the Creative Commons Attribution 4.0 License.
the Creative Commons Attribution 4.0 License.
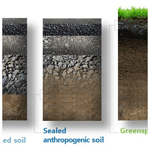
The effects of sealing on urban soil carbon and nutrients
Roisin O'Riordan
Jess Davies
Carly Stevens
John N. Quinton
Urban soils are of increasing interest for their potential to provide ecosystem services such as carbon storage and nutrient cycling. Despite this, there is limited knowledge on how soil sealing with impervious surfaces, a common disturbance in urban environments, affects these important ecosystem services. In this paper, we investigate the effect of soil sealing on soil properties, soil carbon and soil nutrient stocks. We undertook a comparative survey of sealed and unsealed green space soils across the UK city of Manchester. Our results reveal that the context of urban soil and the anthropogenic artefacts added to soil have a great influence on soil properties and functions. In general, sealing reduced soil carbon and nutrient stocks compared to green space soil; however, where there were anthropogenic additions of organic and mineral artefacts, this led to increases in soil carbon and nitrate content. Anthropogenic additions led to carbon stocks equivalent to or larger than those in green spaces; this was likely a result of charcoal additions, leading to carbon stores with long residence times. This suggests that in areas with an industrial past, anthropogenic additions can lead to a legacy carbon store in urban soil and make important contributions to urban soil carbon budgets. These findings shed light on the heterogeneity of urban sealed soil and the influence of anthropogenic artefacts on soil functions. Our research highlights the need to gain a further understanding of urban soil processes, in both sealed and unsealed soils, and of the influence and legacy of anthropogenic additions for soil functions and important ecosystem services.
- Article
(2320 KB) - Full-text XML
- BibTeX
- EndNote
To date, little attention has been given to urban soil and its functions; however, the importance of urban soil is increasingly being recognised due to its role supporting sustainable urban development and the provision of soil ecosystem services in cities (Pavao-Zuckerman, 2012; Morel et al., 2015; Yang and Zhang, 2015; Vasenev et al., 2018; Pouyat et al., 2020). In particular, interest has increased in the ability of urban soils to store carbon (C) and contribute to climate regulation, as well as cycling nutrients and supporting urban plant growth (Pouyat et al., 2006; Edmondson et al., 2014; Herrmann et al., 2017; Setala et al., 2017). The number of people living in urban areas is projected to grow, with almost 70 % of the world's population expected to live in urban areas by 2050 (United Nations, 2019). This expansion of urban areas will have consequences for soil and the ecosystem services it's able to provide in urban areas.
Urbanisation leads to highly heterogeneous soils that exhibit a wide range of soil properties (Lehmann and Stahr, 2007) and changes in soil structure such as alterations to soil horizons (Herrmann et al., 2018). It also leads to additions of anthropogenic materials known as artefacts, which include brick, concrete, metals and plastics (Bullock and Gregory, 1991; Lehmann and Stahr, 2007), as well as contamination with heavy metals which can alter nutrient cycles (Zhao et al., 2013), and organic and inorganic pollutants (Li et al., 2018). Increasing urbanisation will lead to increased soil disturbance, contamination and soil sealing with impermeable surfaces, such as roads and pavements (Scalenghe and Marsan, 2009; EU, 2012; Artmann, 2014). Soil sealing commonly involves the removal of topsoil, also known as scalping, where the upper part of the soil is removed down to the subsoil, thus creating a new soil surface (Lehmann, 2006). Sealing also leads to additions of anthropogenic materials to sealed soils, such as road foundation aggregates and other human-made artefacts, before it is then sealed with tarmac, concrete or paving slabs. As such, sealing is likely to have large effects on soil functioning and the soil-mediated ecosystem services of C storage, water regulation and nutrient cycling.
Recent studies have revealed the notable C storage potential of urban soils (Pouyat et al., 2006; Raciti et al., 2011; Lorenz and Lal, 2015; Vasenev and Kuzyakov, 2018), though less is known about the C storage of sealed soils. Early studies assumed low to zero storage of C beneath sealed surfaces (Bradley et al., 2005). However, more recent research has illustrated that sealed soil C makes an important contribution to wider urban soil C stores (Edmondson et al., 2012; Cambou et al., 2018). Studies indicate that, in general, soil sealing leads to a reduction in soil organic carbon (SOC) (Raciti et al., 2012; Wei et al., 2014a, b; Piotrowska-Długosz and Charzyński, 2015; Vasenev and Kuzyakov, 2018). However, some studies have found similar SOC storage between sealed and non-sealed soils at equivalent depths, indicating that reductions in SOC stocks near the surface were a result of topsoil removal, while stocks in subsoils were no different (Edmondson et al., 2012; Cambou et al., 2018). Inorganic C (IC) also provides an important contribution to C storage in urban soils (Vasenev and Kuzyakov, 2018; Pouyat et al., 2020); however, IC is much less commonly studied in sealed soils. Therefore, the knowledge on soil C storage in sealed soils remains limited; there is a need to further understand the storage potential of sealed soil, the SOC and IC dynamics beneath sealed surfaces, and how this contributes to wider urban soil C storage.
Knowledge is also limited on the effects of soil sealing on nutrient stores, as well as how anthropogenic activities may influence these and the subsequent consequences for urban soil nutrient cycling. A number of studies have revealed that sealing generally leads to a reduction in nitrogen (N) storage (Raciti et al., 2012; Wei et al., 2014a, b; Piotrowska-Długosz and Charzyński, 2015; Majidzadeh et al., 2017), and it has been suggested that there is a decoupling of C and N in sealed soils (Raciti et al., 2012; Wei et al., 2014a). The processes involved in nutrient cycling have, however, been less studied. Observations of N mineralisation and nitrification suggest that these processes are reduced by sealing (Zhao et al., 2012; Wei et al., 2014b), while observations of ammonium and nitrate levels have varied (Zhao et al., 2012; Martinová et al., 2016; Majidzadeh et al., 2018). We are yet to have a clear understanding of both the impacts of sealing on N cycling processes and the mechanisms behind these alterations. In addition, there is very limited research on phosphorus (P) in sealed soils, despite this being an important nutrient for plant productivity and water quality. Studies have observed higher Olsen P and extractable P in sealed soils (Wei et al., 2014b; Martinová et al., 2016; Majidzadeh et al., 2017, 2018), while one study found no difference in available P between sealed and unsealed soils (Piotrowska-Długosz and Charzyński, 2015). Therefore, the effects of urban soil sealing on soil P remain largely unclear. The availability of N and P has implications for soil organic matter (SOM) mineralisation and C storage, as well as soil nutrient status and leaching, and at present, knowledge on the dynamics between C, N and P in sealed soil is lacking.
There is a need to further our understanding of C and nutrient stocks in sealed soils, to gain a clearer picture of how they contribute to C and nutrient stocks across the wider urban landscape, and what the implications are for urban soil ecosystem services. In this paper, we aim to investigate the effects of soil sealing on urban soils, their properties, and important soil functions including carbon storage and nutrient cycling. We undertake a comparative survey of soil C and nutrients in sealed and unsealed soils across the UK city of Manchester, constituting one of the largest studies of sealed soil to date. Our objectives were to investigate the effects of sealing and anthropogenic activities on (i) soil properties (ii) soil C stocks, and (iii) nutrient contents and stocks.
2.1 Study area
Soils were sampled from Greater Manchester, a metropolitan region in the north-west of the UK with a population of 2.8 million (ONS, 2021). The study focused on the wider city area within the M60 motorway and the town of Rochdale within the Greater Manchester region (Fig. 1). The National Soil Map for England and Wales, via the Soilscapes Viewer online (Soilscapes, 2020), shows that the area east of the M60 has slowly permeable, seasonally wet, acid loamy and clayey soils. The south-west has naturally wet and very acidic sandy and loamy soils, while the north-west and Rochdale are a combination of slowly permeable, wet acidic loamy and clayey soils with areas of floodplain soil with high groundwater, as well as areas of freely draining slightly acidic sandy or loamy soils.
