the Creative Commons Attribution 4.0 License.
the Creative Commons Attribution 4.0 License.
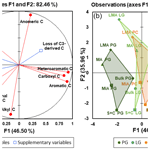
Land-use perturbations in ley grassland decouple the degradation of ancient soil organic matter from the storage of newly derived carbon inputs
Marco Panettieri
Denis Courtier-Murias
Cornelia Rumpel
Marie-France Dignac
Gonzalo Almendros
Abad Chabbi
In a context of global change, soil has been identified as a potential carbon (C) sink, depending on land-use strategies. To detect the trends in carbon stocks after the implementation of new agricultural practices, early indicators, which can highlight changes in short timescales, are required.
This study proposes the combined use of stable isotope probing and chemometrics applied to solid-state 13C nuclear magnetic resonance (NMR) spectra to unveil the dynamics of the storage and mineralization of soil carbon (C) pools. We focused on light organic matter fractions isolated by density fractionation of soil water stable aggregates because they respond faster to changes in land use than the total soil organic matter (SOM). Samples were collected from an agricultural field experiment with grassland, continuous maize cropping, and ley grassland under temperate climate conditions.
Our results indicated contrasting aggregate dynamics depending on land-use systems. Under our experimental conditions, grassland returns larger amounts of C as belowground inputs than maize cropping, evidencing a different distribution of light C fractions between aggregate classes. Coarse aboveground inputs from maize contributed mostly to larger macroaggregates. Land-use changes with the introduction of ley grassland provoked a decoupling of the storage and/or degradation processes after the grassland phase. The newly derived maize inputs were barely degraded during the first 3 years of maize cropping, whereas grassland-derived material was depleted. As a whole, results suggest large microbial proliferation as shown by 13C NMR under permanent grassland, then reduced within the first years after the land-use conversion, and finally restored. The study highlighted a fractal structure of the soil, determining a scattered spatial distribution of the cycles of storage and degradation of soil organic matter related to detritusphere dynamics. As a consequence, vegetal inputs from a new land use are creating new detritusphere microenvironments that may be disconnected from the dynamics of C cycle of the previous land use. The formation of those different and unconnected microenvironments may explain the observed legacy effect of the previous land use, since each microenvironment type contributes separately to the overall soil C cycle. The effects of the new land use on the soil C cycle are delayed until the different detritusphere microenvironments remain unconnected, and the ones from the previous land use represent the predominant microenvironment type. Increasing knowledge of the soil C dynamics at a fine scale will be helpful in refining the prediction models and land-use policies.
- Article
(3145 KB) - Full-text XML
-
Supplement
(321 KB) - BibTeX
- EndNote
Soil carbon (C) stocks represent the largest C pool of the terrestrial biosphere (Scharlemann et al., 2014) which is accumulated and released to the atmosphere and, to an extent, dependent on land-use and anthropogenic factors (Lal, 2004; Powlson et al., 2011). In fact, soil has the potential to store a large amount of C but also to emit great quantities of greenhouse gases (GHGs) depending on management practices (Lal, 2008; Smith, 2016). Agriculture is responsible for 20 % of the total GHG emissions, but the transformation of soil into a C sink with sustainable agricultural practices (Chenu et al., 2019) has been proposed as a promising mitigation strategy by researchers, international panels, and governments (IPCC, 2013; Lal, 2008; Minasny et al., 2017). These mitigation strategies need to be evaluated using adequate biomarkers that can decipher the stabilization and/or destabilization mechanisms and, in particular, the direction of the change in suitable land-use practices (Dignac et al., 2017; Wiesmeier et al., 2019) and refine the prediction models about C balance associated with land-use policies (Chenu et al., 2019).
Changes in land use affect soil C stocks on a timescale of years or decades; therefore, early modifications in soil organic matter (SOM) dynamics may be undetectable when the quantification of soil C content is performed on total soil rather than on reactive SOM pools (Castellano et al., 2015; Panettieri et al., 2017; Wiesmeier et al., 2019).
In a context of land-use change, the chemical characterization and stable isotope probing of SOM will establish biochemical decomposition patterns and C turnover rates, but the complex nature of SOM requires high-end analytical techniques (Derenne and Nguyen Tu, 2014) such as solid-state 13C nuclear magnetic resonance (NMR) or stable isotope probing (SIP). For soils that have experienced a land-use change with a conversion from C3 to C4 vegetation, the use of SIP is a valid method for measuring the turnover of bulk SOM (Balesdent et al., 1987; Dignac et al., 2005) and specific SOM pools (Bol et al., 2009; Matos et al., 2011; Yamashita et al., 2006). The turnover rate of SOM pools is also affected by the type and quality of the litter returned to the soil, which is land-use specific (Armas-Herrera et al., 2016; von Haden et al., 2019). However, the litter's chemical composition and its mineralization pattern are the two main proxies of SOM quality that can be assessed with solid-state 13C NMR (Baldock and Preston, 1995; Knicker et al., 2012). Solid-state 13C NMR analysis of chemically or physically isolated SOM pools has been used to evaluate the degradation status of SOM induced by land use (Rabbi et al., 2014) and agricultural management (Panettieri et al., 2013, 2014).
However, establishing an adequate field experiment to assess the effect of management practices on long-term C storage in the soil is not a trivial task. Most of the research on soils conducted at aggregate or molecular scales has been based on laboratory incubation conditions or with a limited experiment time (Dignac et al., 2017). Therefore, the extrapolation of those results to larger scales, longer intervals of time, and more diverse soil conditions (land uses and physical and chemical characteristics) is always arbitrary.
In this study we focused on the implementation of ley grassland rotations, which have been identified as a way to store carbon and provide ecosystem services (Kunrath et al., 2015; Lemaire et al., 2014). Few field studies have been targeted on the long-term effects of ley grassland on soil organic matter (SOM) dynamics (Crème et al., 2018; Panettieri et al., 2017; Solomon et al., 2007). The combination of two different land uses (namely grassland and maize cropping) in a 9 year ley grassland rotation produces differences in C contents at the arable layer that are only detectable at the aggregate scale, whereas bulk soil did not show significant change (Panettieri et al., 2017). Thus, to detect the magnitude and the direction of the land-use-dependant changes in SOM dynamics, SOM pools with a short turnover time are needed.
Consequently, the present research has been focused on the characterization of the light fraction (LF) of SOM (particulate organic matter), i.e. a fraction that has been identified as an early indicator of changes in land use having a faster turnover time than mineral-associated organic matter (Courtier-Murias et al., 2013; Leifeld and Kögel-Knabner, 2005; Panettieri et al., 2014). The present study has been designed to identify the processes affecting the labile soil C pools resulting from changes in land use. A temporary (ley) grassland system was compared with permanent grassland, permanent cropland, and bare fallow soils as controls, using a novel approach based on a combination of stable isotopes analysis and 13C NMR spectroscopy. To date, the literature reporting the combined use of SIP and 13C NMR on soil LF for assessing the effect of changes in land use on agricultural soils is scarce (Helfrich et al., 2006).
The hypothesis of this work is that the composition of LF provides early information about the direction and magnitude of the changes affecting SOM stocks in terms of accumulation or mineralization, which depend on the litter nature (above versus belowground biomass) and land-use characteristics (cropping versus grassland). To test this hypothesis, the chemical composition of LF, isolated from different water stable aggregates (Plaza et al., 2012), was characterized by solid-state 13C NMR spectroscopy. The obtained information was combined with measures of LF turnover in soil assessed by the natural abundance that 13C enrichment of SOM provided by the in situ labelling of maize crops in a 9-year field experiment in western France.
2.1 Experimental area
Soil samples were collected from the long-term experiment, namely “Systems of Observation and Experimentation in Environmental Research-Agro-ecosystem, Biogeochemical Cycles and Biodiversity (SOERE-ACBB)”, hosted at the INRAE Lusignan facilities (46∘25′12.91′′ N; 0∘07′29.35′′ E) in western France (Fig. 1).
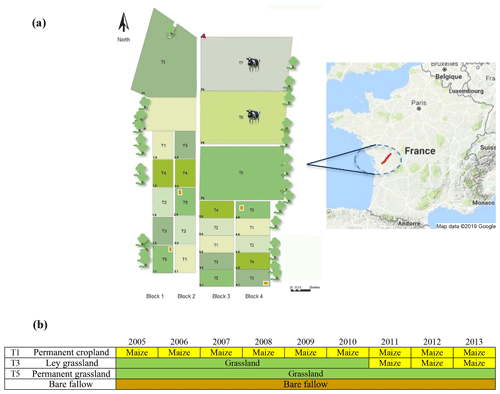
Figure 1National long-term observatory near Lusignan in the Nouvelle-Aquitaine region, France (a). Land-use management of target treatments from 2005 to 2013 used in this study before the sampling (b). Continuous maize bands were installed in the subplots of the T1 and T3 treatments in addition to bare fallow areas that have not received fresh organic matter input since the start of the experiment in 2005. Map data: ©2019 Google Maps.
The pedoclimatic characteristics of the studied area have been extensively described elsewhere (Chabbi et al., 2009; Moni et al., 2010). In summary, the area has a temperate climate with around 846 mm of annual precipitation and an average annual temperature of 11.9 ∘C. The soil texture of the upper soil horizons is a loamy Cambisol (130 g kg−1 sand, 692 g kg−1 silt, 177 g kg−1 clay), while lower soil horizons are clayed and rubefied, with a high content of kaolinite and oxides, classified as a Paleo-Ferralsol (103 g kg−1 sand, 612 g kg−1 silt, 286 g kg−1 clay). The soil bulk density was 1.48 g cm−3 (0–30 cm), with a pH (H2O) of 6.3 and 11 g kg−1 of organic carbon in the first 30 cm.
