowercase letter are not significantly different (p>0.05) among different soil depths and tea plantation ages. Means with the same capital letter are not significantly different (p>0.05) among different-sized aggregates.
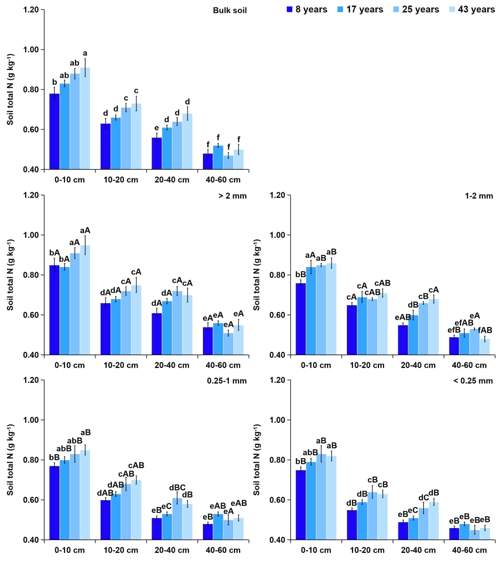
Figure 3Effects of soil depth and tea plantation age on the total N content in bulk soil and different-sized aggregates. Data represent the mean of five replicates, and error bars represent the standard deviations. Means with the same lowercase letter are not significantly different (p>0.05) among different soil depths and tea plantation ages. Means with the same capital letter are not significantly different (p>0.05) among different-sized aggregates.
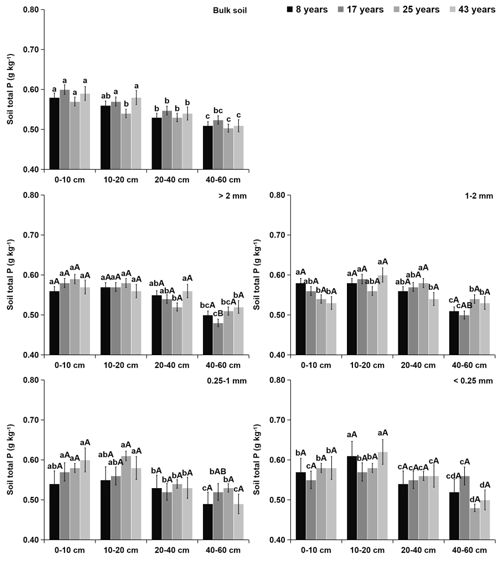
Figure 4Effects of soil depth and tea plantation age on the total P content in bulk soil and different-sized aggregates. Data represent the mean of five replicates, and error bars represent the standard deviations. Means with the same lowercase letter are not significantly different (p>0.05) among different soil depths and tea plantation ages. Means with the same capital letter are not significantly different (p>0.05) among different-sized aggregates.
3.4 Stoichiometric ratios of soil C, N, and P
A three-way ANOVA revealed that the lone and interactive effects of soil depth, tea plantation age, and aggregate size on the and rations were significant, and the effects of soil depth, aggregate size, and their interactions on the ratio were significant (Table 1). In this study, significant increases in aggregate-related (Table S1 in the Supplement), (Table S2 in the Supplement), and (Table S3 in the Supplement) ratios were accompanied by the increasing aggregate size. At the 0–10, 10–20, and 20–40 cm soil depths, the aggregate-related ratio did not exhibit significant variation, while aggregate-related and ratios significantly increased with increasing tea plantation age. Moreover, there was little role of tea plantation age in the aggregate-related , , and ratios at the 40–60 cm soil depth. Among different ages of tea plantations, aggregate-related , , and ratios significantly dropped as the soil depth increased. For example, at the 0–10 cm soil depth, aggregate-related , , and ratios across the four tea plantation ages fluctuated at 20.81–23.04, 28.81–37.07, and 1.31–1.67, respectively. In the meanwhile, at the 40–60 cm soil depth, aggregate-related , , and ratios fluctuated at 16.41–20.74, 13.44–22.88, and 0.84–1.08, respectively.
3.5 Contents of soil alkaline-earth metals and micronutrients
Within aggregate size classes, soil exchangeable alkaline-earth metals (i.e., Ca2+ and Mg2+) were more concentrated in the microaggregates (Figs. 5 and 6). However, soil available micronutrients (i.e., Fe2+ and Mn2+) were mainly found in the coarse macroaggregates (Figs. 7 and 8). From 8 to 43 years of tea plantations, the Ca2+ and Mg2+ contents in soil aggregates were significantly reduced by 31 %–38 % and 10 %–24 %, 23 %–27 % and 9 %–18 %, and 10 %–16 % and 5 %–8 % at the 0–10, 10–20, and 20–40 cm soil depths, respectively. From 8 to 43 years of tea plantations, however, the Fe2+ and Mn2+ contents in soil aggregates were significantly elevated by 16 %–27 % and 6 %–9 %, 11 %–15 % and 4 %–7 %, and 7 %–12 % and 3 %–5 %, respectively. In addition, at the 40–60 cm soil depth, the contents of aggregate-related exchangeable alkaline-earth metals and available micronutrients did not present significant variations over time. Irrespective of the tea plantation age, significant increases in the aggregate-related Ca2+ and Mg2+ contents were observed with the increasing soil depth, whereas the aggregate-related Fe2+ and Mn2+ contents showed an opposite trend.
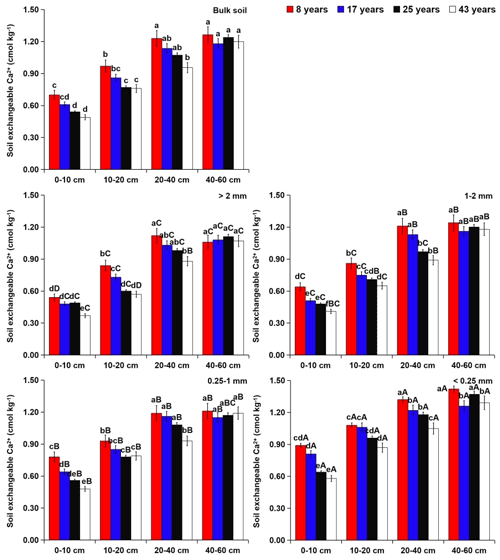
Figure 5Effects of soil depth and tea plantation age on the exchangeable Ca2+ content in bulk soil and different-sized aggregates. Data represent the mean of five replicates, and error bars represent the standard deviations. Means with the same lowercase letter are not significantly different (p>0.05) among different soil depths and tea plantation ages. Means with the same capital letter are not significantly different (p>0.05) among different-sized aggregates.