2.2 Soil sampling
Sampling was undertaken to allow for a comparison of sealed and unsealed green space soils. Sealed soils were sampled from roadworks or construction sites where work had recently opened up the sealed surface of roads and pavements; unsealed soils were sampled from the nearest green space, park or roadside within a grassed lawn area. The distance between sealed and green space sites varied with each sample (between 0.25–330 m), and as such they are not considered paired samples. All soils were sampled to a depth of 10 cm of available soil. In green spaces, soils were sampled from open grassed areas where litter consisted of roots and dead grass leaves. The turf and root mat were removed, and the soil was sampled down to 10 cm. In sealed soils, imported construction materials consisted of limestone gravel or chips, construction rubble including brick or concrete, sharp sand, charcoal, and ash. Profiles and horizons were not consistent across the sites due to the heterogeneous nature of soil sealing. In general, profiles consisted of a sealed surface, various layers of road or pavement foundation materials, and a clay-rich subsoil underneath (see Fig. A1, Appendix A). The depth of construction materials varied from 30–110 cm depth; most samples were collected between 60–80 cm depth, sampling the top 10 cm of available soil under the construction materials. This sampling method allowed for a comparison between the top available soil in each profile to understand the properties and functions of each soil. At all sites two samples were collected: one using a metal bulk density core (6 cm diameter) and a second sample using a trowel for additional analyses using fresh soil. Samples were collected in plastic bags, kept in a cool box while transported, and refrigerated until fresh soil analyses were undertaken within 1 week. A total of 68 sites were sampled, with 36 sealed samples and 32 green space samples.
2.3 Soil analysis
2.3.1 Urban soil categorisation
Urban soil often contains large amounts of anthropogenic additions, or artefacts, which are human-made or derived materials and can include bricks, pottery, glass, crushed stone, charcoal cinders, wood or waste materials. Technosols are defined in the World Reference Base for Soil Resources (WRB) as either containing large amounts of artefacts, having an impermeable geomembrane or having a technic hard material at the soil surface, such as concrete, asphalt or worked stone (FAO, 2015). All the sealed soil samples collected are considered Technosols as a result of the continuous hard sealed surface. However, we observed that some sealed samples contained numerous artefacts while others did not and, apart from being sealed, appeared relatively undisturbed by human activity. These artefacts appeared to be fragments of the materials used in road or pavement construction (see Sect. 2.2), which had disintegrated and been mixed into the soil. The addition of artefacts can considerably alter the properties and functions of soil, such as water holding, C storage or nutrient status. As such, the sealed samples were categorised into two types: those relatively undisturbed other than by sealing, herein referred to as sealed undisturbed soils (SU), and those with notable additions of anthropogenic artefacts, herein referred to as sealed anthropogenic soil (SA) (Fig. 2).
Wet sieving was undertaken on subsamples of the sealed soils to distinguish between SU and SA soils. We used the proportion of material in the >200 µm fraction to determine the level of anthropogenic additions and serve as a proxy for the proportion of artefacts. Soils with visible artefacts exhibited more than 40 % of subsample mass in the >200 µm fraction; thus, subsamples with more than 40 % mass in the >200 µm fraction were classed as SA soils, and those with less than 40 % in the >200 µm fraction were classed as SU soils. The fragmentation of artefacts into smaller fractions made it impractical and inaccurate to use a measure of the mass of artefacts alone. Using material >200 µm served to describe the samples well and enabled a consistent comparison between anthropogenic and undisturbed soils.
2.3.2 Soil preparation and properties
As urban soil commonly contains imported anthropogenic materials, the in situ volume, density and structural properties of the soil are related to these materials, and in some cases, they make up a large proportion of the soil. As such, we deemed it appropriate not to remove the course fraction above 2 mm prior to bulk density calculation to provide a representation of the in situ soil. Bulk density cores were weighed, dried at 105 ∘C for 48 h (due to high clay content) and then reweighed to enable calculations of bulk density and gravimetric soil moisture. They were then used to measure the proportion of material >200 µm by sieving to determine soils with anthropogenic additions.
The second set of samples were used for the fresh soil analyses of pH, nitrate and ammonium extraction. For pH analysis, 10 g of fresh soil was mixed with 25 mL of distilled water, shaken on an orbital shaker for 30 min at 180 rpm and left to settle for 30 min. The pH was then measured with a pH probe (Mettler Toledo, SevenCompact S220) at the soil–water interface. The remaining fresh sample was dried at 70 ∘C for 48 h. It was then homogenised using a pestle and mortar, passed through a 2 mm sieve, and subsamples were taken for analyses of total P, extractable organic carbon (OC) and inorganic C. Further subsamples were then dried to 105 ∘C prior to CN analysis and loss on ignition. Many samples of sealed soil were high in clay, and for this reason the method deviates from the traditional approach to sieving the samples prior to drying.
2.3.3 Carbon and nutrient analysis
To determine total C and N concentrations, samples were dried at 105 ∘C for 24 h, ball-milled to a powder, and analysed for total C and total N concentration as well as CN ratio using a dry combustion CN analyser (El Vario analyser, Elementar, Hanau, Germany). Soil organic matter (SOM) was estimated using the loss on ignition (LOI) method described by Heiri et al. (2001). Samples were dried at 105 ∘C for 24 h prior to weighing, heated at 550 ∘C for 6 h and reweighed to determine the loss of SOM by weight as a percentage.
Organic C (OC) and inorganic C rendered extractable to 0.5 M K2SO4 were measured, as described by Vance et al. (1987) without fumigation with CHCl3 so as to measure non-microbial biomass C. Briefly, the acidity of the K2SO4 was checked and adjusted to between pH 6.8–7 using NaOH. For the extraction, 5 g of dry soil was mixed with 20 mL of K2SO4 (0.5 M) and shaken on an orbital shaker for 1 h at 180 rpm. It was left to settle for 10 min and then filtered through a Whatman no. 42 filter. Filtrate was diluted 1 part to 8 parts Milli-Q water and analysed for extractable total and inorganic C in a total organic carbon (TOC) analyser (Shimadzu TOC-L_CPN TN). Extractable OC is determined as total extractable C minus extractable inorganic C.
Ammonium and nitrate pools were measured with the aim of understanding mineral N content and dynamics. The extraction was undertaken using 1 M of KCl as an extraction matrix (Kachurina et al., 2000; Saha et al., 2018). For extractions, 5 g of fresh soil was mixed with 25 mL KCl (1 M) and shaken on an orbital shaker for 1 h at 180 rpm. It was filtered through a Whatman no. 6 filter, and the filtrate was analysed for nitrate and ammonium using a colorimetric segmented flow analyser (AA3, Seal Analytical, Southampton, UK). Total phosphorous (P) content was analysed using a sulfuric acid/hydrogen peroxide digestion method by Rowland and Grimshaw (1985). For the digest, 0.2 g of dry ground soil was mixed with 4.4 mL digest reagent and heated gently until the vigorous reaction had subsided. Heat was increased to 400 ∘C and boiled for 2 h until the digest had cleared. Once cool, samples were diluted to 50 mL with Milli-Q water and filtered using a Whatman no. 6 filter. Filtrate was diluted a further five times and analysed for total P on a colorimetric segmented flow analyser (AA3, Seal Analytical, Southampton, UK). Soil total C, N and P stocks were calculated using bulk density values to a depth of 10 cm and C, N and P contents, as per the guidance from the FAO (2018).
2.4 Statistical analysis
Analysis was undertaken to determine the difference between sealed and green space soils and also to determine whether there was a difference between the two categories of sealed soil, i.e. SU and SA soils. Data for the majority of variables did not exhibit a normal distribution according to the Shapiro–Wilks test. As such, all datasets were analysed using the non-parametric Kruskal–Wallace test to identify significant differences between the three categories of soil and the Dunn–Bonferroni post hoc test to determine where the significant difference was between soil categories. All analyses were undertaken in SPSS 26 software, and figures were produced using R version 4.1.0.