The long-term experiment started in 2005 on an area previously covered by oak forest and then devoted to agriculture or grassland for at least 100 years.
A total of four treatments, representing different land uses, were distributed on a 10 ha area with four replicates per treatment arranged in four randomized blocks (one replicate per treatment in each block of about 4000 m2). The following four different treatments were selected for sampling in the framework of the present experiment: (i) permanent crop (PC) rotations, (ii) permanent grassland (PG), (iii) ley grassland (LG; 6 years of grassland followed by 3 years of continuous cropping), and (iv) bare fallow (BF). To take advantage of the in situ 13C labelling of SOM induced by the maize plant inputs, only the subplots (500–700 m2) cultivated under maize (Zea mays L.) of PC and LG were sampled (9 years under continuous maize for PC and 6 years under grassland, followed by 3 years under maize for LG). Grassland plots were sown with three dominant species, namely Lolium perenne L. (cv. Milca), Festuca arundinacea Schreb. (cv. Soni), and Dactylis glomerata L. (cv. Ludac), and hay was harvested and exported three times per year. Before each maize growing cycle, soil was tilled with a mouldboard plough at 25–30 cm depth yearly, followed by minor tillage operations before maize sowing (one crop per year). All the treatments, except bare fallow subplots, were N fertilized. Grassland received between 170 and 380 kg N ha−1 yr−1 (on average 240 kg ha−1 yr−1), targeting the nitrogen nutrition index (NNI) between 0.9 and 1 (Lemaire et al., 2008). Maize crops were fertilized following local agronomic practices and received between 36 and 160 kg N ha−1 yr−1 (on average 98 kg N ha−1 yr−1). Finally, subplots of bare fallow were 54 m2 wide without any input from vegetation or fertilization.
2.2 Sampling and aggregate fractionation
In July 2014, 20 cm diameter stainless steel cylinders were used to collect soil samples, limiting aggregate disruption at a 0–30 cm depth, corresponding to the layer affected by tillage operations. A total of five soil cores were sampled for each plot at least 1 m from the edges (three cores in the case of bare fallow subplots) and immediately merged to obtain a composite sample. Further information about the sampling procedure was reported in a previous work (Panettieri et al., 2017).
The water content of bulk soil subsamples was determined gravimetrically right after the sampling, and then soil was dried at room temperature before fractionation.
Water stable aggregates were isolated from bulk soil samples, rewetted by slaking, following the method modified by Le Bissonnais (1996). Briefly, larger clods and plant debris (∅>7.1 mm) were discarded, and soil was left to slake for capillarity on a sand and/or kaolinite basin for 1 h. Successively, 50 g of soil was placed on a 2 mm mesh sieve, submerged in deionized water, and sieved for 2 min. The sieve was oscillated 50 times, with a vertical excursion of ∼3 cm. The soil suspension was passed through the other sieves to obtain four soil aggregate fractions, namely larger macroaggregates (LMAs; ∅ 2–7.1 mm), macroaggregates (MAs; ∅ 0.2–2 mm), microaggregates (MiAs; ∅ 0.05–0.2), and silt and clay-size aggregates (S + C; ∅<0.050 mm). On average, the mass recovery was 98 %, and not lower than 93 %, for all the samples. The mean weight diameter (MWD) for the four treatments was calculated following the method of van Bavel (1950), as follows:
in which n is the number of aggregate classes, fi is the relative abundance of the aggregate class, and is the arithmetic mean between the upper and lower limit of the aggregate class.
2.3 Isolation of light fractions of SOM
The light fraction (LF) was isolated as the floating fraction during wet sieving for each aggregate sample, including the free and occluded sub-fractions. LF was extracted from the soil samples, using the method described by Kölbl and Kögel-Knabner (2004) that was modified to fit the experimental conditions of this study. Briefly, 20 g of bulk soil samples or aggregate fractions were placed in a plastic vessel cooled by a water stream on the external walls to dissipate the heat. Samples were dispersed in 200 mL of a sodium polytungstate (SPT; 3Na2WO4•9WO3•H2O; MWD – 2986.01 g mol−1; Sigma-Aldrich) solution at a density of 1.8 g cm−3, using an ultrasonic probe (Vibra-Cell 75115; Scientific Bioblock) calibrated to apply a power of 450 J mL−1, as described by Poeplau and Don (2014). After sonication, vessels were allowed to settle down overnight and total LF (free and occluded) was separated from the mineral phase by centrifugation at 1000 g. The LF samples were recovered using a pressure filtration system on a cellulose-free membrane filter (0.45 µm pore size; Pall Life Science Supor® 450; Pall Corporation) and successively washed with deionized water to remove all the SPT residues until conductivity became lower than 5 µS cm−1. Finally, samples were freeze dried and adequately stored.
For the present work, bulk soil samples from three blocks were analysed individually for each treatment (for a total of 12 bulk soil samples), whereas, for aggregate fractions, field replicates were merged into a composite sample (one aggregate fraction per treatment for a total of 16 samples) to overcome constraints regarding the quantity of fractions recovered and NMR instrumental time.
2.4 Organic C and isotopic δ13C signature of samples
The experimental area was dominated by C3 vegetation, and the soil δ13C signature at the beginning of the experiment was −25 ‰ relative to Vienna Pee Dee Belemnite (VPDB) standard.
The determination of total organic C (TOC), total N (TN), and 13C isotopic signatures of all the LF samples was performed on dry aliquots using an isotopic ratio mass spectrometer (VG SIRA 10) coupled to an elemental carbon, hydrogen and nitrogen (CHN) analyser (NA 1500; Carlo Erba). The isotopic 13C ∕ 12C ratios (δ13C) were calibrated against the VPDB standard and expressed with Eq. (2), as follows:
The turnover of LF carbon (LF-C) was quantified using the 13C enrichment induced by the maize crops in plots under permanent cropland and ley grassland as described by Balesdent and Mariotti (1996), simplified as described by Dignac et al. (2005), in Eq. (3), as follows:
in which LFC refers to total C quantity within the soil LF-C, and LFCnew refers to C quantity within the LF-C derived from the new maize vegetation.
For the isotopic ratios (δ), the subscript soilM stands for the soil δ13C measured for the two plots under maize (9 years of maize for permanent cropland and 3 years of maize after ley grassland), soilG stands for permanent grassland controls under continuous C3 vegetation, and newM and newG stand for the isotopic composition of maize and grass vegetal material. To estimate the term (δnewM−δnewG), the difference in isotopic composition between plant materials corrected for above- and belowground inputs to the soil was used, as calculated in a previous study on the same experimental farm (Panettieri et al., 2017). Using the values of F, the percentage of C3-derived organic matter remaining in samples from ley grassland and permanent cropland was calculated with reference to the permanent grassland samples. Similarly, degradation of LF in the absence of new vegetal inputs was calculated from bare fallow samples. This approach produced an index of C3-LF persistence under different land uses.
2.5 Solid-state 13C nuclear magnetic resonance
The 13C NMR analyses were carried out on a Bruker Avance 400 spectrometer operating at a 13C frequency of 100.6 MHz, employing a 13C ramped amplitude cross-polarization single pulse (CPSP) sequence under magic angle spinning (MAS) conditions (collectively Ramp-CPSP/MAS). This sequence was first introduced by Shu et al. (2010) in material sciences, then successfully applied by Courtier-Murias et al. (2014) on environmental samples. Spectra of LF samples obtained with a standard cross-polarization (Ramp-CP/MAS) sequence with an equal number of scans (i.e. the same acquisition time) were compared at the beginning of the experiment (Fig. S1 in the Supplement). Preliminary experiments showed that Ramp-CPSP/MAS outperformed Ramp-CP/MAS (hereafter CP) in terms of the signal-to-noise ratio by a factor of ∼2 in spectral regions with a lower proton density, i.e. the aromatic region. Therefore, only Ramp-CPSP/MAS (hereafter CPSP) analyses were carried out for the whole sample set.
Approximately 50–100 mg of samples were placed into a zirconium oxide rotor with a diameter of 4 mm and sealed with Kel-F® caps. For all the measurements, a spinning speed of 10 kHz was applied, the contact time was set to 1 ms, and the recycle delay was 3 s; this value was higher than in our previous works (Courtier-Murias et al., 2014) due to the technical specifications of the NMR probe used in this study. About 5000–10 000 scans were accumulated for each sample, and a ramped 1H pulse was used during Hartmann–Hahn contact to circumvent Hartmann–Hahn mismatches. The spectra were divided in eight main regions; assignments for carbon resonances are reported in Table 1, according to Knicker and Lüdemann (1995) and Knicker (2011).
2.6 Statistical analyses
A Shapiro–Wilk test was used to check data normality before further analyses were conducted. For bulk soil and LF isolated from bulk soil samples, the significance of the differences found for the variables (P≤0.05) induced by the four land uses was assessed by the nonparametric Kruskal–Wallis tests and the Dunn's multiple pairwise comparisons. For the NMR analyses, the significance was assessed on bulk soil samples, whereas, on composite samples, the significance was not assessed due to the lack of replicate measurements. The significance level of Spearman's correlation coefficient (ρs) between the measured variables was assessed at a significance level of P≤0.05. A principal component analysis (PCA) was used to explore how the chemical composition assessed by NMR affected the sample distribution by group. Since integrals of NMR regions are compositional data (their sum is the total measured intensity), the data were pretreated to reduce the effect of collinearity, using the principles of Aitchison's geometry and the centre logratio transformed (CLR) prior to the PCA calculation (Aitchison, 1982). Total organic carbon (TOC), carbon to nitrogen ratios (C∕N), and C3-derived LF-C losses were used as supplementary variables. Statistical analyses were carried out using XLSTAT (Addinsoft, Boston, USA, available at https://www.xlstat.com, last access: 5 March 2020).