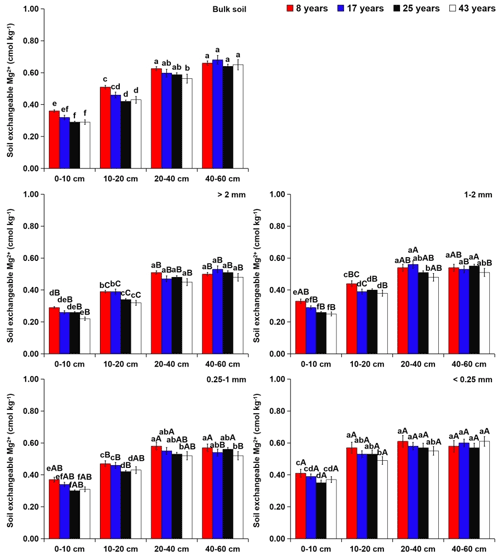
Figure 6Effects of soil depth and tea plantation age on the exchangeable Mg2+ content in bulk soil and different-sized aggregates. Data represent the mean of five replicates, and error bars represent the standard deviations. Means with the same lowercase letter are not significantly different (p>0.05) among different soil depths and tea plantation ages. Means with the same capital letter are not significantly different (p>0.05) among different-sized aggregates.
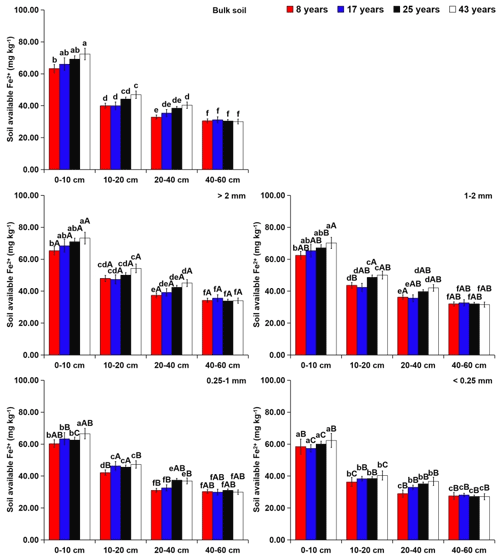
Figure 7Effects of soil depth and tea plantation age on the available Fe2+ content in bulk soil and different-sized aggregates. Data represent the mean of five replicates, and error bars represent the standard deviations. Means with the same lowercase letter are not significantly different (p>0.05) among different soil depths and tea plantation ages. Means with the same capital letter are not significantly different (p>0.05) among different-sized aggregates.
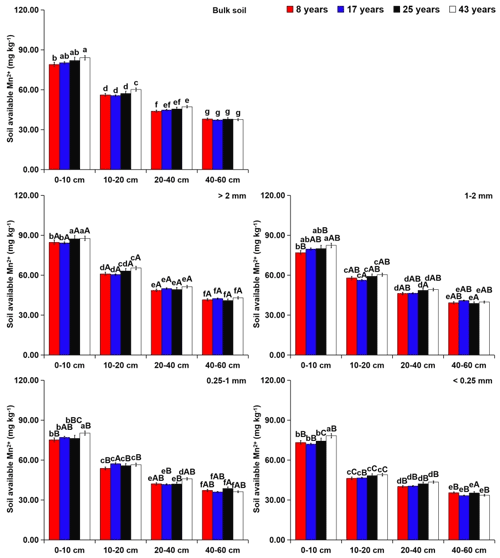
Figure 8Effects of soil depth and tea plantation age on the available Mn2+ content in bulk soil and different-sized aggregates. Data represent the mean of five replicates, and error bars represent the standard deviations. Means with the same lowercase letter are not significantly different (p>0.05) among different soil depths and tea plantation ages. Means with the same capital letter are not significantly different (p>0.05) among different-sized aggregates.
3.6 Stoichiometric ratios of soil alkaline-earth metals and micronutrients
A three-way ANOVA demonstrated that the effect of tea plantation age on the and ratios in soil aggregates was significant (Table 1). In this study, soil (Table S4 in the Supplement) and (Table S5 in the Supplement) ratios did not vary among different sizes of aggregates. At the 0–10, 10–20, and 20–40 cm soil depths, the aggregate-related ratio significantly decreased, while the aggregate-related ratio significantly increased in the tea-planting course. Moreover, there was little role for tea plantation age in the aggregate-related and ratios at the 40–60 cm soil depth. In tea plantations, no significant variations were observed amongst different soil depths in aggregate-related and ratios. For example, at the 0–10 cm soil depth, aggregate-related and ratios across the four tea plantation ages ranged from 1.81 to 1.96 and from 0.76 to 0.85, respectively. Meanwhile, at the 40–60 cm soil depth, aggregate-related and ratios ranged from 1.88 to 1.92 and from 0.78 to 0.82, respectively.
4.1 Composition and stability of soil aggregates
Tea plantation age significantly influenced the aggregate composition and stability at the 0–10 and 10–20 cm soil depths, whereas the effect at the 20–40 and 40–60 cm soil depths was extremely limited. In the early (8–17-year) period, tea planting was conducive to the transition from microaggregates to coarse macroaggregates at the 0–10 and 10–20 cm soil depths (Table 3). Comparatively, in the middle (17–25-year) and late (25–43-year) periods, tea planting induced coarse macroaggregate destruction and microaggregate release (Table 3). According to the hierarchical concept of soil aggregates (Six et al., 2004), the quality of plant litter returning to the soil determines the distribution of decomposition products of litter in different sizes of aggregates, ultimately impacting the aggregate composition. In the early period of tea planting, tea litter displayed greater availability (as indicated by the lower litter ratio), revealing that the decomposition products of litter were easily combined into the coarse macroaggregates, thereby fostering the formation of coarse macroaggregates (Tisdall and Oades, 1982). Reversely, in the middle and late periods of tea planting, tea plants naturally encountered aging processes and litter was progressively subjected to decomposition, inducing the decomposition of coarse macroaggregates into microaggregates (Six and Paustian, 2014). Moreover, the reduced litter amount and covering area after 17 years of tea planting enhanced the rainfall eluviation and artificial interferences (i.e., pruning of tea plants and application of fertilizers), which could also cause the destruction of coarse macroaggregates. In the tea-planting course, variation in aggregate stability was indicated by the change in MWD value (Table 3). At the 0–10 and 10–20 cm soil depths, the MWD value was the greatest in the 17 years of tea planting, which was associated with the highest proportions of coarse macroaggregates in the 17-year tea plantations. The above findings indicated that the 17-year tea plantations exhibited stronger aggregate stability in contrast to other plantations at the 0–10 and 10–20 cm soil depths.