Sealed samples were categorised into two groups: those that were relatively undisturbed other than by sealing, referred to as sealed undisturbed (SU) soils, and those with notable additions of anthropogenic material, referred to as sealed anthropogenic (SA) soils. Of the 36 sealed samples, 22 were classed as SU soils and 14 were classed as SA soils. The results of the analyses are summarised in Table A1 in Appendix A. Results are presented for soil properties, soil C and SOM analyses, nutrient contents, and stoichiometry respectively.
3.1 Soil properties
Figure 3 summarises the soil properties across the sealed and green space categories. Both categories of sealed soil had significantly higher pH than the green space soil (p<0.001); while both had lower soil moisture, only SA soil was significantly lower than green space soil (p=0.006). Both pH and soil moisture data showed no significant difference between the two sealed soils; however, all three soils exhibited significantly different bulk densities (p<0.001) with SU soils having the highest bulk density and SA soil the next highest (Fig. 3a and b).
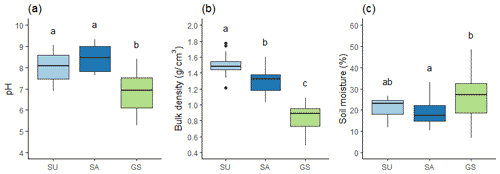
Figure 3Soil properties: (a) pH, (b) bulk density (g cm−3), and (c) soil moisture content (%) for the SU (n=22), SA (n=14), and green space (GS; n=32) soils. Boxplots show upper and lower quartiles, whiskers show upper and lower most values, and horizontal line shows the median. Circled data points show outliers at 1.5 times the interquartile range (IQR). Different letters indicate a statistically significant difference between soil categories at the 0.05 level.
3.2 Carbon
Total C data indicated that SU soil had significantly lower total C concentration than both other soils (p<0.001), while SA soil was statistically no different to green space soil. Total C stock for the top 10 cm of soil exhibited a significant difference across all three soil categories (p<0.001) (Fig. 4b), with SA soil having significantly greater total C stock than green space soil (8.06±4.65 kg m2 and 4.92±1.11 kg m2 respectively) and green space soil having significantly greater stock than SU soil (3.10±1.45 kg m2). SU soil had 37 % less total C stock than green space soil, while SA soil showed an increase of 64 % total C stock on that of green space soil.
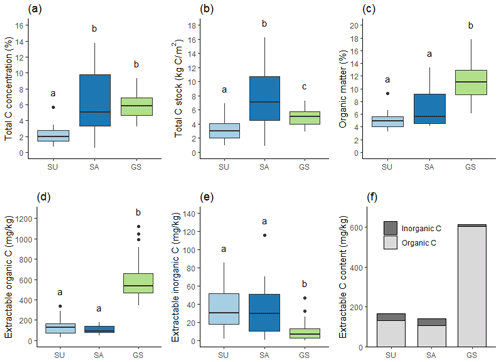
Figure 4Carbon measurements: (a) total C concentration, (b) total C stock, (c) organic matter concentration, (d) K2SO4 extractable organic C content, (e) K2SO4 extractable inorganic C content, and (f) mean K2SO4 extractable total C content; there are for SU (n=22), SA (n=14) and green space (GS; n=32) soils. Stocks are calculated for the top 10 cm of available soil. Different letters indicate a statistically significant difference between soil categories at the 0.05 level.
By contrast, SOM data indicated that both sealed soils had significantly lower SOM concentration than green space soil (p<0.001), and while SA did have greater SOM concentration than SU soil (7.06 % ±3.20 compared to 5.00 % ±1.29), there was no statistically significant difference between them (Fig. 4c). SU soil had 55 % less SOM and SA soil 37 % less SOM than green space soil. SA soils exhibited more variability in total C stock data than in SOM values, with SD=4.65 for total C stock and SD=3.20 for SOM concentration.
Extractable C (K2SO4) was analysed to investigate the amount of extractable organic and inorganic C in the samples. In the sealed soils, 76 %–79 % of the extractable C was organic C and 21 %–24 % was inorganic C. In the green space soils, 98 % of extractable C was organic C, while only 2 % was inorganic C (Fig. 4f). Both sealed soils exhibited significantly lower extractable organic C than green space soils (p<0.001), with no significant difference between the two sealed soils (Fig. 4d). Extractable inorganic C was significantly higher in both sealed soils compared to green space soil (p<0.001), with, again, no significant difference between the two sealed soils.
3.3 Nutrients
Both sealed soils exhibited significantly lower total N stock than green space soils (p<0.001), while the two sealed soils showed no significant difference between them (Fig. 5a and b). SU soil had the lowest total N stock (81.20 g m2±22.47) followed by SA soil (90.11 g m2±28.38), with green space soil having the greatest total N stock (115.44 g m2±26.49). Total P stock showed a similar pattern, with both sealed soils exhibiting significantly lower total P stock than green space soil (p=0.003) (Fig. 5e and f). SU soil had the lowest total P stock (39.62 g m2±20.91), followed by SA soil (39.42 g m2 ± 23.68) and then green space soil with the greatest total P stock (62.62 g m2±36.67).
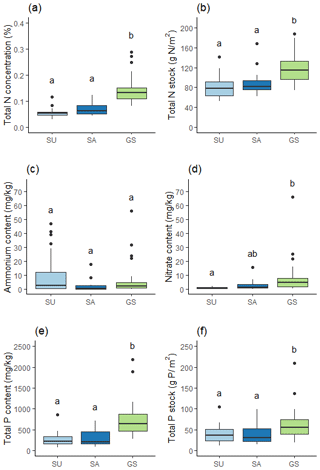
Figure 5Soil nutrients: (a) total N concentration, (b) total N stock, (c) ammonium content, (d) nitrate content, (e) total P content, and (f) total P stock; these are for SU (n=22), SA (n=14) and green space (GS; n=32) soils. Stocks are calculated for the top 10 cm of available soil. Different letters indicate a statistically significant difference between soil categories at the 0.05 level.
Analysis of ammonium and nitrate pools and illustrated that SU soil contained greater ammonium content than SA or green space soils (Fig. 5c), with a mean of 11.06 mg kg−1 (±15.52) compared to 2.53 mg kg−1 (±4.88) and 6.10 mg kg−1 (±11.74) respectively; however, there was no statistically significant difference in ammonium between the three soils (p=0.100). Conversely, SU soil exhibited significantly lower nitrate content compared to green space soil (p<0.001), while there was no significant difference in nitrate between the SA and green space soil (Fig. 5d).
3.4 Stoichiometry
The C:N ratio was significantly higher in SA soil than SU or green space soil (p<0.001), and C:N ratio data for SA soil exhibited much greater variability than that of SU or green space soil (SD = 40.45; 12.44 and 6.99 respectively). There was no significant difference between the C:N ratios of SU and green space soils (Fig. 6a). The C:P ratio showed a similar pattern, with SA soil having a much higher C:P ratio than SU or green space soil (p<0.001), while there was no significant difference between the SU and green space soils (Fig. 6b). There was no significant difference in N:P ratio between the three soil categories (Fig. 6c).
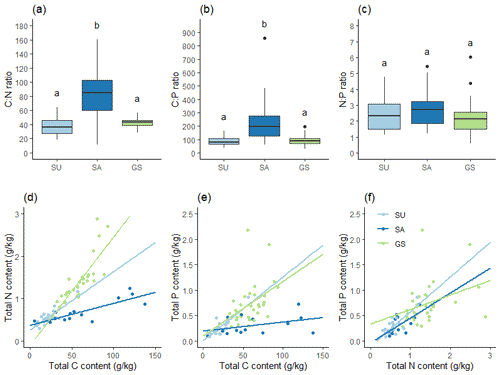
Figure 6Stoichiometry: (a) C:N ratio, (b) C:P ratio, (c) N:P ratio, correlations of (d) C and N, (e) C and P, and (f) N and P; these are for SU (n=22), SA (n=14) and green space (GS; n=32) soils. Different letters indicate a statistically significant difference between soil categories at the 0.05 level.