A chemometrics approach was used to treat NMR spectra and obtain information about the relative contribution of C3 and C4-derived organic matter to LF fractions. Spectra were exported as a two-column matrix, reporting the chemical shift (516 points corresponding to about 2 points per parts per million – ppm) and absolute intensity for each point. Afterwards, normalized intensities (If) were calculated to overcome the different C contents of each sample that may lead to different signal-to-noise ratios for each spectrum following Eqs. (4) and (5).
Measured intensities at each point (In) were divided by the total spectrum intensity (It) calculated as the sum of the intensities for each point (n=516) and then multiplied by an arbitrary factor of 10 000 to keep the normalized intensities within a range of −2 to 40.
Normalized intensities were used to obtain scaled spectra, namely one for each sample. Afterwards, the samples of permanent grassland were chosen as reference spectra (free of C4-derived SOM and with continuous vegetal inputs), whereas spectra from other treatments were subtracted from the corresponding permanent grassland sample spectra. This leads to a graphical view on changes in LF originating from different land uses, with positive signals for the regions in which permanent grassland had higher relative contributions than the subtracted treatment and negative signal for the opposite situation.
3.1 Carbon contents in total soil and light fractions
The LF-C contents represented between 7 % and 30 % of the TOC for bulk soil and aggregates (Fig. 2), in line with results of other studies (Leifeld and Kögel-Knabner, 2005). No significant differences in the relative contributions of LF-C to TOC between the four treatments were highlighted for bulk soil samples (Fig. 2). Larger macroaggregates showed the highest LF-C contribution among the fractions (Fig. 2), and trends towards higher relative contributions of LF-C to TOC were found for permanent cropland samples compared to the corresponding fractions of the other treatments. Ley grassland induced a decrease in the LF-C content of the bulk soil, the larger macroaggregates, and the microaggregate fractions, if compared with permanent grassland and permanent cropland homologous fractions (Fig. 2). Bearing in mind the relative contribution of LF-C to TOC, LF-C has been proposed as being an early indicator of the changes affecting soil quality, due to the faster turnover of LF-C than TOC, which is normally on a time span of years (Poeplau et al., 2018). This useful characteristic has been proposed to detect changes in C stocks modulated by land use against the large background of TOC that is not affected (Leifeld and Kögel-Knabner, 2005). The LF-C content under ley grassland decreased to levels comparable to those measured for bare fallow in which no vegetal inputs were returned to soil during the previous 9 years. We attribute these results to the effects of soil perturbation due to the switch from a grassland soil ecosystem to a crop soil ecosystem. Maize cropping includes deep tillage operations and provides a different type of vegetal input; grassland is characterized by an extended, dense, and relatively shallow root system, whereas maize roots are more spaced and deeper (Jackson et al., 1996).
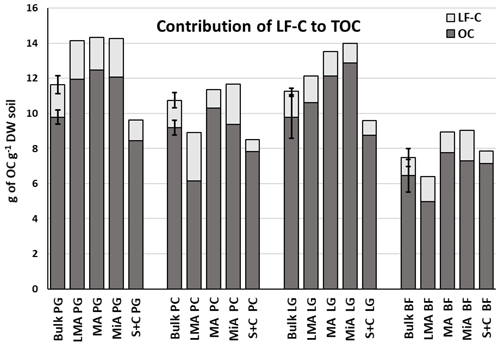
Figure 2Light fraction carbon (LF-C) contribution to total organic carbon (TOC) in bulk soil (n=3) and density fractions (n=1) for different treatments (PG – permanent grassland; PC – permanent cropping; LG – ley grassland; and BF – bare fallow soil). No significant differences between treatments (P<0.05) for bulk samples were found. Error bars show the calculated standard deviations for replicate samples.
On the one hand, cropping produces changes in soil aggregation (Álvaro-Fuentes et al., 2008; Bronick and Lal, 2005) which are associated with LF-C degradation due to the increase in microbial activity (Courtier-Murias et al., 2013; Panettieri et al., 2014) and changes in physical protection that soil aggregates provide to the land-use-specific proportions of LF-C (Leifeld and Kögel-Knabner, 2005).
On the other hand, previous studies on the same experimental area reported that grassland total inputs to soil were higher than those from maize crops, but the type and distribution of those inputs presented meaningful differences (Panettieri et al., 2017; Armas-Herrera et al., 2016). Maize returned a larger proportion of aboveground biomass, successively incorporated into soil during tillage, and a lower percentage of root-derived material, whereas grassland provides a large proportion of belowground inputs to soil in a more extended area.
Despite the higher number of tillage operations performed under ley grassland and permanent cropland, the MWD was significantly higher for permanent cropland compared with bare fallow (Table 2). No significant differences were found between permanent cropland and the two grassland systems due to large data dispersion, even if a trend towards a lower MWD for ley grassland and permanent grassland was highlighted (Table 2). Given that grassland returns a larger amount of belowground inputs than maize crops to the soil, we can suggest that such an incorporation takes place at the level of smaller size aggregates richer in C, compared to the incorporation into coarser aggregates of C originating from maize crops.
Table 2C to N ratios, mean weight diameter (MWD), and δ13C signature measured for the bulk soil and aggregate fractions of four treatments. Significant differences between treatments (P<0.05) for bulk soil samples, according to the Kruskal–Wallis test, are indicated with different bold letters. Note: LMA – larger macroaggregate; MA – macroaggregate; MiA – microaggregate; and S + C – silt and clay.
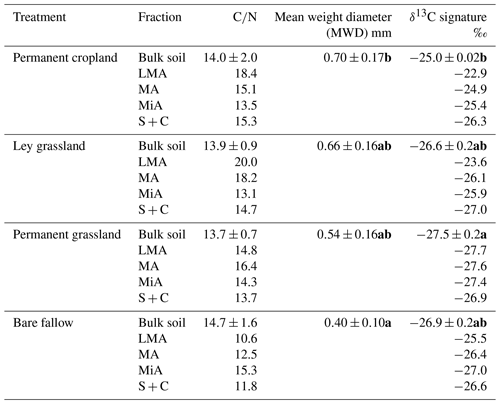
3.2 Local proxies of soil organic matter dynamics
The use of stable isotope probing allowed us to distinguish the percentages of C4-derived material proceeding from maize vegetal inputs from the C3-derived material in the LF-C extracted from soils under ley grassland and permanent cropland (Fig. 3). The latter showed higher proportions of C4-derived LF-C than ley grassland within all the aggregate fractions, due to the longer time cropped under maize. LF-C from the silt and clay fraction was mostly composed of C3-derived C, less than 5 % of new C was found for permanent cropland, and no new inputs were detected for ley grassland. The contribution of new C in the LF increased with the aggregate size for all the fractions of the permanent cropland; 31 % of LF-C in larger macroaggregates of permanent cropland was maize derived, evidencing the faster turnover of larger aggregates, which has been extensively described in the literature and corresponds to the preferential accumulation of particulate, slightly decomposed, LF in coarse soil fractions (Puget et al., 1995, 2000; Tisdall and Oades, 1982). The contribution of maize-derived material increased with aggregate size for permanent cropland, whereas a different trend was observed for ley grassland treatment. The contribution of new C to the LF of larger macroaggregates was similar to that of permanent cropland; however, the contributions of maize-derived C to the LF-C of macroaggregates and microaggregates of ley grassland were very similar, representing a break in the linear pattern found for ley grassland (Fig. 3).
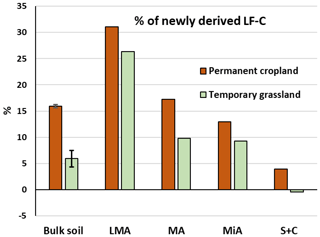
Figure 3Percentage of maize-derived (C4) light fraction carbon (LF-C) for bulk soils and aggregate fractions under permanent cropland and ley grassland. Note: LMA – larger macroaggregate; MA – macroaggregate; MiA – microaggregate; and S + C – silt and clay. Values of S + C for ley grassland were slightly lower (−0.4 %) than those found for permanent grassland, probably due to field variability. Error bars show the calculated standard deviations for replicate samples.
Taking into account the total amount of LF-C (C3 and C4 derived), Fig. 4 shows that the larger macroaggregates and microaggregates of ley grassland contained the lowest amount of LF-C if compared with all the treatments, which is a different trend to that observed for permanent cropland. These results showed that 3 years of continuous maize cropping, following 6 years of grassland, produced a severe disruption and/or rearrangement of C pools. The measured losses of LF-C under ley grassland were not supported by similar losses of TOC; therefore, the tillage operations and maize cropping may have led to the redistribution of this C, favouring its incorporation into heavier-mineral-associated C pools (Basile-Doelsch et al., 2009). Such LF-C losses are not attributable to maize cropping, since the observed trends for soils under permanent maize suggested that longer periods of maize cropping will restore the depleted LF-C of larger macroaggregates and microaggregates. Moreover, losses of C3-derived LF-C were not registered for plots under permanent maize. Only two pools of LF-C have been partitioned using the 13C in situ labelling, but we cannot exclude that the C3-derived LF-C is, in turn, composed of different land-use-specific pools accumulated before the establishment of the experiment (DeGryze et al., 2005; Meyer et al., 2012). Those pools may have been more susceptible to alteration by the land-use changes from grassland to maize but not to continuous maize cropping. Since no further isotopic partitioning is possible on the LF-C accumulated before the beginning of the experiment, the chemical composition of LF-C pools assessed by solid-state 13C NMR will be used to provide further insights about the effect of land-use change on C stocks.