Regardless of the tea plantation age, coarse macroaggregates were dominant in the topsoil (0–10 cm), while microaggregates were dominant in the subsoil (10–60 cm), suggesting transformation of aggregate composition from coarse macroaggregate-prevailing to microaggregate-prevailing with increasing soil depth (Table 3). In addition, similar outcomes were corroborated by Li et al. (2015) and Zhu et al. (2017) from studies on tea plantations in southwestern Sichuan in China. In this study, coarse macroaggregates were the prevailing fractions in the topsoil, not the subsoil, which was caused by the surface cumulation of soil OC (Fig. 2). As an essential cementing agent, soil OC could foster the formation of coarse macroaggregates (Al-Kaisi et al., 2014). Moreover, the reduced proportions of coarse macroaggregates as the soil depth increased also resulted from the elevated soil compactness (as indicated by the bulk density) (Table 2). Soil densification could prevent the growth of plant roots, hence causing the activities of soil microorganisms to decrease, especially soil fungi (Kurmi et al., 2020). Reduced activities of soil fungi could diminish the production of polysaccharose and glomalin-related soil protein (GRSP) from the fungal hyphae, thereby causing the proportions of soil macroaggregates to decrease (Ji et al., 2019). Similarly, as per our past studies (S. Wang et al., 2017; Zhu et al., 2019), soil microbial activities and GRSP content served as the vital effects in the formation and stabilization of soil macroaggregates and also presented the higher levels in the topsoil compared with the subsoil in tea plantation ecosystems. With the increasing soil depth, the decrease in MWD value was mainly associated with the change in soil aggregate composition (Table 3), especially for the decomposition of coarse macroaggregates into microaggregates, implying that the topsoil exhibited stronger aggregate stability, in contrast to the subsoil.
4.2 Contents of soil C, N, and P
In this study, more contents of soil OC and TN could be detected in coarse macroaggregates (Figs. 2 and 3), which conformed to the findings of Six et al. (2004) that macroaggregates were comprised of microaggregates via temporary binding agents. Meanwhile, macroaggregates could provide the protection for the OM, causing the cumulation of OC and TN in macroaggregates. Different from soil OC and TN, soil TP was evenly distributed in different sizes of aggregates (Fig. 4). Moreover, Bhatnagar and Miller (1985) also detected similar outcomes from soil specimens subjected to fresh poultry manure treatments and promoted the mechanisms influencing the distribution of TP in soil aggregates. Specifically, (i) introduced P firstly adsorbed by clay particulates in soil and clay particulates were discrepant in different sizes of aggregates, and (ii) introduced P had selective absorptive properties for the different sizes of aggregates. According to our findings, stochasticity seems to be one probable mechanism that sheds light on the TP distribution in soil aggregates.
Tea plantation age could positively affect the cumulation of soil OC and TN, while such positive effects were more obvious at the 0–40 cm soil depth, in contrast to the 40–60 cm soil depth. In this study, soil OC and TN contents exhibited a significant growing trend over time (Figs. 2 and 3), which was possibly associated with the following mechanisms. At first, numerous long-period tests demonstrated the proactive roles of manure and chemical fertilizer applications in soil OM cumulation (Tong et al., 2009; Zhou et al., 2013). Similarly, in the tea-planting course, growing soil OC and TN contents were probably caused by the application of substantial swine manure every year (12 ) in this tea-planting region (Wang and Ye, 2020). Second, plants serve as the prime OM sources in soil via root exudates and litter remains (Franklin et al., 2020). In the tea-planting course, soil OC and TN cumulation probably occurred as a result of the growing root systems and the increasing amounts of aboveground litter attained from trimmed branches and leaves. Third, no tillage could provide physical protection for the OM combined with soil aggregates, then further improving soil OC and TN sequestration (Wulanningtyas et al., 2021). Notably, although the positive correlations of OC and TN contents with clay content in soil have been reported, this study revealed that significant increases in the OC and TN contents were accompanied by no significant variation in the clay content during the process of tea growth (Table 2). Similarly, Li et al. (2015) and Wang et al. (2018) also discovered that the changes in soil OC and TN contents were not influenced by the clay content over time in tea plantation ecosystems, mainly because soil OC and TN contents primarily depend on fertilization, tillage, root exudates, and litter remains, whereas soil clay content is mainly controlled by its parent material (Rakhsh et al., 2020). Different from soil OC and TN, regardless of the soil depth, there existed no significant difference in soil TP content amongst differently aged tea plantations (Fig. 4), implying the resistance of soil TP content to the change in tea plantation age. Moreover, previous studies verified that soil TP content was not associated with the tea plantation age (Wu et al., 2018; Yan et al., 2018), as soil P primarily derives from the weathering release of soil minerals, instead of the short-period biology cycle (Cui et al., 2019). In tea plantation ecosystems, the decreasing OC, TN, and TP contents with the increasing soil depth (Figs. 2, 3, and 4) coincided with some previous findings in other ecosystems, including tropic forests, bushlands, and grasslands (Stone and Plante, 2014; Yu et al., 2018; Qiao et al., 2020). In this study, the higher contents of OC, TN, and TP in the topsoil were associated with the higher OM input, where the soil OM content in the topsoil was enriched by the input of surface tea litter, root debris and exudates, and swine manure.
4.3 Stoichiometric ratios of soil C, N, and P
Soil , , and ratios serve as vital indicators of soil health (Liu et al., 2018), which can be employed to explore C circulation and guide the equilibrium between N and P in soil ecosystems (Sardans et al., 2012). In this study, the soil ratio grew with increasing aggregate size (Table S1), indicating that the OM in macroaggregates was younger and more unstable, in contrast to microaggregates (Six et al., 2004). Meanwhile, the OM associated with microaggregates experienced more degradation, resulting in the lower ratio in the microaggregates (Xu et al., 2019). Among different ages of tea plantations, soil OC and TN were predominantly distributed in the coarse macroaggregates, whereas the TP was evenly distributed in different sizes of aggregates. As a result, the associations of and ratios to aggregate size primarily depended on the relationships of OC and TN contents with aggregate size (Tables S2 and S3). As far as we know, the changes in soil and ratios within aggregates are rarely examined, even though these kinds of knowledge are imperative due to the biogeochemical cycles of N and P being influenced by the dynamics of soil aggregates (Cui et al., 2021). Consequently, the impact generated by the aggregate size on the and ratios is required to be studied more for the accurate forecast of soil N and P cycling under natural or human-intervened ecosystems.