Correlation analysis highlighted how SA soil differed from SU and green space soil in the relationship between total C and total N, and total C and total P. In SA soil, the C:N and C:P ratios were both significantly greater than those in SU and green space soil (Fig. 6a and b); while the N:P ratio showed no significant difference across all three soils (Fig. 6c). There were significant positive correlations between total C and N for all three soils (p<0.001). Figure 6d shows the difference in correlations, illustrating that in SA soil, total C increased markedly though total N did not; while in green space soil, both total C and N increased in concordance. SU soil exhibited a positive relationship between total C and N to a lesser degree. A similar pattern was seen in total C and total P correlation (Fig. 6e); however, SU soil exhibited a similarly positive relationship between total C and P to that seen in green space soil (p<0.001 and p=0.019 respectively). SA soil did not show a significant correlation between total C and P. Correlation between total N and total P (Fig. 6f) illustrated relatively strong positive correlations in both SU and SA soils (p<0.001 and p=0.007 respectively), though no significant correlation was seen in green space soil.
4.1 Sealed soil C stocks
4.1.1 SA soil and anthropogenic additions
We found that where there are additions of anthropogenic material to sealed soils, they can provide notably large soil C stores. Our results indicate that SA soil had much greater total C stocks than green space soil, and also greater SOM contents than SU soil, though this was not statistically significantly. SA soil had total C stock of 8.06 kg C m2 between 0 and 10 cm depth which is markedly greater than published data for sealed soils to date, while SU soil stored 3.10 kg C m2, which is comparable, while still larger than most existing sealed soil C observations. Previous studies have observed stocks of 2.35 kg OC m−2 between 0–20 cm in Nanjing City, China (Wei et al., 2014b); 2.29 kg OC m−2 between 0–15 cm in New York (Raciti et al., 2012); and 1.25 kg C m−2 between 0–10 cm in Alabama, USA (Majidzadeh et al., 2017). In Leicester, UK, Edmondson et al. (2012) found stores of 6.7 kg OC m−2 between 40–100 cm under roads, which at equivalent depths to this study would also represent smaller C stocks than SA soil. It is likely that the large discrepancy between SA stocks and previous observations is due to the anthropogenic additions of C to the SA soil. This suggests that materials used in road construction and artefacts added to the soil can contribute significant amounts of C to sealed soil. This flow of materials into soil is commonly cited as a key characteristic of urban soils, in particular Technosols, and leads to the mixing of these materials into soil horizons (Bullock and Gregory, 1991; Weil and Brady, 2016; Herrmann et al., 2018). This highlights the need to consider history, land use and archaeology alongside soil science when studying urban soils (Lehmann and Stahr, 2007; Ziter and Turner, 2018). The influence of human activity on urban soil has been termed a “cultural layer” which can contribute to urban soil C stores (Vasenev et al., 2013) and, as illustrated by our results, can create a legacy of C storage.
In contrast to the high total C values, SA soil had lower SOM and extractable OC content than green space soil. This suggests that some of the total C measured was not detected in the SOM or extractable OC analyses and, thus, was a more stable form of OC or inorganic C. During the sealed soil categorisation (Sect. 2.2.4) anthropogenic artefacts were found which would contribute to stable OC or inorganic C in the soil, such as charcoal, concrete and limestone rubble. Charcoal cinders were historically a plentiful waste product from industry and coal power stations in the UK through the 19th and 20th centuries and were commonly used as a base layer on top of subsoil in road construction in the UK and USA (MacBride, 2013). This stable OC would not have been detected in extractable OC analysis, and it may also not have been completely combusted at the temperatures used in LOI as recalcitrant OC, such as black C, can burn to approximately 600 ∘C (Edmondson et al., 2015). These charcoal cinder additions provide similar recalcitrant OC storage to that of black C, which is ubiquitous in unsealed urban soils as a result of traffic and fossil fuel burning (Hamilton and Hartnett, 2013). Black C plays an important role in soil C storage due to its long residence time and its protection from rapid decomposition (Kuzyakov et al., 2014; Lehmann et al., 2015), allowing it to contribute to stable long-term urban C stores. The contribution of black C to urban soil is a small but important area of study (Rawlins et al., 2008; Edmondson et al., 2015; Schifman et al., 2018); however, at present little is known about black C or charcoal within sealed soils. Our results illustrate that historical human activity may have contributed notable amounts of stable OC to sealed soils in the UK and possibly the USA and that these likely make a significant contribution to urban soil stable C stores in these areas. This has also been observed in New York, where Technosols formed from coal ash exhibited much higher OC stocks than other soils, illustrating that human transported materials can be a source of high OC stocks (Cambou et al., 2018). However, further work on the stability of sealed soil OC is needed to fully understand the C dynamics and long-term impacts on C storage.
Some of this additional stable C may also have come from recalcitrant IC sources. IC, such as carbonate, does not thermally decompose until reaching temperatures of approximately 700–800 ∘C (Washbourne et al., 2012; Edmondson et al., 2015) and thus would also not be captured in LOI analysis. In addition, K2SO4 extraction of IC may not have extracted all recalcitrant IC in the sample. We found high IC content in our sealed samples, consistent with other observations of urban soils, which is due to the weathering of calcium minerals from concrete (Washbourne et al., 2015; Weil and Brady, 2016) and the use of calcareous materials such as cement-based rubble and limestone in road subbase layers, which add calcium and carbonates to urban soil (Shaw and Reeve, 2008; Kida and Kawahigashi, 2015; Asabere et al., 2018). Soil IC stocks make an important contribution to sealed soils and subsoils in cities, highlighting their importance as hidden stocks in C assessments (Vasenev and Kuzyakov, 2018). The importance of calcium-rich minerals and dissolved carbonates in urban soil has been highlighted for the removal of CO2 to form calcium carbonate (Washbourne et al., 2015; Jorat et al., 2020), which is a process that is also being promoted for agricultural soils as a carbon capture mechanism (Beerling et al., 2020). While little is known about water or air flow under sealed surfaces, this process has been observed in sealed soils where cracks in paving have allowed water to infiltrate, and dissolved calcium reacted to form calcium carbonate which then moved into deeper soil horizons (Kida and Kawahigashi, 2015). At present, the extent to which this process is occurring in sealed soils is unknown.
4.1.2 SU soil compared to green space soil
The SU soil had been sealed and remained largely undisturbed and altered by human activity following sealing. In comparison with green space soil, SU soil exhibited reduced C stocks, a pattern that has been seen in most other studies of sealed soil (Raciti et al., 2012; Wei et al., 2014a, b; Majidzadeh et al., 2017, 2018). Our results showed a reduction of 37 % of C stock in SU soil compared to green space soil, a notably smaller reduction than that seen in other studies, where reductions were 66 % in New York (Raciti et al., 2012), 68 % in China (Wei et al., 2014a), and 61.86 % in Alabama and Georgia, USA (Majidzadeh et al., 2017). However, a review by Vasenev and Kuzyakov (2018) found that on average, at 50–100 cm depth, sealed soil OC stocks were 25 % lower than under lawns but 10 % higher than under trees and shrubs. This suggests that C losses may be smaller further down the soil profile and additionally that the context of the unsealed green space soil is important when making comparisons. Indeed, many green space soils are also influenced by urban land use and anthropogenic additions, whether directly or indirectly, and may also exhibit altered C stocks as a result (Ziter and Turner, 2018; Canedoli et al., 2020). Despite our results showing smaller soil C stocks in SU soil than green space soil (3.10 and 4.92 kg C m2 at 0–10 cm), our SU soil C stocks were still greater than other published sealed C stocks (reported in Sect. 4.1.1), and they were comparable to those reported for green spaces in Alabama, at 3.38 kg C m2 at 0–10 cm (Majidzadeh et al., 2017), and Nanjing City, at 4.52 kg C m2 at 0–20 cm (Wei et al., 2014b).