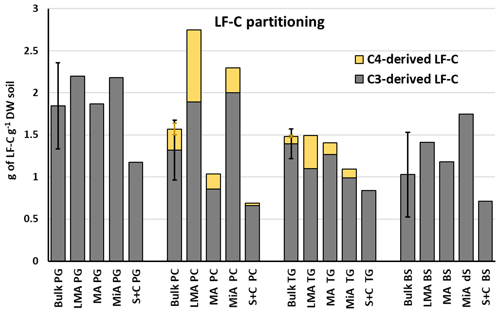
Figure 4C3 and C4 light fraction C contributions to total light fraction C for different treatments and soil fractions. Note: PG – permanent grassland; LG – ley grassland; PC – permanent cropland; BF – bare fallow soil; LMA – larger macroaggregate; MA – macroaggregate; MiA – microaggregate; and S + C – silt and clay. No significant differences between treatments (P<0.05) were found for bulk soil samples. Error bars show the calculated standard deviations for replicate samples.
3.3 Performance of the NMR method
To test the performance of the CPSP sequence against the more commonly used CP sequence, two spectra from the same sample were acquired using the same number of scans for both experiments. Figure S1 shows that CPSP sequences were able to detect a higher intensity of the signal for aromatic C in comparison with the CP sequence, with negligible modifications detected for the other regions. This is due to the lower proton density in condensed aromatic moieties that can lead to a less effective polarization transfer from proton to carbon nuclei. In CP experiments, an increase in the contact time for transferring the magnetization along the distance between the condensed aromatic C and the closest proton could be used as a solution for overcoming this problem. However, longer contact times are also correlated with losses of signal intensity mediated by the spin-lattice relaxation, which will produce lower signal intensity (Knicker, 2011). The extra 13C pulse of the CPSP sequence allows us to measure 13C atoms of the condensed aromatic moieties that are far from protons better and, therefore, improve the signal-to-noise ratio of this region, especially when their 13C NMR T1 relaxation values are short (Courtier-Murias et al., 2014). In addition, some differences for CH2 groups in the non-crystalline poly(methylene) and carbonyls groups have also been detected (Courtier-Murias et al., 2014). However, CPSP always equals or improves CP performance, even for soils with a low aromaticity, as confirmed for our comparison spectra. Consequently, CPSP was therefore selected as the standard sequence for this study.
Table 3Integration values (expressed as a percent of the total spectral area) and signal-to-area ratios of the main regions of 13C NMR spectra from soils under contrasting agricultural management with ( ± ) standard deviations. Significant differences between treatments (P<0.05) for field replicates of bulk soil samples (n=3), according to the Kruskal–Wallis test, are indicated with different bold letters.
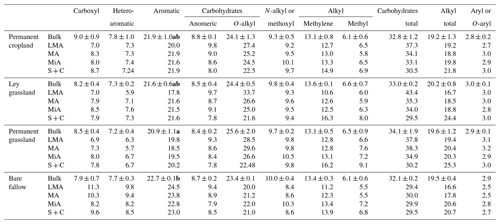
Standard deviations of the calculated areas for the field replicates (three different blocks) of bulk soil samples were lower than 1.35 % for all the integrated regions, except for the O-alkyl C region of permanent grassland (2.05 %). This showed that the variability due to spatial conditions and sample preparation was reasonably low, and the integrated areas of the spectra from the composite samples are valid enough to be interpreted in terms of differences in SOM in the different aggregate fractions.
Contributions from carboxyl C and N-alkyl C were constant (7 %–9 % and 9 %–10 %, respectively) for all the measured samples, with the exception of bare fallow samples, in which carboxyl C accounted for more than 10 % in larger macroaggregates and microaggregates. The region assigned to N-alkyl C may also represent the typical signal assigned to methoxyl C of lignin structures (Lüdemann and Nimz, 1973). Signal intensity in the N-alkyl region showed a significantly positive Spearman's correlation with the total N content (ρs=0.647, P<0.05) and a significantly negative correlation with the intensity of the heteroaromatic C region (, P<0.05), suggesting that the N-alkyl signal is derived mainly from C in proteinaceous material rich in N rather than methoxyl C. The persistence of protein-derived material in SOM pools has been described in other studies (Diekow et al., 2005; Nannipieri and Eldor, 2009; Panettieri et al., 2014), and it has been used to characterize LF as “new” SOM, rich in fresh litter, but also exoenzymes and cytoplasmic material from microbial biomass and necromass (Miltner et al., 2012).
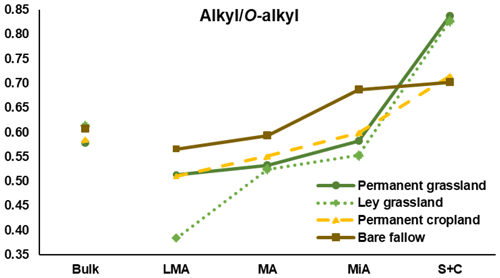
Figure 5The alkyl to O-alkyl ratios calculated for light fraction C isolated from bulk soils and aggregate fractions for the four treatments. Note: LMA – larger macroaggregate; MA – macroaggregate; MiA – microaggregate; and S + C – silt and clay.
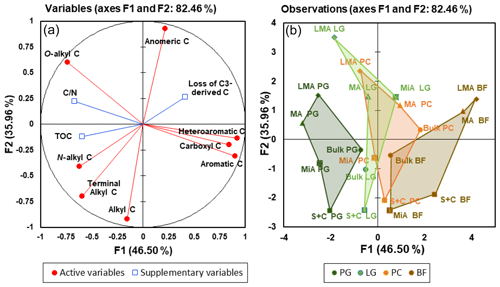
Figure 6Results of principal component analysis applied to 13C NMR analysis of the C distribution within different aggregate fractions from soils under different land uses. Projected loadings of the soil-measured variables (a) and representation of the light fraction C isolated from aggregate size fractions of the four different treatments on the plain, as defined by the two first principal components (b). Labels for samples and variables refer to Tables 1, 2, and 3.
The alkyl to O-alkyl C ratio is commonly used as a proxy of SOM degradation (Baldock and Preston, 1995). Isotopic results showed how larger aggregates contain fresher LF-C, confirmed by the decrease in the alkyl ∕ O-alkyl with the increase in aggregate size. Fresh litter is richer in carbohydrates from cellulose, for which anomeric C resonates in the O-alkyl region, whereas litter in a comparatively more advanced stage of degradation is characterized by the progressive enhancement of the alkyl C signal, suggesting the selective enrichment of long-chain and condensed aliphatic structures, including cutins and suberins from higher plants, or phospholipids from microbial and fungal biomass (Miltner et al., 2012; Panettieri et al., 2013). In this study, the presence of a clear peak of the terminal methyl group that accounted for half of the intensity of the methylene group indicated that most of the total alkyl C intensity is due to chains shorter than those expected for cutins and suberins. Alkyl C contribution was higher for smaller fractions, probably explained, for this experiment, by the microbial growth stimulated by the diffusion into fine pores of smaller molecules released during the enzymatic breakdown of macromolecules (Ludwig et al., 2015). Similar results were found by Clemente et al. (2011), in which combined analyses of solution-state 1H NMR and diffusion-edited 1H NMR unveiled that the alkyl contribution to the fine fraction of soils under prairie is mainly ascribed to microbial synthesis rather than preservation of plant material. However, the preservation of plant-derived aliphatic short chains adsorbed on mineral surfaces of soil fine particles could not be excluded (Basile-Doelsch et al., 2015).
The larger macroaggregates of the three treatments with vegetal inputs were characterized by a high contribution of O-alkyl C; in the case of ley grassland, 43 % of the total C intensity measured by NMR was assigned to carbohydrates, a value close to that of the non-degraded plant tissue. Figure 5 shows the alkyl ∕ O-alkyl ratios for the four treatments. Trends along the aggregate fractions evidenced how ley grassland had similar ratios than those of permanent grassland for fine fractions, but a totally different ratio for larger macroaggregates, suggesting that this fraction is mainly composed of coarse vegetal material derived from the recently established maize crops.
3.4 Chemical composition of the soil organic matter pools from different land uses
At this point, the interpretation of the relative abundances of each of the compound classes obtained from the 13C NMR spectra and the quantification of LF-C turnover using in situ labelling provided by maize constitutes a valid and original approach for understanding how land use affects the dynamics of SOM pools within different aggregate compartments.