Irrespective of the soil depth, the soil ratio showed little significant variation in the tea-planting course (Table S1). Meanwhile, tea plantation age significantly affected soil and ratios at the 0–40 cm soil depth rather than the 40–60 cm soil depth (Tables S2 and S3). The soil ratio is generally treated as the critical indicator which can affect the formation and degradation of soil OM (Khan et al., 2016). Since the response of the soil TN content to soil environment change is almost the same as the soil OC content (Wang et al., 2018), the soil ratio did not present significant differences amongst differently aged tea plantations (Table S1). Similarly, Zhou et al. (2018) proved that no close correlation existed between soil ratio and vegetation coverage, because C and N are structure elements and their cumulation and consumption in soil remain relatively consistent. The soil ratio is the indicator suggesting P effectiveness, and a higher ratio often denotes lower P effectiveness (Khan et al., 2016). In acidic soil (Table 2), available P was adsorbed on the surfaces of oxides and clay minerals in a preferential way, because oxides and clay minerals with greater surface areas could afford enough sites available P adsorption (Wu et al., 2018). Therefore, as the tea plantation age increased, soil acidification generated the decrease in P effectiveness (evidenced by the significant increase in the soil ratio) (Table S2). Soil N and P are the prohibiting factors mostly observed during the process of plant growth, and thus the ratio can be utilized as an efficient indicator that shows nutrient restriction (Khan et al., 2016). In this study, the soil ratio significantly increased in the tea-planting course (Table S3), mainly because soil TN content experienced a significant increase, while no such significant change was observed in TP content over time.
Regardless of the tea plantation age, the soil ratio decreased with the increasing soil depth (Table S1), which coincided with the results from Cao et al. (2015), Feng and Bao (2017), and Yu et al. (2019). They suggested that the decrease in the soil ratio as the soil depth increased was triggered by the older and more processed OM in the deeper soil layer. Moreover, in this study, the lower soil and ratios in the subsoil (Tables S2 and S3) backed the outcomes of past studies in terrestrial ecosystems in China, which were on the foundation of the data obtained from both the second soil investigation in China (Tian et al., 2010) and the Chinese Ecosystem Research Network (CERN) (Chai et al., 2015).
Across the four tea plantation ages, the mean contents of OC and TN in bulk soil (0–20 cm) were 16.70 and 0.77 g kg−1, respectively, which were below the mean contents of OC (21.30 g kg−1) and TN (2.17 g kg−1) in Chinese tea plantations (Sun et al., 2020; Xie et al., 2020). Moreover, in this tea-planting region, the mean content of TP in bulk soil (0–20 cm) was 0.57 g kg−1, corresponding to the moderate level in Chinese tea plantations, where TP content varied in the range of 0.35–1.20 g kg−1 (Wu et al., 2018; Sun et al., 2020). Herein, the soil ratio is higher compared with other tea-planting regions in China, whereas soil and ratios are much lower (Sun et al., 2020). The above findings are primarily associated with the lower contents of soil OC and TN, especially TN. In general, N is the most limiting element in the net primary production of tea plantation ecosystems (Miner et al., 2018). In addition, this phenomenon also appeared in southern Guangxi in China.
4.4 Contents of soil alkaline-earth metals and micronutrients
According to the findings from Adesodun et al. (2007) and Emadi et al. (2009), the higher contents of exchangeable alkaline-earth metals (including Ca2+ and Mg2+) were detected in both 2–4.76 and <0.25 mm aggregates in the non-tillage soil. However, in the tillage course, the contents of these two cations decreased in the 2–4.76 mm aggregates and increased in the <0.25 mm aggregates, revealing that the tillage practice could lead soil Ca2+ and Mg2+ to redistribute in different sizes of aggregates. Comparatively, this study exhibited that the distribution of soil Ca2+ and Mg2+ in aggregates was similar among different ages of tea plantations (Figs. 5 and 6), suggesting that the distribution of these two cations in aggregates was seldom influenced by the tea plantation age. Specifically, coarse macroaggregates had the lowest contents of Ca2+ and Mg2+, whereas microaggregates exhibited the highest contents. These findings could be ascribed to the larger specific surface areas of microaggregates (Adesodun et al., 2007), which increased microaggregates' adsorption to Ca2+ and Mg2+ derived from root exudates, litter remains, and manure (Emadi et al., 2009). Different from exchangeable alkaline-earth metals, the contents of soil available micronutrients (including Fe2+ and Mn2+) usually correspond to the content of soil OM (R. Wang et al., 2017) and are more abundant in macroaggregates (Six et al., 2004). Moreover, this study also found that the Fe2+ and Mn2+ had a similar distribution pattern to OC within aggregates (Figs. 7 and 8). Since the decomposition products of litter can be easily integrated into the coarse macroaggregates (Six et al., 2004), the nutrient cycling of plant–soil systems might contribute to the higher contents of soil Fe2+ and Mn2+ in the coarse macroaggregates (R. Wang et al., 2017).
At the 0–40 cm soil depth, the contents of soil Ca2+ and Mg2+ significantly decreased over time (Figs. 5 and 6), which might be caused by the applications of urea and –N fertilizer in the tea-planting course for increasing tea leaf outputs. Urea hydrolysis can promote the production of ammonium ions which are readily nitrified into nitrate, and the excessive proton produced by the nitrification can compete for the adsorption sites with Ca2+ and Mg2+ (R. Wang et al., 2017). As a result, these cations were easy to lose from soil in the manner of leaching. Except at the 40–60 cm soil depth, continuous planting of tea generated the significant increases in soil Fe2+ and Mn2+ contents (Figs. 7 and 8), which were increased by 7 %–27 % and 3 %–9 % from 8 to 43 years of tea planting, respectively. This phenomenon was possibly caused by the soil acidification (Table 2), stimulating the release of soil Fe2+ and Mn2+ by mineralization and desorption from soil OM and minerals (R. Wang et al., 2017). Tea, as an aluminum (Al)-cumulating crop, is capable of cumulating Al in leaves (Li et al., 2016). Soil acidification in the tea-planting course was due to the substantial tea litter into the soil annually through trimmed branches and leaves (Li et al., 2016). At the same time, the rhizosphere deposition of massive organic acids (i.e., malate, lemon acid, and oxalate acid) around the tea roots could provoke localized acidification (Xue et al., 2006). Apart from that, to increase the output of tea, tea plantations needed to apply N fertilizers (i.e., urea and –N), thus leading to soil acidification by the nitration (Yang et al., 2018). Across the four tea plantation ages, the contents of soil Fe2+ and Mn2+ were higher in the topsoil than the subsoil (Figs. 7 and 8), primarily due to the usage of swine manure and the inputs of tea litter and roots in the topsoil (Miner et al., 2018). Nevertheless, the contents of soil Ca2+ and Mg2+ showed an opposite trend as the soil depth increased (Figs. 5 and 6), because soil Ca2+ and Mg2+ were easy to move from topsoil to subsoil in the manner of leaching (Hansen et al., 2017).