Due to the excavation of topsoil for road construction, the sealed soil studied is typically from deep in the soil profile. Deep urban soils beyond 50 cm depth are rarely studied, particularly so for sealed soils; however, they form a very large proportion of the urban soil profile and play an important role in urban soil C storage (Cambou et al., 2018; Vasenev and Kuzyakov, 2018). Our larger sealed soil C stocks may be a result of various factors. It has been suggested that sealing prevents decomposition due to sealed soil being isolated from the atmosphere and creating unfavourable conditions for microbes (Raciti et al., 2012; Piotrowska Długosz and Charzyński, 2015); thus, while sealing may isolate subsoil C stocks from litter inputs and decomposition, it does not necessarily deplete them (Vasenev and Kuzyakov, 2018). It is likely that the SU soil C stocks are a result of the high clay content of the sealed soil, as clay soils can provide high C stabilisation due to organo-mineral complexes which protect C from decomposition (Hassink, 1997; Six et al., 2002; Lorenz et al., 2008). However, the addition of anthropogenic C to road subbases, as previously discussed for SA soil, may also have contributed to the SU soil C stocks through transport of C. Little is known about the movement of water and dissolved nutrients through sealed soil, though it has been suggested that dissolved C may travel into sealed soils from more C-rich unsealed areas (Majidzadeh et al., 2017; Pereira et al., 2021), and dissolved charcoal is known to be mobilised in soils where there is water flow (Jaffé et al., 2013). Overall, the findings for SU soil C stocks support the argument that sealed subsoil C plays an important role in urban soil C storage and should be included in urban soil C assessments.
4.2 Nutrient stocks are reduced by sealing
Stocks of total N and total P were significantly smaller in both sealed soils compared to green space soil. The findings for N stocks corroborate those of other sealed studies, where N content and stocks have been consistency lower than in unsealed soils (Raciti et al., 2012; Wei et al., 2014a, b; Majidzadeh et al., 2017; Piotrowska-Długosz and Charzyński, 2015). This is potentially due to the loss of N as a result of topsoil removal during the sealing construction process and the consequent lack of litter inputs following sealing. The reduction in plant growth and organic matter inputs will lead to low levels of substrate and low rates of mineralisation and nitrification, impacting nutrient stocks. It has also been suggested that N content may be reduced in sealed soils due to aqueous losses of dissolved N, or gaseous losses as a result of denitrification (Raciti et al., 2012).
The C:N ratio was notably higher in SA soil than both other soils, likely as a result of additions of anthropogenic C, and correlation analysis showed there were significant positive relationships between C and N in both sealed soils and green space soil. This is in contrast to previous studies that found no relationship between C and N in sealed soil (Raciti et al., 2012; Majidzadeh et al., 2017), while one study found sealing led to a lower C:N ratio, with lower C explained by a lack of organic matter inputs, and a disruption in the relationship between C and N (Wei et al., 2014a). It has been suggested that sealing decouples C and N (Raciti et al., 2012; Wei et al., 2014a), an assertion which is supported by the C:N ratio seen here in SA soil; however, the strong positive correlation between C and N in both SU and SA soil suggest this decoupling may not always occur in sealed soils.
Studies of total P stocks are rare in sealed soils. We found that sealing significantly reduced total P stocks in both sealed soils compared to green space soil. These findings are contrary to other observations of P, where Olsen P concentration was greater in sealed soils (Wei et al., 2014b; Martinová et al., 2016), and P extracted using the Mehlich-1 method was greater in soils of crawl spaces beneath houses than in adjacent lawns (Majidzadeh et al., 2017, 2018). These observed increases have been explained by the absence of P uptake by plants and reduced loss of P by leaching or runoff (Wei et al., 2014b; Majidzadeh et al., 2018). In addition, P may be higher in some studies where sealing has occurred more recently, as urban green space soils can have high P contents (Qin et al., 2019); however, the length of time sealed was not included in this study. In a study in Poland, no difference in available P content was observed between sealed and unsealed soil, though semi-pervious soil did have a slightly lower P content (Piotrowska-Długosz and Charzyński, 2015). Our results may differ from other studies due to the difference in analysis method and the form of P analysed. In addition, some of the differences may be attributable to notable climatic differences between the study locations.
4.3 Anthropogenic additions influence nutrient dynamics
The SU soil had greater ammonium content and significantly lower nitrate content than green space soil, but the SA soil showed no difference in mineral N to the green space soil. The presence of ammonium in SU soil suggests that mineralisation had occurred in this soil to some degree; however, it is unknown whether this had occurred previously, utilising SOM leftover prior to sealing, or whether the process was ongoing. Potential N mineralisation has been observed to be significantly lower in sealed soil (Zhao et al., 2012), though some have found no significant difference in N mineralisation or inorganic N levels between sealed and unsealed soils (Wei et al., 2014b). It has been suggested that nutrient deficiency in sealed soils may stimulate microbes to decompose any available N into ammonium for their survival and that anaerobic conditions may promote the conversion of nitrate to ammonium by nitrate reductase or to N2O gas through denitrification (Norton and Stark, 2011; Raciti et al., 2012; Zhao et al., 2012). Ammonium can also accumulate in soil as a result of sorption to clay minerals (Nieder et al., 2011; Sahrawat, 2008; Weil and Brady, 2016), and exchangeable ammonium has been seen to increase following vegetation removal, perhaps due to reduced plant uptake, death of root material and decreased transpiration leading to more water movement in soil (Page, 2004), processes which may also occur in sealed soil.
Average nitrate content for green space and SA soils were similar to previous urban soil nitrate observations, which range from 3.3 mg kg−1 for bare soil in Beijing (Zhao et al., 2012) to 8.7 mg kg−1 for park soil in Leuven, Belgium (Martinová et al., 2016). There was variation between the two sealed soils, with SU soil having significantly lower nitrate content than green space soil and SA soil showing no significant difference to green space soil. In the SU soil, it is possible that sealing conditions restricted the growth of the microbial community (Lorenz and Lal, 2009), thus preventing nitrification. SU soils exhibited high bulk density, suggesting the soil is compacted and may be limited in oxygen. The presence of oxygen is a key control in nitrate production (Sahrawat, 2008; Weil and Brady, 2016), and compacted soils typically have lower aeration, soil moisture and reduced rates of nitrification (De Neve and Hofman, 2000). Our data for SU soil support findings from previous sealing studies, where nitrification and other microbial activities were notably reduced by sealing (Zhao et al., 2012; Wei et al., 2014b; Pereira et al., 2021). There is also the possibility that our low nitrate content was a result of losses due to denitrification or leaching of dissolved mineral N, as suggested by Raciti et al. (2012). The high pH of the SU soil and the presence of redoximorphic features exhibited by these samples both suggest reduction conditions and anoxic patches, within which denitrification could occur. As such, the low nitrate content in SU soil may have been due to reduced nitrification or increased denitrification, both of which can occur at low oxygen concentrations (Norton and Stark, 2011).
Conversely, the SA soil exhibited slightly higher nitrate content than SU soil and showed no difference to green space soil. This may be a result of numerous factors. Bulk density was lower in SA soil, suggesting improved aeration and greater oxygen levels than in the SU soil. This could lead to conditions sufficient for nitrification in SA soil, leading to the slightly higher nitrate levels, or, alternatively, to the reduction of denitrification conditions, which would lead to reduced nitrate losses as N2O gas. In addition, charcoal added to soil has been found to alleviate factors that inhibit nitrification (Abdelrahman et al., 2018), suggesting there may be benefits for nitrate levels from the anthropogenic additions of charcoal to the SA soil. As seen in our findings, soil nutrient dynamics within sealed soils remain largely unknown, highlighting the importance of further research into sealed soil processes and the potential effects of anthropogenic materials on these important soil functions.