A PCA analysis was performed to represent the differences in LF isolated from aggregate fractions of four treatments based on the relative abundance of NMR compound classes (Fig. 6). In the plane defined by the two first components (82.5 % variance explained), the four treatments could be differentiated along the first component, while the second component ordered the samples by aggregate size (i.e. coarser fractions on the top of the plot and finer fractions on the bottom). The observations corresponding to permanent grassland fractions were clearly placed on the left of the plot, on the opposite side of the bare fallow ones. Two out of four fraction classes (namely macroaggregates and silt and clay) may be connected with a quasi-horizontal line from left to right, following the order of permanent grassland, ley grassland, permanent cropland, and bare fallow, whereas larger macroaggregates and microaggregates had a more scattered distribution. Looking at the position of the different treatments, the permanent cropland hull was partially overlapped with the hulls described by ley grassland and with the bulk soil of bare fallow. Ley grassland presented the greatest scattering along the second component, meaning that the chemical composition of aggregates was highly different, and it evidenced a prominent shift from the original grassland footprint to the cropland footprint. Similar changes occurred in the molecular composition of SOM under ley grassland studied by analytical pyrolysis (Rumpel et al., 2009). This result demonstrated that 13C NMR analyses of LF-C may be useful for detecting changes in SOM quality due to land use on a short-term timescale, since this shift of ley grassland samples through a permanent cropland footprint was not detected with analyses performed on total SOM from bulk soil on the same experimental area (Crème et al., 2018; Panettieri et al., 2017).
The active variables of the PCA were split into two groups; on the right side, a cluster formed by the variables of aromatic, O-aryl, and carboxyl C was correlated to the first component and had a higher relative contribution to the observations under bare fallow for which no vegetal inputs were returned for 9 years. This cluster can be interpreted as an advanced status of degradation of the organic matter in the LF associated with the land use (Leifeld and Kögel-Knabner, 2005). On the left, and more scattered along the second component axis, the variables of O-alkyl, N-alkyl, alkyl, and terminal alkyl reflected the different composition of SOM based on the different proportion of microbial-derived SOM and maize-derived and grassland-derived inputs.
The exploration of PCA indicated that the largest distances between homologous fractions of different land uses were found for larger macroaggregates and microaggregates, showing that the four land uses caused chemical differences of a larger magnitude within those fractions. The supplementary variables were most effectively described by the 13C NMR regions of O-alkyl, N-alkyl, and terminal alkyl plus the degradation status cluster on the right side of the plot.
The supplementary variables of TOC and C∕N were placed on the left part of the graph; TOC was correlated to the area described by the permanent grassland observation and the N-alkyl C, whereas C∕N was correlated to the larger macroaggregates and O-alkyl C. Losses of C3-derived LF-C were placed on the right of the plot, closely correlated to the area of the graph described by bare fallow and perfectly opposite (thus negatively correlated) to N-alkyl C and terminal alkyl.
The fact that losses of LF-C were correlated to the N cycle (in this case, the 13C NMR signal attributed to proteinaceous material) and to the intensity of the terminal methyl group attributed to microbial aliphatic material agrees with recent findings about the stoichiometric relationships controlling the microbial degradation of vegetal litter (Chen et al., 2019; Sinsabaugh et al., 2013). As a result, the progressive mineralization of fresh litter induces a higher contribution of microbial-derived C to SOM and a possible redistribution of the C through heavier fractions of SOM. These dynamics appear to be land-use and aggregate size dependent.
3.5 The effect of land use on the degradation status of organic matter pools
The spectral subtractions, with respect to permanent grassland samples, were used to define selective losses (positive values) or gains (negative values) in the 13C NMR intensities for each land use (Fig. 7).
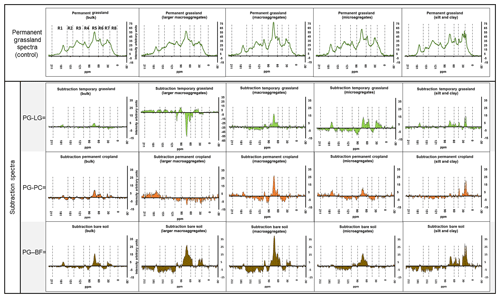
Figure 7Comparisons of 13C NMR spectra based on subtractions of the spectra obtained from ley grassland (LG), permanent cropland (PC), and bare fallow (BF) samples from the homologous spectra obtained from permanent grassland (PG; first row, used as control). Spectral regions include carboxyl C (R1), heteroaromatic (R2), aromatic (R3), anomeric (R4), O-alkyl (R5), N-alkyl (R6), alkyl (R7), and terminal alkyl (R8). All the intensities of all the resulting spectra were normalized by an arbitrary factor (10 000) to fit within the interval of −2 to +40 arbitrary units on the graphs. The y axes of all the graphs were scaled to the same interval of the arbitrary units, except for LMAs of ley grassland.
The results highlighted that LF isolated from bulk soil, and most of the aggregate fractions of permanent grassland had a higher contribution of O-alkyl C if compared with homologous samples from the other treatments, with the exception of larger macroaggregates in ley grassland and permanent cropland. This trend was compensated with an enrichment in the aromatic, heteroaromatic, and carboxylic regions with respect to the intensities registered for permanent grassland soils. When a soil under grassland is left bare for 9 years, the quantity of LF-C decreased, and the 13C NMR intensity assigned to the carbohydrates of LF-C decreased with the size of the aggregates (Panettieri et al., 2014; Plaza et al., 2013; Six et al., 2004). With the exception of larger macroaggregate fractions, the magnitude of these effects increased from ley grassland and permanent cropland to bare fallow, clearly indicating that the LF-C of the treatments under maize presented a more degraded status than those under permanent grassland. The LF-C isolated from the silt and clay fraction of permanent grassland had also higher intensities in alkyl and terminal alkyl regions. The data also suggest that the LF-C of the silt and clay fraction suffers minor changes related to land use in terms of quantity, but the 13C NMR signal intensity attributed to alkyl decreases for this fraction when the soil is either cultivated under maize or left bare. The fact that bare fallow presents a similar trend to lower alkyl contributions than treatments cultivated under maize confirms that this result is mostly attributable to the losses of microbial-derived C, presumably rich in short aliphatic chains, rather than to contributions of plant-derived short aliphatic chains, as explained above.
The presence of higher proportions of carbohydrate-derived material in LF-C isolated from large aggregates and the corresponding higher contributions of microbial C in fine fractions agrees with the literature describing the size-dependant reactivity of the aggregates (Puget et al., 1995; Six et al., 2000; Tisdall and Oades, 1982). Large aggregates contain high amounts of fresh plant material, rich in carbohydrates, that is preferentially degraded by exoenzymes (Baldock and Preston, 1995), the so-called detritusphere. Moreover, aggregates contain the by-products of the enzymatic breakdown of macromolecules, which tend to diffuse into finer pores, sustaining the higher microbial proliferation in finer fractions (Courtier-Murias et al., 2013; Ludwig et al., 2015; Miltner et al., 2012). When not enough macromolecules are degraded in coarser fractions (i.e. lower contribution or lower degradation of polysaccharides), the flux of by-products could be interrupted, and this proliferation is not sustained anymore (Plaza et al., 2013). However, when a different vegetal input is returned to the soil after changing land use (from grassland to permanent maize cropping), losses in the alkyl C intensity of LF-C for the silt and clay fraction were also registered for ley grassland and permanent cropland. This could be indicating that the location and the type of input had an influence on the alkyl C contribution to this fraction, supporting the idea that alkyl moieties found in this fraction are microbial-derived material (Eclesia et al., 2016). After 3 years of maize cropping, LF-C from ley grassland showed a higher proportion of carbohydrate-derived material (O-alkyl) in the larger macroaggregates and a higher contribution of maize-derived LF-C, as assessed by isotopic analyses. Therefore, land-use-specific characteristics of the maize phase, such as the new type of vegetal input, a different root network, or the tillage operation, were responsible for a change in the quantity and chemical composition of the LF-C from larger macroaggregates under ley grassland (von Haden et al., 2019).
Table 4 summarizes the specific behaviours of SOM degradation found for LFC isolated from different aggregate fractions of ley grassland. For LF-C extracted from the other fractions of ley grassland, the O-alkyl intensities were lower than those of permanent grassland, showing that grassland-derived material is being degraded under maize cropping. A similar trend of O-alkyl losses in comparison to permanent grassland was detected for permanent cropland, with the exception of the larger macroaggregate fraction in which the O-alkyl intensities were similar in the two treatments. Therefore, we can infer that (i) maize inputs rich in carbohydrates are mostly deposited as surface litter (Panettieri et al., 2017), (ii) maize cropping tends to increase aggregates of MWD compared to grassland, and (iii) surface litter lasts in LF-C of larger macroaggregates as non-degraded material 3 years after land-use change, but a degradation and/or a redistribution to heavier soil fractions is expected on a timescale of 9 years.
Table 4Summary of the characteristics of light fraction C isolated from bulk soil and aggregates of ley grassland. A decoupling between SOM dynamics within larger aggregates and fine fractions is highlighted.
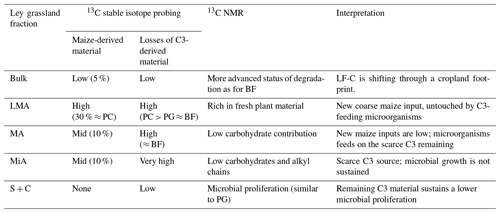
On the other hand, grassland provides higher belowground inputs, resulting in higher amounts of LF-C but, in this case, a lower MWD. The trend to higher aggregates of MWD when maize is implemented into the crop rotation may be explained by the differences between the most abundant input types under maize and grassland (i.e. coarse maize-derived aboveground inputs and grassland belowground ones). Large contributions of grassland-derived belowground inputs rich in vegetal macromolecules are progressively degraded and redistributed from larger to finer aggregates, and that may be feeding and sustaining the microbial growth found in the finest fraction of permanent grassland. As soon as grassland is substituted by maize, the changes in litter traits may hamper this flux of nutrients, and the contribution of microbial C to LF-C decreases. Under our experimental conditions, maize-derived belowground inputs are less abundant and, with different root traits compared to grassland (Panettieri et al., 2017; Armas-Herrera et al., 2016), a larger proportion of coarse aboveground input is returned to the soil and left “untouched” until a new type of detritusphere ecosystem is built around it, and the new equilibrium will be reached (Kumar et al., 2016; Kuzyakov and Blagodatskaya, 2015). In fact, the O-alkyl C contribution to the silt and clay fraction of permanent cropland is higher than that found for ley grassland, demonstrating that the 13C NMR signal attributed to carbohydrates is restored within a longer timescale.