4.5 Stoichiometric ratios of soil alkaline-earth metals and micronutrients
Tea plantation age exerted a significant influence on the and ratios at the 0–40 cm soil depth rather than the 40–60 cm soil depth (Tables S4 and S5). To be specific, a significant decline in the ratio was found at the 0–40 cm soil depth over time. From 8 to 43 years of tea planting, the contents of Ca2+ and Mg2+ at the 0–40 cm soil depth decreased by 10 %–38 % and 5 %–24 %, respectively, revealing that the role of tea plantation age in the content of soil Ca2+ was greater than that of soil Mg2+ (Figs. 5 and 6). Lu et al. (2014) suggested that the selective losses of soil exchangeable alkaline-earth metals () could lead to the disequilibrium of soil metal ions in forest ecosystems. Similarly, in this study, the preferential loss of soil Ca2+ relative to Mg2+ was the prime reason for the significant decline in the soil ratio in the tea-planting course. The depletion of soil exchangeable alkaline-earth metals (especially Ca2+) could generate the decrease in soil-buffering capacity and soil acidification (Hansen et al., 2017). Thus, the ratio at the 0–40 cm soil depth was positively related (p≤0.05) to soil pH across the four tea plantation ages (Fig. S1 in the Supplement). Soil acidification accelerated the mineralization and desorption of soil available micronutrients from soil OM and minerals (R. Wang et al., 2017), which was conducive to the significant increases in Fe2+ and Mn2+ contents at the 0–40 cm soil depth, especially Fe2+ (Figs. 7 and 8). In a chronological sequence of tea plantations, the negative relationship (p≤0.05) of the soil ratio with soil pH at different soil depths indicated more cumulation of soil Fe2+ relative to Mn2+ over time (Fig. S1). Furthermore, during the process of tea plant uptake, the change in the soil ratio was also triggered by the antagonistic relationship between soil Fe2+ and Mn2+ (R. Wang et al., 2017). Tian et al. (2016) discovered that soil acidification could reduce Fe2+ absorption and enhance Mn2+ uptake by various plant species, causing the increase in the soil ratio and threatening plant productivity.
To conclude, soil OC, TN, and TP contents as well as , , and ratios decreased as the soil depth increased. Moreover, soil Ca2+ and Mg2+ contents were lower in the topsoil than the subsoil, whereas soil Fe2+ and Mn2+ contents showed an opposite trend, and no differences were detected amongst different soil depths in soil and ratios. At the 0–40 cm soil depth, continuous planting of tea was favorable to the increases in soil OC, TN, Fe2+, and Mn2+ contents, whereas soil Ca2+ and Mg2+ contents decreased over time, thus supporting our hypotheses. Compared with other tea-planting regions in China, the soil ratio is higher in this tea-planting region, whereas soil and ratios are much lower, suggesting that soil OC and TN contents in this study were lower, especially TN. In the tea-planting course, the losses of soil Ca2+ and Mg2+, especially Ca2+ (as indicated by the decrease in the soil ratio), could lead to the soil acidification. Meanwhile, soil acidification could reduce Fe2+ absorption and enhance Mn2+ uptake by tea plants (as indicated by the increase in the soil ratio). In general, tea plantation age could influence the variations in soil nutrient contents and stoichiometry, whereas such effects were more obvious at the 0–40 cm soil depth, in contrast to the 40–60 cm soil depth.
The data supporting the discovered information here can be presented by the relevant author based on reasonable requests.
The supplement related to this article is available online at: https://doi.org/10.5194/soil-8-487-2022-supplement.
SW and SY designed the experiments. LM carried out the experiments. SW and LM analyzed the experimental results. LM, SW, and SY wrote and edited the manuscript.
The contact author has declared that none of the authors has any competing interests.
Publisher's note: Copernicus Publications remains neutral with regard to jurisdictional claims in published maps and institutional affiliations.
The authors would like to express their gratitude to the editor and innominate referees for giving constructive advice and optimizing this article.
This study was funded by the Natural Science Foundation of Guangxi (no. 2019GXNSFBA185012), the Innovation Project of Guangxi Graduate Education (no. YCSW2022114), and the Cooperation Project of Dagui Mountain Forest Farm (no. 202200100).
This paper was edited by Jocelyn Lavallee and reviewed by two anonymous referees.
Adesodun, J. K., Adeyemi, E. F., and Oyegoke, C. O.: Distribution of nutrient elements within water-stable aggregates of two tropical agro-ecological soils under different land uses, Soil Till. Res., 92, 190–197, https://doi.org/10.1016/j.still.2006.03.003, 2007.
Al-Kaisi, M. M., Douelle, A., and Kwaw-Mensah, D.: Soil microaggregate and macroaggregate decay over time and soil carbon change as influenced by different tillage systems, J. Soil Water Conserv., 69, 574–580, https://doi.org/10.2489/jswc.69.6.574, 2014.
Bai, Y., Chen, S., Shi, S., Qi, M., Liu, X., Wang, H., Wang, Y., and Jiang, C.: Effects of different management approaches on the stoichiometric characteristics of soil C, N, and P in a mature Chinese fir plantation, Sci. Total Environ., 723, 137868, https://doi.org/10.1016/j.scitotenv.2020.137868, 2020.
Bhatnagar, V. K. and Miller, M. H.: Sorption of carbon and phosphorus from liquid poultry manure by soil aggregates of differing size, Can. J. Soil Sci., 65, 467–473, https://doi.org/10.4141/cjss85-050, 1985.
Bray, R. H. and Kurtz, L. T.: Determination of total, organic, and available forms of phosphorus in soils, Soil Sci., 59, 39–46, https://doi.org/10.1097/00010694-194501000-00006, 1945.
Bremner, J. M.: Nitrogen-total, Part 3, edited by: Sparks, D. L., American Society of Agronomy, 1085–1121, 1996.
Cao, J., Yan, W., Xiang, W., Chen, X., and Lei, P.: Stoichiometry characterization of soil C, N, and P of Chinese fir plantations at three different ages in Huitong, Hunan Province, China, Sci. Silvae Sin., 51, 1–8, http://www.linyekexue.net/CN/10.11707/j.1001-7488.20150701 (last access: 22 July 2022), 2015.
Chai, H., Yu, G., He, N., Wen, D., Li, J., and Fang, J.: Vertical distribution of soil carbon, nitrogen, and phosphorus in typical Chinese terrestrial ecosystems, Chinese Geogr. Sci., 25, 549–560, https://doi.org/10.1007/s11769-015-0756-z, 2015.
Chinese Tea Committee: An area planted with tea in various provinces at the end of each year, Annu. Bull. Statistics, 47, 20, 2020.
Cui, H., Ou, Y., Wang, L., Wu, H., Yan, B., and Li, Y.: Distribution and release of phosphorus fractions associated with soil aggregate structure in restored wetlands, Chemosphere, 223, 319–329, https://doi.org/10.1016/j.chemosphere.2019.02.046, 2019.