This study has found a number of widespread effects of sealing on soil properties, carbon storage and nutrient dynamics. Soil properties were significantly affected by soil sealing, leading to higher pH, lower soil moisture and higher bulk density than in green space soils. This study has highlighted, for the first time, the potential importance of anthropogenic additions to soil carbon stocks under sealed surfaces. We found that anthropogenic additions of C-rich materials to sealed soils can lead to notably large soil C stocks, in some cases larger than green space soils, forming a legacy C store under sealed surfaces. This highlights that land development history is potentially an important control on urban soil C storage and its heterogeneity within, and between, cities. Our findings also indicate that this legacy C storage in sealed soils may include stable OC with long residence times as a result of historic OC additions in areas with an industrial past. However, further work into anthropogenic additions and the long-term OC stability and storage capabilities of sealed soil is needed to provide more detailed information. Further research into the effect of anthropogenic additions on OC stability and storage across the wider urban landscape, including green space and sealed soils, would provide a more complete picture of urban soil OC storage. Inorganic C also contributes to the legacy C store in sealed soils due to the weathering of minerals from concrete and calcareous materials, and the potential for atmospheric CO2 removal due to calcium carbonate production in sealed soils is another area in need of further investigation. Mineral N dynamics in sealed soils also appeared to be influenced by anthropogenic additions. This may have been a result of improved soil structure and conditions for nitrification, or it may have been a result of a lack of conditions leading to denitrification compared to relatively undisturbed sealed soils. Where sealed soils remained relatively undisturbed and altered by human additions, carbon, N and P stocks were all reduced. Overall, this study points to a need to understand how land development history influences sealed soil functioning and for further studies that advance our understanding of carbon stocks, carbon stability and nutrient dynamics in sealed soils.
The results of the analyses are summarised in Table A1, and examples of the sealed soil profiles with descriptions are set out in Fig. A1.
Table A1Urban soil measurements showing the mean (±SD). Different letters indicate a statistically significant difference between soil categories at the 0.05 level.
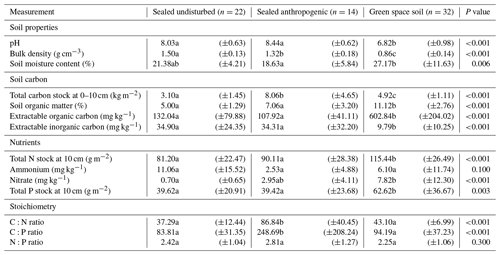
The data are available in the Lancaster University repository, with https://doi.org/10.17635/lancaster/researchdata/422 (O'Riordan 2021).
The concept and data collection were designed by RO'R, JD and CS; sample collection, lab analyses and data analyses were undertaken by RO'R. The article was prepared by RO'R with contributions from all co-authors.
Some authors are members of the editorial board of SOIL. The peer-review process was guided by an independent editor, and the authors have also no other competing interests to declare.
Publisher’s note: Copernicus Publications remains neutral with regard to jurisdictional claims in published maps and institutional affiliations.
This work was carried out as part of a Faculty of Science and Technology PhD at Lancaster University, in part supported by the UK Engineering and Physical Sciences Research Council, Living With Environmental Change Programme as part of the Soil-Value project (EP/N030532/1). We would like to thank colleagues at Lancaster Environment Centre for their support in the Plant-Soil Ecology lab, particularly Annette Ryan and Clare Benskin for providing support and advice throughout the duration of this study. We would also like to acknowledge our colleague Chris Boyko at ImaginationLancaster for their support through this project. We would also like to thank the companies that provided access to excavation sites for sealed samples: Beaumont Morgan, United Utilities, Balfour Beatty and Gallagher, as well as the councils of Greater Manchester for allowing access to sample in green spaces. Basemap data were supplied by Ordnance Survey. The soil type data were provided by the Soilscapes Viewer by LandIS © at Cranfield University.
This work was carried out as part of a Faculty of Science and Technology PhD at Lancaster University, in part supported by the UK Engineering and Physical Sciences Research Council, Living With Environmental Change Programme as part of the Soil-Value project (EP/N030532/1).
This paper was edited by Jerzy Weber and reviewed by two anonymous referees.
Abdelrahman, H., Hofmann, D., Berns, A. E., Meyer, N., Bol, R., and Borchard, N.: Historical charcoal additions alter water extractable, particulate and bulk soil C composition and stabilization, J. Plant Nutr. Soil Sc., 181, 809–817, https://doi.org/10.1002/jpln.201800261, 2018.
Artmann, M.: Assessment of Soil Sealing Management Responses, Strategies, and Targets Toward Ecologically Sustainable Urban Land Use Management, Ambio, 43, 530–541, https://doi.org/10.1007/s13280-014-0511-1, 2014.
Asabere, S. B., Zeppenfeld, T., Nketia, K. A., and Sauer, D.: Urbanization leads to increases in pH, carbonate, and soil organic matter stocks of arable soils of Kumasi, Ghana (West Africa), Frontiers in Environmental Science, 6, 119, https://doi.org/10.3389/fenvs.2018.00119, 2018.
Beerling, D. J., Kantzas, E. P., Lomas, M. R., Wade, P., Eufrasio, R. M., Renforth, P., Sarkar, B., Andrews, M. G., James, R. H., Pearce, C. R., Mercure, J.-F., Pollitt, H., Holden, P. B., Edwards, N. R., Khanna, M., Koh, L., Quegan, S., Pidgeon, N. F., Janssens, I. A., Hansen, J., and Banwart, S. A.: Potential for large-scale CO2 removal via enhanced rock weathering with croplands, Nature, 583, 242–248, https://doi.org/10.1038/s41586-020-2448-9, 2020.
Bradley, R., Milne, R., Bell, J., Lilly, A., Jordan, C., and Higgins, A.: A soil carbon and land use database for the United Kingdom, Soil Use Manage., 21, 363–369, 2005.
Bullock, P. and Gregory, P. J.: Soils in the urban environment, Blackwell Scientific Publications, Oxford, ISBN 9780632029884, 1991.
Cambou, A., Shaw, R. K., Huot, H., Vidal-Beaudet, L., Hunault, G., Cannavo, P., Nold, F., and Schwartz, C.: Estimation of soil organic carbon stocks of two cities, New York City and Paris, Sci. Total Environ., 644, 452–464, 2018.
Canedoli, C., Ferre, C., Abu El Khair, D., Padoa-Schioppa, E., and Comolli, R.: Soil organic carbon stock in different urban land uses: high stock evidence in urban parks, Urban Ecosyst., 23, 159–171, https://doi.org/10.1007/s11252-019-00901-6, 2020.
De Neve, S. and Hofman, G.: Influence of soil compaction on carbon and nitrogen mineralization of soil organic matter and crop residues, Biol. Fert. Soils, 30, 544–549, https://doi.org/10.1007/s003740050034, 2000.
Edmondson, J. L., Davies, Z. G., McHugh, N., Gaston, K. J., and Leake, J. R.: Organic carbon hidden in urban ecosystems, Sci. Rep.-UK, 2, 963, https://doi.org/10.1038/srep00963, 2012.
Edmondson, J. L., Davies, Z. G., Gaston, K. J., and Leake, J. R.: Urban cultivation in allotments maintains soil qualities adversely affected by conventional agriculture, J. Appl. Ecol., 51, 880–889, https://doi.org/10.1111/1365-2664.12254, 2014.
Edmondson, J. L., Stott, I., Potter, J., Lopez-Capel, E., Manning, D. A. C., Gaston, K. J., and Leake, J. R.: Black Carbon Contribution to Organic Carbon Stocks in Urban Soil, Envir. Sci. Technol., 49, 8339–8346, https://doi.org/10.1021/acs.est.5b00313, 2015.