Comparison of the trends of the three vegetated treatments with those found for bare fallow shows that, despite the new inputs being returned to the soil under maize, the change in land use in ley grassland will provoke the disruption of the equilibrium reached under the previous grassland cover. Some of the aggregate fractions of ley grassland will continue to function if the land-use change did not happen, showing a degradation of LF-C in terms of quantity of quality similar to that registered for bare fallow. This may be explained by the fact that land-use-specific microenvironments are not adapted to the degradation of the new vegetal inputs because of their different chemical characteristics and/or different spatial arrangements (Castellano et al., 2015; Eclesia et al., 2016). Later on, the newly built aggregates and microenvironments under the new phase of the rotation will become the majority of the total soil matrix, switching the net soil functionality to the new land use. We suggest that this may be one of the explanations for the so-called “legacy effect”, described as the influence of previous land uses on the soil C recovery and/or loss dynamics after the establishment of a new soil management (Compton et al., 1998; Smith, 2014). Moreover, the optimum for soil microbial diversity and soil C storage tends to happen at an optimum level of soil perturbation and land-use switch following a humped-back curve, different for each type of soil (Acosta-Martínez et al., 2008; Tardy et al., 2015). For our study, 3 years of maize cropping in ley grassland affected the LF-C dynamics in a way that is similar to that observed for bare fallow. This is clearly an alert signal that C stocks accumulated under grassland may be hampered by future cropping years (Sleutel et al., 2006). Nevertheless, C losses were not observed for bulk soil (Crème et al., 2018), and longer times under maize tended to restore LF-C pools and increase the MWD but with an overall loss of C stocks (Panettieri et al., 2017). Therefore, refining the data on the land use, depending on C persistence in soil, may be helpful for deciding which land-use rotations will be the most suitable for C storage strategies (Rumpel et al., 2019).
This study provides new insights that unveil the land-use dependency of the storage and degradation dynamics that affect reactive C pools. Our findings indicate that C under ley grassland is subjected to two different and mostly independent mechanisms, namely the degradation regarding the grassland-derived LF-C and the accumulation of new maize-derived LF-C. Considering the difference between grassland species and maize plants, we assume that the root architecture of the rhizosphere contributed to the change in the chemical nature and spatial distribution of the vegetal inputs returned to soil.
We found evidence that these factors regulated by land use led to the formation of land-use-specific detrituspheres in ley grassland, each of them with specific LF-C dynamics of redistribution among different pools and mineralization. The microbial proliferation suggested by 13C NMR for LF-C accumulated during the grassland phase is not sustained during the maize phase; it is as if the microenvironments and microbial communities were not sensitive to the new maize inputs returned as coarse material. As a whole, results showed that grassland-derived LF-C continues to be degraded as if no inputs were returned to soil, whereas maize-derived material is slowly degraded. We expect that longer maize cropping times will establish a new equilibrium among LF-C isolated from aggregates.
Agricultural intensification in ley grassland provokes, firstly, a decrease in soil LF-C, then a depletion of total soil C contents. The analytical characterization of LF-C is proposed here as a way to evaluate the impact of crop rotations at shorter timescales, before the soil C contents are hampered. This study enables us to generate sufficient evidence and develop an understanding of C dynamics at the fine scale to devise soil organic carbon (SOC) model predictions and policies to sustain C storage under land-use practices.
The data generated in the framework of the Systems of Observation and Experimentation in Environmental Research-Agro-ecosystem, Biogeochemical Cycles and Biodiversity (SOERE-ACBB) observatory are freely available and accessible after the validation of a specific request from the responsible scientist. The data that support the findings of this study are available from the corresponding and lead authors, upon reasonable request.
The supplement related to this article is available online at: https://doi.org/10.5194/soil-6-435-2020-supplement.
MP, AC, CR, and MFD conceptualized this paper. AC, CR, MP, and GA curated the data. A formal analysis of the findings was conducted by MP, DCM, MFD, CR, and GA, while AC, CR, and MP acquired the funds. The investigation was led by MP and DCM, who also contributed to the methodology together with input from CR and MFD. AC, MP, and CR administered the project, while AC, CR, MFD, and GA acquired the resources. The software was developed by DCM, GA, and MP. Moreover, MP, CR, and AC supervised the project, while AC, CR, and MFD validated the findings. This paper was visualized by MP and GA, with MP writing the original version of the paper, and DCM, GA, MFD, CR, and AC contributing to the writing and review of the paper.
The authors declare that they have no conflict of interest.
We are deeply indebted to Xavier Charrier for his technical support in the experimental area of Systems of Observation and Experimentation in Environmental Research–Agro-ecosystems, Biogeochemical Cycles, and Biodiversity (SOERE–ACBB), and to Valerie Pouteau, Cyril Girardin, and Daniel Billiou for their technical support in the analyses carried out.
This research has been supported by the AgreenSkills fellowship programme under the EU Seventh Framework Programme (grant nos. FP7-26719 and ExpeER 262060), AnaEE France (grant no. ANR-11-INBS-0001), and the IR-RMN-THC (grant no. Fr3050) CNRS.
This paper was edited by Boris Jansen and reviewed by two anonymous referees.
Álvaro-Fuentes, J., López, M. V., Cantero-Martinez, C., and Arrúe, J. L.: Tillage effects on soil organic carbon fractions in Mediterranean dryland agroecosystems, Soil Sci. Soc. Am. J., 72, 541–547, https://doi.org/10.2136/sssaj2007.0164, 2008.
Armas-Herrera, C. M., Dignac, M. F., Rumpel, C., Arbelo, C. D., and Chabbi, A.: Management effects on composition and dynamics of cutin and suberin in topsoil under agricultural use, Eur. J. Soil Sci., 67, 360–373, https://doi.org/10.1111/ejss.12328, 2016.
Baldock, J. A. and Preston, C. M.: Chemistry of carbon decomposition processes in forests as revealed by solid-state carbon-13 nuclear magnetic resonance, in: Carbon forms and functions in forest soils, edited by: Kelly, J. M. and McFee, W. W., 89–117, Soil Science Society of America, Madison, WI, 1995.
Balesdent, J. and Mariotti, A.: Measurement of soil organic matter turnover using 13C natural abundance, in: Mass spectrometry of soils, edited by: Boutton, T. W. and Yamasaki, S. I., 83–111, Marcel Dekker, New York (USA)., 1996.
Balesdent, J., Mariotti, A., and Guillet, B.: Natural 13C abundance as a tracer for studies of soil organic matter dynamics, Soil Biol. Biochem., 19, 25–30, https://doi.org/10.1016/0038-0717(87)90120-9, 1987.
Basile-Doelsch, I., Balesdent, J., and Rose, J.: Are Interactions between Organic Compounds and Nanoscale Weathering Minerals the Key Drivers of Carbon Storage in Soils?, Environ. Sci. Technol., 49, 3997–3998, https://doi.org/10.1021/acs.est.5b00650, 2015.
Bol, R., Poirier, N., Balesdent, J., and Gleixner, G.: Molecular turnover time of soil organic matter in particle-size fractions of an arable soil, Rapid Commun. Mass Sp., 23, 2551–2558, https://doi.org/10.1002/rcm.4124, 2009.
Bronick, C. J. and Lal, R.: Soil structure and management: A review, Geoderma, 124, 3–22, 2005.
Castellano, M. J., Mueller, K. E., Olk, D. C., Sawyer, J. E., and Six, J.: Integrating plant litter quality, soil organic matter stabilization, and the carbon saturation concept, Glob. Change Biol., 21, 3200–3209, https://doi.org/10.1111/gcb.12982, 2015.
Chabbi, A., Kögel-Knabner, I., and Rumpel, C.: Stabilised carbon in subsoil horizons is located in spatially distinct parts of the soil profile, Soil Biol. Biochem., 41, 256–261, https://doi.org/10.1016/j.soilbio.2008.10.033, 2009.
Chen, J., Seven, J., Zilla, T., Dippold, M. A., Blagodatskaya, E., and Kuzyakov, Y.: Microbial C : N : P stoichiometry and turnover depend on nutrients availability in soil: A 14C, 15N and 33P triple labelling study, Soil Biol. Biochem., 131, 206–216, https://doi.org/10.1016/j.soilbio.2019.01.017, 2019.
Chenu, C., Angers, D. A., Barré, P., Derrien, D., Arrouays, D., and Balesdent, J.: Increasing organic stocks in agricultural soils: Knowledge gaps and potential innovations, Soil Till. Res., 188, 41–52, https://doi.org/10.1016/j.still.2018.04.011, 2019.
Clemente, J. S., Simpson, A. J., and Simpson, M. J.: Association of specific organic matter compounds in size fractions of soils under different environmental controls, Org. Geochem., 42, 1169–1180, https://doi.org/10.1016/j.orggeochem.2011.08.010, 2011.
Compton, J. E., Boone, R. D., Motzkin, G., and Foster, D. R.: Soil carbon and nitrogen in a pine-oak sand plain in central Massachusetts: Role of vegetation and land-use history, Oecologia, 116, 536–542, https://doi.org/10.1007/s004420050619, 1998.