Cui, H., Ou, Y., Wang, L., Liang, A., Yan, B., and Li, Y.: Dynamic changes in microbial communities and nutrient stoichiometry associated with soil aggregate structure in restored wetlands, Catena, 197, 104984, https://doi.org/10.1016/j.catena.2020.104984, 2021.
Elser, J. J., Acharya, K., Kyle, M., Cotner, J., Makino, W., Markow, T., Watts, T., Hobbie, S., Fagan, W., Schade, J., Hood, J., and Sterner, R. W.: Growth rate-stoichiometry coupling in diverse biota, Ecol. Lett., 6, 936–943, https://doi.org/10.1046/j.1461-0248.2003.00518.x, 2003.
Emadi, M., Baghernejad, M., and Memarian, H. R.: Effect of land-use change on soil fertility characteristics within water-stable aggregates of two cultivated soils in northern Iran, Land Use Policy, 26, 452–457, https://doi.org/10.1016/j.landusepol.2008.06.001, 2009.
Feng, D. and Bao, W.: Review of the temporal and spatial patterns of soil stoichiometry and its driving factors, Chinese J. Appl. Environ. Biol., 23, 400–408, http://www.cibj.com/oa/DArticle.aspx?type=view&id=DG201604018 (last access: 22 July 2022), 2017.
Franklin, H. M., Carroll, A. R., Chen, C., Maxwell, P., and Burford, M. A.: Plant source and soil interact to determine characteristics of dissolved organic matter leached into waterways from riparian leaf litter, Sci. Total Environ., 703, 134530, https://doi.org/10.1016/j.scitotenv.2019.134530, 2020.
Hansen, M., Bang-Andreasen, T., Sørensen, H., and Ingerslev, M.: Micro vertical changes in soil pH and base cations over time after application of wood ash on forest soil, Forest Ecol. Manag., 406, 274–280, https://doi.org/10.1016/j.foreco.2017.09.069, 2017.
IUSS Working Group: International soil classification system for naming soils and creating legends for soil maps, in: World Soil Resources Reports no. 106, FAO, Rome, 2014.
Ji, L., Tan, W., and Chen, X.: Arbuscular mycorrhizal mycelial networks and glomalin-related soil protein increase soil aggregation in Calcaric Regosol under well-watered and drought stress conditions, Soil Till. Res., 185, 1–8, https://doi.org/10.1016/j.still.2018.08.010, 2019.
Jiang, J., Jiang, W., and Jiang, W.: The present situation and countermeasures of the development of tea industry in Guangxi, Pop. Sci. Technology, 20, 72–73, http://www.dzkjzz.net/oa/DArticle.aspx?type=view&id=201803023 (last access: 22 July 2022), 2018.
Kemper, W. D. and Chepil, W. S.: Size distribution of aggregation, Part 1, edited by: Black, C. A., American Society of Agronomy, 499–510, 1965.
Khan, K. S., Mack, R., Castillo, X., Kaiser, M., and Joergensen, R. G.: Microbial biomass, fungal and bacterial residues, and their relationships to the soil organic matter ratios, Geoderma, 271, 115–123, https://doi.org/10.1016/j.geoderma.2016.02.019, 2016.
Krouk, G. and Kiba, T.: Nitrogen and phosphorus interactions in plants: From agronomic to physiological and molecular insights, Curr. Opin. Plant Biol., 57, 104–109, https://doi.org/10.1016/j.pbi.2020.07.002, 2020.
Kurmi, B., Nath, A. J., Lal, R., and Das, A. K.: Water stable aggregates and the associated active and recalcitrant carbon in soil under rubber plantation, Sci. Total Environ., 703, 135498, https://doi.org/10.1016/j.scitotenv.2019.135498, 2020.
Li, S., Li, H., Yang, C., Wang, Y. D., Xue, H., and Niu, Y. F.: Rates of soil acidification in tea plantations and possible causes, Agr. Ecosyst. Environ., 233, 60–66, https://doi.org/10.1016/j.agee.2016.08.036, 2016.
Li, W., Zheng, Z., Li, T., Zhang, X., Wang, Y., Yu, H., He, S., and Liu, T.: Effect of tea plantation age on the distribution of soil organic carbon fractions within water-stable aggregates in the hilly region of Western Sichuan, China, Catena, 133, 198–205, https://doi.org/10.1016/j.catena.2015.05.017, 2015.
Lindsay, W. L., and Norvell, W. A.: Development of a DTPA soil test for zinc, iron, manganese, and copper, Soil Sci. Soc. Am. J., 42, 421–428, https://doi.org/10.2136/sssaj1978.03615995004200030009x, 1978.
Liu, D., Ju, W., Jin, X., Li, M., Shen, G., Duan, C., Guo, L., Liu, Y., Zhao, W., and Fang, L.: Associated soil aggregate nutrients and controlling factors on aggregate stability in semiarid grassland under different grazing prohibition timeframes, Sci. Total Environ., 777, 146104, https://doi.org/10.1016/j.scitotenv.2021.146104, 2021.
Liu, H., Wang, R., Lu, X., Cai, J., Feng, X., Yang, G., Li, H., Zhang, Y., Han, X., and Jiang, Y.: Effects of nitrogen addition on plant-soil micronutrients vary with nitrogen form and mowing management in a meadow steppe, Environ. Pollut., 289, 117969, https://doi.org/10.1016/j.envpol.2021.117969, 2021.
Liu, X., Li, L., Wang, Q., and Mu, S.: Land-use change affects stocks and stoichiometric ratios of soil carbon, nitrogen, and phosphorus in a typical agro-pastoral region of northwest China, J. Soil. Sediment., 18, 3167–3176, https://doi.org/10.1007/s11368-018-1984-5, 2018.
Lu, M., Yang, M., Yang, Y., Wang, D., and Sheng, L.: Soil carbon and nutrient sequestration linking to soil aggregate in a temperate fen in Northeast China, Ecol. Indic., 98, 869–878, https://doi.org/10.1016/j.ecolind.2018.11.054, 2019.
Lu, R.: Analysis of soil agrochemistry, Chinese Agricultural Science and Technology Press, Beijing, 2000.
Lu, X., Mao, Q., Gilliam, F. S., Luo, Y., and Mo, J.: Nitrogen deposition contributes to soil acidification in tropical ecosystems, Global Change Biol., 20, 3790–3801, https://doi.org/10.1111/gcb.12665, 2014.
Miner, G. L., Delgado, J. A., Ippolito, J. A., Barbarick, K. A., Stewart, C. E., Manter, D. K., Del Grosso, S. J., Halvorson, A. D., Floyd, B. A., and D'Adamo, R. E.: Influence of long-term nitrogen fertilization on crop and soil micronutrients in a no-till maize cropping system, Field Crop. Res., 228, 170–182, https://doi.org/10.1016/j.fcr.2018.08.017, 2018.