EU: European Commission: Guidelines on best practice to limit, mitigate or compensate soil sealing, Luxembourg: European Union SWD (2012) 101, 2012.
FAO: World Reference Base for Soil Resources 2014, update 2015, International soil classification system for naming soils and creating legends for soil maps, Rome, 106, 2015.
FAO: Measuring and modelling soil carbon stocks and stock changes in livestock production systems: Guidelines for assessment (Version 1), Rome, FAO, 2018.
Hamilton, G. A. and Hartnett, H. E.: Soot black carbon concentration and isotopic composition in soils from an arid urban ecosystem, Org. Geochem., 59, 87–94, https://doi.org/10.1016/j.orggeochem.2013.04.003, 2013.
Hassink, J.: The capacity of soils to preserve organic C and N by their association with clay and silt particles, Plant Soil, 191, 77–87, https://doi.org/10.1023/A:1004213929699, 1997.
Heiri, O., Lotter, A. F., and Lemcke, G.: Loss on ignition as a method for estimating organic and carbonate content in sediments: reproducibility and comparability of results, J. Paleolimnol., 25, 101–110, https://doi.org/10.1023/A:1008119611481, 2001.
Herrmann, D. L., Shuster, W. D., and Garmestani, A. S.: Vacant urban lot soils and their potential to support ecosystem services, Plant Soil, 413, 45–57, https://doi.org/10.1007/s11104-016-2874-5, 2017.
Herrmann, D. L., Schifman, L. A., and Shuster, W. D.: Widespread loss of intermediate soil horizons in urban landscapes, P. Natl. Acad. Sci. USA, 115, 6751–6755, https://doi.org/10.1073/pnas.1800305115, 2018.
Jaffé, R., Ding, Y., Niggemann, J., Vähätalo, A. V., Stubbins, A., Spencer, R. G. M., Campbell, J., and Dittmar, T.: Global Charcoal Mobilization from Soils via Dissolution and Riverine Transport to the Oceans, Science, 340, 345, https://doi.org/10.1126/science.1231476, 2013.
Jorat, M. E., Goddard, M. A., Manning, P., Lau, H. K., Ngeow, S., Sohi, S. P., and Manning, D. A. C.: Passive CO2 removal in urban soils: Evidence from brownfield sites, Sci. Total Environ., 703, 135573, https://doi.org/10.1016/j.scitotenv.2019.135573, 2020.
Kachurina, O. M., Zhang, H., Raun, W. R., and Krenzer, E. G.: Simultaneous determination of soil aluminum, ammonium- and nitrate-nitrogen using 1 M potassium chloride extraction, Commun. Soil Sci. Plan., 31, 893–903, https://doi.org/10.1080/00103620009370485, 2000.
Kida, K. and Kawahigashi, M.: Influence of asphalt pavement construction processes on urban soil formation in Tokyo, Soil Sci. Plant Nutr., 61, 135–146, https://doi.org/10.1080/00380768.2015.1048182, 2015.
Kuzyakov, Y., Bogomolova, I., and Glaser, B.: Biochar stability in soil: Decomposition during eight years and transformation as assessed by compound-specific 14C analysis, Soil Biol. Biochem., 70, 229–236, https://doi.org/10.1016/j.soilbio.2013.12.021, 2014.
Lehmann, A.: Technosols and other proposals on urban soils for the WRB (World Reference Base for Soil Resources), Int. Agrophys., 20, 129–134, 2006.
Lehmann, A. and Stahr, K.: Nature and significance of anthropogenic urban soils, J. Soil. Sediment., 7, 247–260, 2007.
Lehmann, J., Abiven, S., Kleber, M., Pan, G., Singh, B. P., Sohi, S. P., and Zimmerman, A. R.: Persistence of biochar in soil, in: Biochar for environmental management, 2nd Edition, Earthscan, London, 233–280, https://doi.org/10.4324/9780203762264, 2015.
Li, G., Sun, G. X., Ren, Y., Luo, X. S., and Zhu, Y. G.: Urban soil and human health: a review, Eur. J. Soil Sci., 69, 196–215, 2018.
Lorenz, K. and Lal, R.: Biogeochemical C and N cycles in urban soils, Environ. Int., 35, 1–8, https://doi.org/10.1016/j.envint.2008.05.006, 2009.
Lorenz, K. and Lal, R.: Managing soil carbon stocks to enhance the resilience of urban ecosystems, Carbon Manag., 6, 35–50, https://doi.org/10.1080/17583004.2015.1071182, 2015.
Lorenz, K., Lal, R., and Shipitalo, M. J.: Chemical stabilization of organic carbon pools in particle size fractions in no-till and meadow soils, Biol. Fert. Soils, 44, 1043–1051, https://doi.org/10.1007/s00374-008-0300-8, 2008.
MacBride, S.: The archeology of coal ash: An industrial-urban solid waste at the dawn of the hydrocarbon economy, IA, Journal of the Society for Industrial Archeology, 39, 23–39, 2013.
Majidzadeh, H., Lockaby, B. G., and Governo, R.: Effect of home construction on soil carbon storage-A chronosequence case study, Environ. Pollut., 226, 317–323, 2017.
Majidzadeh, H., Lockaby, B. G., Price, R., and Governo, R.: Soil carbon and nitrogen dynamics beneath impervious surfaces, Soil Sci. Soc. Am. J., 82, 663–670, 2018.
Martinová, V., Van Geel, M., Lievens, B., and Honnay, O.: Strong differences in Quercus robur-associated ectomycorrhizal fungal communities along a forest-city soil sealing gradient, Fungal Ecol., 20, 88–96, 2016.
Morel, J. L., Chenu, C., and Lorenz, K.: Ecosystem services provided by soils of urban, industrial, traffic, mining, and military areas (SUITMAs), J. Soil. Sediment., 15, 1659–1666, https://doi.org/10.1007/s11368-014-0926-0, 2015.
Nieder, R., Benbi, D. K., and Scherer, H. W.: Fixation and defixation of ammonium in soils: a review, Biol. Fert. Soils, 47, 1–14, https://doi.org/10.1007/s00374-010-0506-4, 2011.
Norton, J. M. and Stark, J. M.: Chapter Fifteen – Regulation and Measurement of Nitrification in Terrestrial Systems, in: Methods in Enzymology, edited by: Klotz, M. G., Academic Press, 486, 343–368, https://doi.org/10.1016/B978-0-12-381294-0.00015-8, 2011.
Office for National Statistics: Estimates of the population for the UK, England and Wales, Scotland and Northern Ireland, Office for National Statistics, available at: https://www.ons.gov.uk/peoplepopulationandcommunity/ populationandmigration/populationestimates/datasets/population estimatesforukenglandandwalesscotlandandnorthern ireland , last access: 19 July 2021.
O'Riordan, R.: The effects of sealing on urban soil carbon and nutrients, Lancaster University, https://doi.org/10.17635/lancaster/researchdata/422, last access: February 2021.
Page, K., Dalal, R., Menzies, N. W., and Strong W.: Identification of the causes of subsoil ammonium accumulations in southeastern Queensland, SuperSoil 2004: 3rd Australian New Zealand Soils Conference, Symposium 9: Soil and farming systems, University of Sydney, Australia, available at: http://www.regional.org.au/au/asssi/supersoil2004/s9/poster/1485_pagek.htm (last access: 11 September 2020), 2004.
Pavao-Zuckerman, M.: Urbanization, Soils, and Ecosystem Services, in: Soil Ecology and Ecosystem Services, edited by: Wall DH, B. R., Behan-Pelletier V., Herrick, J. E., Jones, T. H., Ritz, K., Six, J., Strong, D. R., and van der Putten, W. H., Oxford University Press, Oxford, 270–281, 2012.