Courtier-Murias, D., Simpson, A. J., Marzadori, C., Baldoni, G., Ciavatta, C., Fernández, J. M., López-de-Sá, E. G., and Plaza, C.: Unraveling the long-term stabilization mechanisms of organic materials in soils by physical fractionation and NMR spectroscopy, Agr. Ecosyst. Environ., 171, 9–18, https://doi.org/10.1016/J.AGEE.2013.03.010, 2013.
Courtier-Murias, D., Farooq, H., Longstaffe, J. G., Kelleher, B. P., Hart, K. M., Simpson, M. J., and Simpson, A. J.: Cross polarization-single pulse/magic angle spinning (CPSP/MAS): A robust technique for routine soil analysis by solid-state NMR, Geoderma, 226–227, 405–414, https://doi.org/10.1016/j.geoderma.2014.03.006, 2014.
Crème, A., Rumpel, C., Le Roux, X., Romian, A., Lan, T., and Chabbi, A.: Ley grassland under temperate climate had a legacy effect on soil organic matter quantity, biogeochemical signature and microbial activities, Soil Biol. Biochem., 122, 203–210, https://doi.org/10.1016/j.soilbio.2018.04.018, 2018.
De Gryze, S., Six, J., Brits, C., and Merckx, R.: A quantification of short-term macroaggregate dynamics: Influences of wheat residue input and texture, Soil Biol. Biochem., 37, 55–66, https://doi.org/10.1016/j.soilbio.2004.07.024, 2005.
Derenne, S. and Nguyen Tu, T. T.: Characterizing the molecular structure of organic matter from natural environments: An analytical challenge, CR Geosci., 346, 53–63, https://doi.org/10.1016/j.crte.2014.02.005, 2014.
Diekow, J., Mielniczuk, J., Knicker, H., Bayer, C., Dick, D. P., and Kögel-Knabner, I.: Carbon and nitrogen stocks in physical fractions of a subtropical Acrisol as influenced by long-term no-till cropping systems and N fertilisation, Plant Soil, 268, 319–328, 2005.
Dignac, M. F., Bahri, H., Rumpel, C., Rasse, D. P., Bardoux, G., Balesdent, J., Girardin, C., Chenu, C., and Mariotti, A.: Carbon-13 natural abundance as a tool to study the dynamics of lignin monomers in soil: An appraisal at the Closeaux experimental field (France), Geoderma, 128, 3–17, https://doi.org/10.1016/j.geoderma.2004.12.022, 2005.
Dignac, M.-F., Derrien, D., Barré, P., Barot, S., Cécillon, L., Chenu, C., Chevallier, T., Freschet, G. T., Garnier, P., Guenet, B., Hedde, M., Klumpp, K., Lashermes, G., Maron, P.-A., Nunan, N., Roumet, C., and Basile-Doelsch, I.: Increasing soil carbon storage: mechanisms, effects of agricultural practices and proxies. A review, Agron. Sustain. Dev., 37, 14, https://doi.org/10.1007/s13593-017-0421-2, 2017.
Eclesia, R. P., Jobbagy, E. G., Jackson, R. B., Rizzotto, M., and Piñeiro, G.: Stabilization of new carbon inputs rather than old carbon decomposition determines soil organic carbon shifts following woody or herbaceous vegetation transitions, Plant Soil, 409, 99–116, https://doi.org/10.1007/s11104-016-2951-9, 2016.
Helfrich, M., Ludwig, B., Buurman, P., and Flessa, H.: Effect of land use on the composition of soil organic matter in density and aggregate fractions as revealed by solid-state 13C NMR spectroscopy, Geoderma, 136, 331–341, https://doi.org/10.1016/j.geoderma.2006.03.048, 2006.
IPCC: Climate Change 2013: The Physical Science Basis. Contribution of Working Group I to the Fifth Assessment Report of the Intergovernmental Panel on Climate Change, edited by: Qin, D., Plattner, G.-K., Tignor, M., Allen, S. K., Boschung, J., Nauels, A., Xia, Y., Bex, V., and Midgley, P. M., Cambridge University Press, Cambridge, United Kingdom and New York, NY, USA, 2013.
Jackson, R. B., Canadell, J., Ehleringer, J. R., Mooney, H. A., Sala, O. E., and Schulze, E. D.: A global analysis of root distributions for terrestrial biomes, Oecologia, 108, 389–411, https://doi.org/10.1007/bf00333714, 1996.
Knicker, H.: Solid state CPMAS 13C and 15N NMR spectroscopy in organic geochemistry and how spin dynamics can either aggravate or improve spectra interpretation, Org. Geochem., 42, 867–890, 2011.
Knicker, H. and Lüdemann, H. D.: N-15 and C-13 CPMAS and solution NMR studies of N-15 enriched plant material during 600 days of microbial degradation, Org. Geochem., 23, 329–341, 1995.
Knicker, H., Nikolova, R., Dick, D. P., and Dalmolin, R. S. D.: Alteration of quality and stability of organic matter in grassland soils of Southern Brazil highlands after ceasing biannual burning, Geoderma, 181–182, 11–21, 2012.
Kölbl, A. and Kögel-Knabner, I.: Content and composition of free and occluded particulate organic matter in a differently textured arable Cambisol as revealed by solid-state 13C NMR spectroscopy, J. Plant Nutr. Soil Sc., 167, 45–53, 2004.
Kumar, A., Kuzyakov, Y., and Pausch, J.: Maize rhizosphere priming: field estimates using 13C natural abundance, Plant Soil, 409, 87–97, https://doi.org/10.1007/s11104-016-2958-2, 2016.
Kunrath, T. R., de Berranger, C., Charrier, X., Gastal, F., de Faccio Carvalho, P. C., Lemaire, G., Emile, J. C., and Durand, J. L.: How much do sod-based rotations reduce nitrate leaching in a cereal cropping system?, Agr. Water Manage., 150, 46–56, https://doi.org/10.1016/j.agwat.2014.11.015, 2015.
Kuzyakov, Y. and Blagodatskaya, E.: Microbial hotspots and hot moments in soil: Concept & review, Soil Biol. Biochem., 83, 184–199, https://doi.org/10.1016/J.SOILBIO.2015.01.025, 2015.
Lal, R.: Soil carbon sequestration to mitigate climate change, Geoderma, 123, 1–22, https://doi.org/10.1016/J.GEODERMA.2004.01.032, 2004.
Lal, R.: Sequestration of atmospheric CO2 in global carbon pools, Energ. Environ. Sci., 1, 86–100, 2008.
Le Bissonnais, Y.: Aggregate stability and assessment of soil crustability and erodibility: I. Theory and methodology – Stabilité structurale et évaluation de la sensibilité des sols à la battance et à l'érosion: I: Théorie et méthologie, Eur. J. Soil Sci., 47, 425–437, https://doi.org/10.1111/j.1365-2389.1996.tb01843.x, 1996.
Leifeld, J. and Kögel-Knabner, I.: Soil organic matter fractions as early indicators for carbon stock changes under different land-use?, Geoderma, 124, 143–155, https://doi.org/10.1016/j.geoderma.2004.04.009, 2005.
Lemaire, G., Jeuffroy, M. H., and Gastal, F.: Diagnosis tool for plant and crop N status in vegetative stage. Theory and practices for crop N management, Eur. J. Agron., 28, 614–624, https://doi.org/10.1016/j.eja.2008.01.005, 2008.
Lemaire, G., Franzluebbers, A., Carvalho, P. C. de F., and Dedieu, B.: Integrated crop-livestock systems: Strategies to achieve synergy between agricultural production and environmental quality, Agr. Ecosyst. Environ., 190, 4–8, https://doi.org/10.1016/j.agee.2013.08.009, 2014.
Lüdemann, H. D. and Nimz, H.: Carbon 13 nuclear magnetic resonance spectra of lignins, Biochem. Biophys. Res. Commun., 52, 1162–1169, 1973.
Ludwig, M., Achtenhagen, J., Miltner, A., Eckhardt, K.-U., Leinweber, P., Emmerling, C., and Thiele-Bruhn, S.: Microbial contribution to SOM quantity and quality in density fractions of temperate arable soils, Soil Biol. Biochem., 81, 311–322, https://doi.org/10.1016/j.soilbio.2014.12.002, 2015.
Matos, E. S., Freese, D., Mendonça, E. S., Slazak, A., and Hüttl, R. F.: Carbon, nitrogen and organic C fractions in topsoil affected by conversion from silvopastoral to different land use systems, Agroforest. Syst., 81, 203–211, https://doi.org/10.1007/s10457-010-9314-y, 2011.
Meyer, S., Leifeld, J., Bahn, M., and Fuhrer, J.: Land-use change in subalpine grassland soils: Effect on particulate organic carbon fractions and aggregation, J. Plant Nutr. Soil Sci., 175, 401–409, https://doi.org/10.1002/jpln.201100220, 2012.
Miltner, A., Bombach, P., Schmidt-Brücken, B., and Kästner, M.: SOM genesis: Microbial biomass as a significant source, Biogeochemistry, 111, 41–55, 2012.
Minasny, B., Malone, B. P., McBratney, A. B., Angers, D. A., Arrouays, D., Chambers, A., Chaplot, V., Chen, Z.-S., Cheng, K., Das, B. S., Field, D. J., Gimona, A., Hedley, C. B., Hong, S. Y., Mandal, B., Marchant, B. P., Martin, M., McConkey, B. G., Mulder, V. L., O'Rourke, S., Richer-de-Forges, A. C., Odeh, I., Padarian, J., Paustian, K., Pan, G., Poggio, L., Savin, I., Stolbovoy, V., Stockmann, U., Sulaeman, Y., Tsui, C.-C., Vågen, T.-G., van Wesemael, B., and Winowiecki, L.: Soil carbon 4 per mille, Geoderma, 292, 59–86, https://doi.org/10.1016/J.GEODERMA.2017.01.002, 2017.