Nelson, D. W. and Sommers, L. E.: Total carbon, organic carbon and organic matter, Part 3, edited by: Sparks, D. L., American Society of Agronomy, 961–1010, 1996.
Otero, X. L., Fernández, S., de Pablo Hernandez, M. A., Nizoli, E. C., and Quesada, A.: Plant communities as a key factor in biogeochemical processes involving micronutrients (Fe, Mn, Co, and Cu) in Antarctic soils (Byers Peninsula, maritime Antarctica), Geoderma, 195, 145–154, https://doi.org/10.1016/j.geoderma.2012.11.018, 2013.
Piazza, G., Pellegrino, E., Moscatelli, M. C., and Ercoli, L.: Long-term conservation tillage and nitrogen fertilization effects on soil aggregate distribution, nutrient stocks and enzymatic activities in bulk soil and occluded microaggregates, Soil Till. Res., 196, 104482, https://doi.org/10.1016/j.still.2019.104482, 2020.
Prescott, C. E., Grayston, S. J., Helmisaari, H. S., Kastovska, E., Korner, C., Lambers, H., Meier, I. C., Millard, P., and Ostonen, I.: Surplus carbon drives allocation and plant-soil interactions, Trends Ecol. Evol., 35, 1110–1118, https://doi.org/10.1016/j.tree.2020.08.007, 2020.
Qiao, Y., Wang, J., Liu, H., Huang, K., Yang, Q., Lu, R., Yan, L., Wang, X., and Xia, J.: Depth-dependent soil C-N-P stoichiometry in a mature subtropical broadleaf forest, Geoderma, 370, 114357, https://doi.org/10.1016/j.geoderma.2020.114357, 2020.
Rakhsh, F., Golchin, A., Agha, A. B., and Nelson, P. N.: Mineralization of organic carbon and formation of microbial biomass in soil: Effects of clay content and composition and the mechanisms involved, Soil Biol. Biochem., 151, 108036, https://doi.org/10.1016/j.soilbio.2020.108036, 2020.
Sardans, J., Rivas-Ubach, A., and Penuelas, J.: The stoichiometry of organisms and ecosystems in a changing world: A review and perspectives, Perspect. Plant Ecol., 14, 33–47, https://doi.org/10.1016/j.ppees.2011.08.002, 2012.
Sarker, J. R., Singh, B. P., Cowie, A. L., Fang, Y., Collins, D., Badgery, W., and Dalal, R. C.: Agricultural management practices impacted carbon and nutrient concentrations in soil aggregates, with minimal influence on aggregate stability and total carbon and nutrient stocks in contrasting soils, Soil Till. Res., 178, 209–223, https://doi.org/10.1016/j.still.2017.12.019, 2018.
Six, J. and Paustian, K.: Aggregate-associated soil organic matter as an ecosystem property and a measurement tool, Soil Biol. Biochem., 68, 4–9, https://doi.org/10.1016/j.soilbio.2013.06.014, 2014.
Six, J., Bossuyt, H., Degryze, S., and Denef, K.: A history of research on the link between (micro) aggregates, soil biota, and soil organic matter dynamics, Soil Till. Res., 79, 7–31, https://doi.org/10.1016/j.still.2004.03.008, 2004.
Sparling, G., Ross, D., Trustrum, N., Arnold, G., West, A., Speir, T., and Schipper, L.: Recovery of topsoil characteristics after landslip erosion in dry hill country of New Zealand, and a test of the space-for-time hypothesis, Soil Biol. Biochem., 35, 1575–1586, https://doi.org/10.1016/j.soilbio.2003.08.002, 2003.
Stone, M. M. and Plante, A. F.: Changes in phosphatase kinetics with soil depth across a variable tropical landscape, Soil Biol. Biochem., 71, 61–67, https://doi.org/10.1016/j.soilbio.2014.01.006, 2014.
Su, L., Du, H., Zeng, F., Peng, W., Rizwan, M., Nunez-Delgado, A., Zhou, Y., Song, T., and Wang, H.: Soil and fine roots ecological stoichiometry in different vegetation restoration stages in a karst area, southwest China, J. Environ. Manage., 252, 109694, https://doi.org/10.1016/j.jenvman.2019.109694, 2019.
Sun, D., Zong, M., Li, S., Li, H., Duan, C., Peng, C., Zhao, Y., Bai, J., Lin, C., Feng, Y., Huang, W., and Wang, D.: The effects of the soil environment on soil organic carbon in tea plantations, Agr. Ecosyst. Environ., 297, 106951, https://doi.org/10.1016/j.agee.2020.106951, 2020.
Thomas, G. W.: Exchangeable cations, Part 2, edited by: Page, A. L., American Society of Agronomy, 159–165, 1982.
Tian, H., Chen, G., Zhang, C., Melillo, J. M., and Hall, C. A. S.: Pattern and variation of ratios in China's soils: A synthesis of observational data, Biogeochemistry, 98, 139–151, https://doi.org/10.1007/s10533-009-9382-0, 2010.
Tian, Q., Liu, N., Bai, W., Li, L., Chen, J., Reich, P. B., Yu, Q., Guo, D., Smith, M. D., Knapp, A. K., Cheng, W., Lu, P., Gao, Y., Yang, A., Wang, T., Li, X., Wang, Z., Ma, Y., Han, X., and Zhang, W.: A novel soil manganese mechanism drives plant species loss with increased nitrogen deposition in a temperate steppe, Ecology, 97, 65–74, https://doi.org/10.1890/15-0917.1, 2016.
Tisdall, J. M. and Oades, J. M.: Organic matter and water-stable aggregates in soils, Eur. J. Soil Sci., 33, 141–163, https://doi.org/10.1111/j.1365-2389.1982.tb01755.x, 1982.
Tong, C., Xiao, H., Tang, G., Wang, H., Huang, T., Xia, H., Keith, S. J., Li, Y., Liu, S., and Wu, J.: Long-term fertilizer effects on organic carbon and total nitrogen and coupling relationships of C and N in paddy soils in subtropical China, Soil Till. Res., 106, 8–14, https://doi.org/10.1016/j.still.2009.09.003, 2009.
Wang, R., Dungait, J. A. J., Buss, H. L., Yang, S., Zhang, Y., Xu, Z., and Jiang, Y.: Base cations and micronutrients in soil aggregates as affected by enhanced nitrogen and water inputs in a semi-arid steppe grassland, Sci. Total Environ., 575, 564–572, https://doi.org/10.1016/j.scitotenv.2016.09.018, 2017.
Wang, S. and Ye, S.: Dynamics of bacterial function characteristics in soil aggregates during the tea-planting process, Geoderma, 377, 114609, https://doi.org/10.1016/j.geoderma.2020.114609, 2020.