Pereira, M. C., O'Riordan, R., and Stevens, C.: Urban soil microbial community and microbial-related carbon storage are severely limited by sealing, J. Soil. Sediment., 21, 1455–1465, 2021.
Piotrowska-Długosz, A., and Charzyński, P.: The impact of the soil sealing degree on microbial biomass, enzymatic activity, and physicochemical properties in the Ekranic Technosols of Toruń (Poland), J. Soil. Sediment., 15, 47–59, 2015.
Pouyat, R. V., Yesilonis, I. D., and Nowak, D. J.: Carbon storage by urban soils in the United States, J. Environ. Qual., 35, 1566–1575, 2006.
Pouyat, R. V., Day, S. D., Brown, S., Schwarz, K., Shaw, R. E., Szlavecz, K., Trammell, T. L., and Yesilonis, I. D.: Urban Soils, in: Forest and Rangeland Soils of the United States Under Changing Conditions, Springer, Cham, 127–144, ISBN 978-3-030-45216-2, 2020.
Qin, G., Wu, J., Zheng, X., Zhou, R., and Wei, Z.: Phosphorus Forms and Associated Properties along an Urban–Rural Gradient in Southern China, Water, 11, 2504, https://doi.org/10.3390/w11122504, 2019.
Raciti, S. M., Groffman, P. M., Jenkins, J. C., Pouyat, R. V., Fahey, T. J., Pickett, S. T., and Cadenasso, M. L.: Accumulation of carbon and nitrogen in residential soils with different land-use histories, Ecosystems, 14, 287–297, 2011.
Raciti, S. M., Hutyra, L. R., and Finzi, A. C.: Depleted soil carbon and nitrogen pools beneath impervious surfaces, Environ. Pollut., 164, 248–251, 2012.
Rawlins, B. G., Vane, C. H., Kim, A. W., Tye, A. M., Kemp, S. J., and Bellamy, P. H.: Methods for estimating types of soil organic carbon and their application to surveys of UK urban areas, Soil Use Manage., 24, 47–59, https://doi.org/10.1111/j.1475-2743.2007.00132.x, 2008.
Rowland, A. P. and Grimshaw, H. M.: A wet oxidation procedure suitable for total nitrogen and phosphorus in soil, Commun. Soil Science Plan., 16, 551–560, https://doi.org/10.1080/00103628509367628, 1985.
Saha, U. K., Sonon, L., and Biswas, B. K.: A Comparison of Diffusion-Conductimetric and Distillation-Titration Methods in Analyzing Ammonium- and Nitrate-Nitrogen in the KCl-Extracts of Georgia Soils, Commun. Soil Sci. Plan., 49, 63–75, https://doi.org/10.1080/00103624.2017.1421647, 2018.
Sahrawat, K. L.: Factors Affecting Nitrification in Soils, Commun. Soil Sci. Plan., 39, 1436–1446, https://doi.org/10.1080/00103620802004235, 2008.
Scalenghe, R. and Marsan, F. A.: The anthropogenic sealing of soils in urban areas, Landscape Urban Plan., 90, 1–10, https://doi.org/10.1016/j.landurbplan.2008.10.011, 2009.
Schifman, L. A., Prues, A., Gilkey, K., and Shuster, W. D.: Realizing the opportunities of black carbon in urban soils: Implications for water quality management with green infrastructure, Sci. Total Environ., 644, 1027–1035, https://doi.org/10.1016/j.scitotenv.2018.06.396, 2018.
Setala, H., Francini, G., Allen, J. A., Jumpponen, A., Hui, N., and Kotze, D. J.: Urban parks provide ecosystem services by retaining metals and nutrients in soils, Environ. Pollut., 231, 451–461, https://doi.org/10.1016/j.envpol.2017.08.010, 2017.
Shaw, P. and Reeve, N.: Influence of a parking area on soils and vegetation in an urban nature reserve, Urban Ecosyst., 11, 107–120, https://doi.org/10.1007/s11252-007-0044-5, 2008.
Six, J., Conant, R. T., Paul, E. A., and Paustian, K.: Stabilization mechanisms of soil organic matter: Implications for C-saturation of soils, Plant Soil, 241, 155–176, https://doi.org/10.1023/A:1016125726789, 2002.
Soilscapes Viewer, Cranfield University, available at: http://www.landis.org.uk/soilscapes/ (last access: 8 October 2020), 2020.
United Nations: Department of Economic and Social Affairs, Population Division: World Urbanization Prospects 2018: Highlights, United Nations, New York, ISBN: 978-92-1-148318-5, 2019.
Vance, E. D., Brookes, P. C., and Jenkinson, D. S.: An extraction method for measuring soil microbial biomass C, Soil Biol. Biochem., 19, 703–707, 1987.
Vasenev, V. and Kuzyakov, Y.: Urban soils as hot spots of anthropogenic carbon accumulation: Review of stocks, mechanisms and driving factors, Land Degrad. Dev., 29, 1607–1622, https://doi.org/10.1002/ldr.2944, 2018.
Vasenev, V. I., Stoorvogel, J. J., and Vasenev, I. I.: Urban soil organic carbon and its spatial heterogeneity in comparison with natural and agricultural areas in the Moscow region, CATENA, 107, 96–102, https://doi.org/10.1016/j.catena.2013.02.009, 2013.
Vasenev, V. I., Van Oudenhoven, A. P. E., Romzaykina, O. N., and Hajiaghaeva, R. A.: The Ecological Functions and Ecosystem Services of Urban and Technogenic Soils: from Theory to Practice (A Review), Eurasian Soil Sci.+, 51, 1119–1132, https://doi.org/10.1134/s1064229318100137, 2018.
Washbourne, C. L., Renforth, P., and Manning, D. A. C.: Investigating carbonate formation in urban soils as a method for capture and storage of atmospheric carbon, Sci. Total Environ., 431, 166–175, https://doi.org/10.1016/j.scitotenv.2012.05.037, 2012.
Washbourne, C.-L., Lopez-Capel, E., Renforth, P., Ascough, P. L., and Manning, D. A. C.: Rapid Removal of Atmospheric CO2 by Urban Soils, Environ. Sci. Technol., 49, 5434–5440, https://doi.org/10.1021/es505476d, 2015.
Wei, Z., Wu, S., Yan, X., and Zhou, S.: Density and stability of soil organic carbon beneath impervious surfaces in urban areas, PloS One, 9, e109380, https://doi.org/10.1371/journal.pone.0109380, 2014a.
Wei, Z.-Q., Wu, S.-H., Zhou, S.-L., Li, J.-T., and Zhao, Q.-G.: Soil organic carbon transformation and related properties in urban soil under impervious surfaces, Pedosphere, 24, 56–64, 2014b.
Weil, R. and Brady, N.: The nature and properties of soils, Fifteenth edition, Pearson Education, Limited, Columbus, 1105 pp., ISBN 9781292162249, 2016.
Yang, J.-L. and Zhang, G.-L.: Formation, characteristics and eco-environmental implications of urban soils – A review, Soil Sci. Plant Nutr., 61, 30–46, https://doi.org/10.1080/00380768.2015.1035622, 2015.
Zhao, D., Li, F., Wang, R. S., Yang, Q. R., and Ni, H. S.: Effect of soil sealing on the microbial biomass, N transformation and related enzyme activities at various depths of soils in urban area of Beijing, China, J. Soil. Sediment., 12, 519–530, https://doi.org/10.1007/s11368-012-0472-6, 2012.
Zhao, D., Li, F., Yang, Q., Wang, R., Song, Y., and Tao, Y.: The influence of different types of urban land use on soil microbial biomass and functional diversity in Beijing, China, Soil Use Manage., 29, 230–239, https://doi.org/10.1111/sum.12034, 2013.
Ziter, C. and Turner, M. G.: Current and historical land use influence soil-based ecosystem services in an urban landscape, Ecol. Appl., 28, 643–654, https://doi.org/10.1002/eap.1689, 2018.