Moni, C., Rumpel, C., Virto, I., Chabbi, A., and Chenu, C.: Relative importance of sorption versus aggregation for organic matter storage in subsoil horizons of two contrasting soils, Eur. J. Soil Sci., 61, 958–969, https://doi.org/10.1111/j.1365-2389.2010.01307.x, 2010.
Nannipieri, P. and Eldor, P.: The chemical and functional characterization of soil N and its biotic components, Soil Biol. Biochem., 41, 2357–2369, 2009.
Panettieri, M., Knicker, H., Berns, A. E. E., Murillo, J. M. M., and Madejón, E.: Moldboard plowing effects on soil aggregation and soil organic matter quality assessed by 13C CPMAS NMR and biochemical analyses, Agr. Ecosyst. Environ., 177, 48–57, https://doi.org/10.1016/j.agee.2013.05.025, 2013.
Panettieri, M., Knicker, H., Murillo, J. M., Madejón, E., and Hatcher, P. G.: Soil organic matter degradation in an agricultural chronosequence under different tillage regimes evaluated by organic matter pools, enzymatic activities and CPMAS 13C NMR, Soil Biol. Biochem., 78, 170–181, https://doi.org/10.1016/j.soilbio.2014.07.021, 2014.
Panettieri, M., Rumpel, C., Dignac, M.-F., and Chabbi, A.: Does grassland introduction into cropping cycles affect carbon dynamics through changes of allocation of soil organic matter within aggregate fractions?, Sci. Total Environ., 576, 251–263, https://doi.org/10.1016/j.scitotenv.2016.10.073, 2017.
Plaza, C., Fernández, J. M., Pereira, E. I. P., and Polo, A.: A comprehensive method for fractionating soil organic matter not protected and protected from decomposition by physical and chemical mechanisms, Clean – Soil, Air, Water, 40, 134–139, 2012.
Plaza, C., Courtier-Murias, D., Fernández, J. M., Polo, A., and Simpson, A. J.: Physical, chemical, and biochemical mechanisms of soil organic matter stabilization under conservation tillage systems: A central role for microbes and microbial by-products in C sequestration, Soil Biol. Biochem., 57, 124–134, https://doi.org/10.1016/j.soilbio.2012.07.026, 2013.
Poeplau, C. and Don, A.: Effect of ultrasonic power on soil organic carbon fractions, J. Plant Nutr. Soil Sci., 177, 137–140, https://doi.org/10.1002/jpln.201300492, 2014.
Poeplau, C., Don, A., Six, J., Kaiser, M., Benbi, D., Chenu, C., Cotrufo, M. F., Derrien, D., Gioacchini, P., Grand, S., Gregorich, E., Griepentrog, M., Gunina, A., Haddix, M., Kuzyakov, Y., Kühnel, A., Macdonald, L. M., Soong, J., Trigalet, S., Vermeire, M. L., Rovira, P., van Wesemael, B., Wiesmeier, M., Yeasmin, S., Yevdokimov, I., and Nieder, R.: Isolating organic carbon fractions with varying turnover rates in temperate agricultural soils – A comprehensive method comparison, Soil Biol. Biochem., 125, 10–26, https://doi.org/10.1016/j.soilbio.2018.06.025, 2018.
Powlson, D. S., Gregory, P. J., Whalley, W. R., Quinton, J. N., Hopkins, D. W., Whitmore, A. P., Hirsch, P. R., and Goulding, K. W. T.: Soil management in relation to sustainable agriculture and ecosystem services, Food Policy, 36, S72–S87, https://doi.org/10.1016/J.FOODPOL.2010.11.025, 2011.
Puget, P., Chenu, C., and Balesdent, J.: Total and young organic matter distributions in aggregates of silty cultivated soils, Eur. J. Soil Sci., 46, 449–459, 1995.
Puget, P., Chenu, C., and Balesdent, J.: Dynamics of soil organic matter associated with particle-size fractions of water-stable aggregates, Eur. J. Soil Sci., 51, 595–605, https://doi.org/10.1046/j.1365-2389.2000.00353.x, 2000.
Rabbi, S. M. F., Linser, R., Hook, J. M., Wilson, B. R., Lockwood, P. V., Daniel, H., and Young, I. M.: Characterization of Soil Organic Matter in Aggregates and Size-Density Fractions by Solid State 13C CPMAS NMR Spectroscopy, Commun. Soil Sci. Plan., 45, 1523–1537, https://doi.org/10.1080/00103624.2014.904335, 2014.
Rumpel, C., Chabbi, A., Nunan, N., and Dignac, M. F.: Impact of landuse change on the molecular composition of soil organic matter, J. Anal. Appl. Pyrol., 85, 431–434, https://doi.org/10.1016/j.jaap.2008.10.011, 2009.
Rumpel, C., Amiraslani, F., Chenu, C., Garcia Cardenas, M., Kaonga, M., Koutika, L. S., Ladha, J., Madari, B., Shirato, Y., Smith, P., Soudi, B., Soussana, J. F., Whitehead, D., and Wollenberg, E.: The 4p1000 initiative: Opportunities, limitations and challenges for implementing soil organic carbon sequestration as a sustainable development strategy, Ambio, 49, 350–360, https://doi.org/10.1007/s13280-019-01165-2, 2019.
Scharlemann, J. P., Tanner, E. V., Hiederer, R., and Kapos, V.: Global soil carbon: understanding and managing the largest terrestrial carbon pool, Carbon Manag., 5, 81–91, https://doi.org/10.4155/cmt.13.77, 2014.
Shu, J., Li, P., Chen, Q., and Zhang, S.: Quantitative Measurement of Polymer Compositions by NMR Spectroscopy:Targeting Polymers with Marked Difference in Phase Mobility, Macromolecules, 43, 8993–8996, https://doi.org/10.1021/ma101711f, 2010.
Sinsabaugh, R. L., Manzoni, S., Moorhead, D. L., and Richter, A.: Carbon use efficiency of microbial communities: stoichiometry, methodology and modelling, Ecol. Lett., 16, 930–939, https://doi.org/10.1111/ele.12113, 2013.
Six, J., Paustian, K., Elliott, E. T., and Combrink, C.: Soil structure and organic matter: I. Distribution of aggregate-size classes and aggregate-associated carbon, Soil Sci. Soc. Am. J., 64, 681–689, 2000.
Six, J., Bossuyt, H., Degryze, S., and Denef, K.: A history of research on the link between (micro)aggregates, soil biota, and soil organic matter dynamics, Soil Till. Res., 79, 7–31, 2004.
Sleutel, S., De Neve, S., Singier, B., and Hofman, G.: Organic C levels in intensively managed arable soils – long-term regional trends and characterization of fractions, Soil Use Manag., 22, 188–196, https://doi.org/10.1111/j.1475-2743.2006.00019.x, 2006.
Smith, P.: Do grasslands act as a perpetual sink for carbon?, Glob. Change Biol., 20, 2708–2711, https://doi.org/10.1111/gcb.12561, 2014.
Smith, P.: Soil carbon sequestration and biochar as negative emission technologies, Glob. Change Biol., 22, 1315–1324, https://doi.org/10.1111/gcb.13178, 2016.
Solomon, D., Lehman, J., Kinyangi, J., Amelung, W., Lobe, I., Pell, A., Riha, S., Ngoze, S., Verchot, L., Mbugua, D., Skjemstad, J., and Schafer, T.: Long-term impacts of anthropogenic perturbations on dynamics and speciation of organic carbon in tropical forest and subtropical grassland ecosystems, Glob. Change Biol., 13, 511–530, https://doi.org/10.1111/j.1365-2486.2006.01304.x, 2007.
Tisdall, J. M. and Oades, J. M.: Organic matter and water-stable aggregates in soils, J. Soil Sci., 33, 141–163, 1982.
van Bavel, C. H. M.: Mean Weight-Diameter of Soil Aggregates as a Statistical Index of Aggregation1, Soil Sci. Soc. Am. J., 14, 20–23, https://doi.org/10.2136/sssaj1950.036159950014000c0005x, 1950.
von Haden, A. C., Kucharik, C. J., Jackson, R. D., and Marín-Spiotta, E.: Litter quantity, litter chemistry, and soil texture control changes in soil organic carbon fractions under bioenergy cropping systems of the North Central U.S., Biogeochemistry, 143, 313–326, https://doi.org/10.1007/s10533-019-00564-7, 2019.
Wiesmeier, M., Urbanski, L., Hobley, E., Lang, B., von Lützow, M., Marin-Spiotta, E., van Wesemael, B., Rabot, E., Ließ, M., Garcia-Franco, N., Wollschläger, U., Vogel, H. J., and Kögel-Knabner, I.: Soil organic carbon storage as a key function of soils – A review of drivers and indicators at various scales, Geoderma, 333, 149–162, https://doi.org/10.1016/j.geoderma.2018.07.026, 2019.
Yamashita, T., Flessa, H., John, B., Helfrich, M., and Ludwig, B.: Organic matter in density fractions of water-stable aggregates in silty soils: Effect of land use, Soil Biol. Biochem., 38, 3222–3234, https://doi.org/10.1016/j.soilbio.2006.04.013, 2006.