Wang, S., Li, T., and Zheng, Z.: Distribution of microbial biomass and activity within soil aggregates as affected by tea plantation age, Catena, 153, 1–8, https://doi.org/10.1016/j.catena.2017.01.029, 2017.
Wang, S., Li, T., and Zheng, Z.: Tea plantation age effects on soil aggregate-associated carbon and nitrogen in the hilly region of western Sichuan, China, Soil Till. Res., 180, 91–98, https://doi.org/10.1016/j.still.2018.02.016, 2018.
Wu, W., Zheng, Z., Li, T., He, S., Zhang, X., Wang, Y., and Liu, T.: Distribution of inorganic phosphorus fractions in water-stable aggregates of soil from tea plantations converted from farmland in the hilly region of western Sichuan, China, J. Soil. Sediment., 18, 906–916, https://doi.org/10.1007/s11368-017-1834-x, 2018.
Wulanningtyas, H. S., Gong, Y., Li, P., Sakagami, N., Nishiwaki, J., and Komatsuzaki, M.: A cover crop and no-tillage system for enhancing soil health by increasing soil organic matter in soybean cultivation, Soil Till. Res., 205, 104749, https://doi.org/10.1016/j.still.2020.104749, 2021.
Xie, S., Yang, F., Feng, H., Yu, Z., Liu, C., Wei, C., and Liang, T.: Organic fertilizer reduced carbon and nitrogen in runoff and buffered soil acidification in tea plantations: Evidence in nutrient contents and isotope fractionations, Sci. Total Environ., 762, 143059, https://doi.org/10.1016/j.scitotenv.2020.143059, 2020.
Xu, C., Pu, L., Li, J., and Zhu, M.: Effect of reclamation on C, N, and P stoichiometry in soil and soil aggregates of a coastal wetland in eastern China, J. Soil. Sediment., 19, 1215–1225, https://doi.org/10.1007/s11368-018-2131-z, 2019.
Xue, D., Yao, H., and Huang, C.: Microbial biomass, N mineralization and nitrification, enzyme activities, and microbial community diversity in tea orchard soils, Plant Soil, 288, 319–331, https://doi.org/10.1007/s11104-006-9123-2, 2006.
Yan, P., Shen, C., Fan, L., Li, X., Zhang, L., Zhang, L., and Han, W.: Tea planting affects soil acidification and nitrogen and phosphorus distribution in soil, Agr. Ecosyst. Environ., 254, 20–25, https://doi.org/10.1016/j.agee.2017.11.015, 2018.
Yang, X., Ni, K., Shi, Y., Yi, X., Zhang, Q., Fang, L., Ma, L., and Ruan, J.: Effects of long-term nitrogen application on soil acidification and solution chemistry of a tea plantation in China, Agr. Ecosyst. Environ., 252, 74–82, https://doi.org/10.1016/j.agee.2017.10.004, 2018.
Yang, Y. H., Fang, J. Y., Guo, D. L., Ji, C. J., and Ma, W. H.: Vertical patterns of soil carbon, nitrogen and carbon: nitrogen stoichiometry in Tibetan grasslands, Biogeosciences Discuss., 7, 1–24, https://doi.org/10.5194/bgd-7-1-2010, 2010.
Yu, P., Liu, S., Xu, Q., Fan, G., Huang, Y., and Zhou, D.: Response of soil nutrients and stoichiometric ratios to short-term land use conversions in a salt-affected region, northeastern China, Ecol. Eng., 129, 22–28, https://doi.org/10.1016/j.ecoleng.2019.01.005, 2019.
Yu, Z., Wang, M., Huang, Z., Lin, T. C., Vadeboncoeur, M. A., Searle, E. B., and Chen, H. Y. H.: Temporal changes in soil C-N-P stoichiometry over the past 60 years across subtropical China, Global Change Biol., 24, 1308–1320, https://doi.org/10.1111/gcb.13939, 2018.
Yue, K., Fornara, D. A., Yang, W., Peng, Y., Li, Z., Wu, F., and Peng, C.: Effects of three global change drivers on terrestrial stoichiometry: A global synthesis, Global Change Biol., 23, 2450–2463, https://doi.org/10.1111/gcb.13569, 2017.
Zanella, A., Ponge, J. F., and Briones, M. J.: Terrestrial humus systems and forms: Biological activity and soil aggregates, space-time dynamics, Appl. Soil Ecol., 122, 103–137, https://doi.org/10.1016/j.apsoil.2017.07.020, 2018.
Zeng, L., Li, J., Zhou, Z., and Yu, Y.: Optimizing land use patterns for the grain for Green Project based on the efficiency of ecosystem services under different objectives, Ecol. Indic., 114, 106347, https://doi.org/10.1016/j.ecolind.2020.106347, 2020.
Zhang, Q., Gao, W., Su, S., Weng, M., and Cai, Z.: Biophysical and socioeconomic determinants of tea expansion: Apportioning their relative importance for sustainable land use policy, Land Use Policy, 68, 438–447, https://doi.org/10.1016/j.landusepol.2017.08.008, 2017.
Zhang, S., Yan, L., Huang, J., Mu, L., Huang, Y., Zhang, X., and Sun, Y.: Spatial heterogeneity of soil C:N ratio in a mollisol watershed of northeast China, Land Degrad. Dev., 27, 295–304, https://doi.org/10.1002/ldr.2427, 2016.
Zheng, Z., Wang, Y., Li, T., and Yang, Y.: Effect of abandoned cropland on stability and distribution of organic carbon in soil aggregates, J. Nat. Resour., 26, 119–127, https://doi.org/10.11849/zrzyxb.2011.01.012, 2011.
Zhou, Y., Boutton, T. W., and Wu, X. B.: Soil stoichiometry responds to vegetation change from grassland to woodland, Biogeochemistry, 140, 341–357, https://doi.org/10.1007/s10533-018-0495-1, 2018.
Zhou, Z., Gan, Z., Shangguan, Z., and Zhang, F.: Effects of long-term repeated mineral and organic fertilizer applications on soil organic carbon and total nitrogen in a semi-arid cropland, Eur. J. Agron., 45, 20–26, https://doi.org/10.1016/j.eja.2012.11.002, 2013.
Zhu, R., Zheng, Z., Li, T., Zhang, X., He, S., Wang, Y., Liu, T., and Li, W.: Dynamics of soil organic carbon mineralization in tea plantations converted from farmland at Western Sichuan, China, PloS One, 12, e0185271, https://doi.org/10.1371/journal.pone.0185271, 2017.
Zhu, R., Zheng, Z., Li, T., He, S., Zhang, X., Wang, Y., and Liu, T.: Effect of tea plantation age on the distribution of glomalin-related soil protein in soil water-stable aggregates in southwestern China, Environ. Sci. Pollut. R., 26, 1973–1982, https://doi.org/10.1007/s11356-018-3782-4, 2019.