the Creative Commons Attribution 4.0 License.
the Creative Commons Attribution 4.0 License.
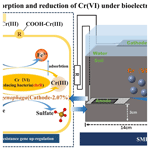
Cr(VI) reduction, electricity production, and microbial resistance variation in paddy soil under microbial fuel cell operation
Huan Niu
Xia Luo
Peihan Li
Hang Qiu
Liyue Jiang
Subati Maimaitiaili
Minghui Wu
Fei Xu
Heng Xu
The microbial fuel cell (MFC) is an efficient in situ approach to combat pollutants and generate electricity. This study constructed a soil MFC (SMFC) to reduce Cr(VI) in paddy soil and to investigate its influence on microbial community and microbial resistance characteristics. Ferroferric oxide (Fe3O4) nanoparticles, as the cathodic catalyst, effectively boosted power generation (0.97 V, 102.00 mW m−2), with the porous structure and reducibility also contributing to chromium (Cr) reduction and immobilization. After 30 d, 93.67 % of Cr(VI) was eliminated. The bioavailable Cr decreased by 97.44 %, while the residual form increased by 88.89 %. SMFC operations greatly changed soil enzymatic activity and microbial structure, with exoelectrogens like Desulfotomaculum (3.32 % in the anode) and Cr(VI)-reducing bacteria like Hydrogenophaga (2.07 % in the cathode) in more than 1000 folds of soil. In particular, SMFC operations significantly enhanced heavy-metal resistance gene (HRG) abundance. Among them, chrA, chrB, and chrR increased by 99.54 %–3314.34 % in SMFC anodes, probably attributable to the enrichment of potential tolerators like Acinetobacter, Limnohabitans, and Desulfotomaculum. These key taxa were positively correlated with HRGs but were negatively correlated with pH, electrical conductivity (EC), and Cr(VI), which could have driven Cr(VI) reduction. This study provided novel evidence for bio-electrochemical system applications in contaminated paddy soil, which could be a potential approach for environmental remediation and detoxification.
- Article
(8107 KB) - Full-text XML
-
Supplement
(2109 KB) - BibTeX
- EndNote
Chromium (Cr) is a main toxic heavy metal (HM) that enters the environment mainly due to its wide use in electroplating, tanning, and other industries (Coetzee et al., 2020). Mineral-sourced phosphate fertilizer also contains high-level Cr, promoting its spread, migration, and accumulation in soil and underground water (Chen et al., 2022a). The persistence and toxicity of HMs create permanent and selective stress on environmental microbes. Under the toxic stress, microorganisms have deployed various strategies, including active efflux of the toxins from the microbial cell, regulation of targets, and enzymatic modification of the toxic, presenting as an elevation of the heavy-metal resistance gene (HRG) and tolerant microbes (Van Hoek et al., 2011). Even a sub-dose of Cr (especially the Cr(VI) state) can promote plasmid-mediated horizontal gene transfer (HGT) (Zhang et al., 2018), causing HRG enrichment, threatening environmental safety (Guo et al., 2021; Wang et al., 2020a, 2023b). Meanwhile, due to the co-selection effect, the long-term existence of HMs also causes the enrichment of antibiotic-resistant bacteria (ARB), further increasing the resistance-gene-spreading risk in the environment (Men et al., 2018). Such a threat could be more fierce under the coexistence of HMs and antibiotics (Ashbolt Nicholas et al., 2013). Hence, the enrichment of HRGs and ARB under Cr exposure has become an emerging concern.
Common remediation methods for Cr-influenced soil include chemical reduction and leaching (Cong et al., 2022), electrokinetic remediation (Morales-Benítez et al., 2023), and phytoaccumulation (Yaashikaa et al., 2022), which convert Cr(VI) into insoluble and low-toxicity forms (e.g., Cr(III)) by adsorption, ion exchange, and redox (Rani et al., 2022). Among them, the microbial approach using functional microbes is commonly used for the continuous treatment of soil groundwater, which has a low cost without side effects (Fan et al., 2023). For example, Comamonas testosteroni bacterial strains are used to degrade hexachlorobenzene, and Acinetobacter calcoaceticus GSN3 strain is used to degrade phenol (Dimova et al., 2022; Irankhah et al., 2019). However, pollutants can be tightly adsorbed by soil particles and persistently remain (Wang et al., 2023b). The complex soil constituents and the competition of indigenous microorganisms inhibit the colonization and development of functional microbes and limit their effectiveness (Guo et al., 2021).
Microbial fuel cell (MFC) technology can transform or immobilize HMs and generate electricity utilizing electrochemical active bacteria (EAB) (Gupta et al., 2023; Chen et al., 2022b), which have been used in sediment or soil to treat HMs and organics and to monitor environmental toxicity (Li et al., 2023b). For example, soil microbial fuel cells can mitigate the accumulation of heavy metals in rice and promote the removal of atrazine in the soil (Gustave et al., 2020; Farkas et al., 2024). At present, soil MFC (SMFC) has been used for pollution control, focusing on pollutant content and forms, as well as on the electrochemical properties (Hamdan and Salam, 2023; Liu et al., 2023a). There is a lack of systematic research about the MFC effect on soil microbial community structure shifting and resistance characteristics, especially under the HM contamination circumstance.
In this study, an SMFC was constructed to remediate Cr(VI)-contaminated paddy soil. EAB were pre-loaded on the SMFC anode to promote electricity production and Cr transformation. Ferroferric oxide (Fe3O4) nanoparticles, which can reduce and fix Cr(VI) directly, were used as a catalyst for cathodic oxygen reduction reaction (ORR) to improve SMFC performance due to their catalytic effect, non-toxicity, and cost-effectiveness, as well as the fact that they are free of secondary pollution (Liu et al., 2023b). During operation, Cr(VI) was simultaneously reduced and immobilized by bio-physical adsorption and electrochemical–microbial reduction. The Cr(VI) reduction mechanism and the microbial community structure shaping and HRG variation were comprehensively studied. For the first time, SMFC-driven Cr(VI) reduction was associated with microbial resistance, which evolved along with microbial adaptation and development. This study not only provides a reference for the microbial remediation of polluted soil but also improves the practical field application of MFC. The method can be used for in situ contaminant treatment in various environments, such as aquaculture ponds, inland lakes, and wetlands.
2.1 Chemicals
All the chemicals and reagents were analytical grade or premium pure from the Kelong Chemical reagent factory, Chengdu, China.
2.2 Construction of the SMFC
2.2.1 Soil
Paddy soil from Jintang County, Chengdu, China (30°74′ N, 104°59′ W) was collected and used to construct the SMFC. The soil has organic matter, organic carbon, and total nitrogen of 8.84 %±0.02 %, 1.74 %±0.01 %, and , respectively. Referring to previous studies and the Chinese Soil Environmental Quality Standard (GB15618-2018), 120.00 mg kg−1 potassium dichromate was added to the soil, and a final Cr(VI) concentration of 118.80 mg kg−1 was achieved before use (Liu et al., 2020; Mandal et al., 2017; Li et al., 2024).
2.2.2 Electrode preparation
Aluminum foam (, porosity of 60.00 %–80.00 %, bulk density of 0.50–1.10 g cm−3) (Sanzheng Metal material, Chengdu, China) was used as the anode. The anode microflora was derived from municipal sludge (Chengdu Sixth Sewage Treatment Plant, China) after acclimating with 100.00 mg L−1 Cr(VI). Before assembling, the aluminum foam was cultivated in the anode microflora for 2 weeks. Then the anode was tied tightly to titanium mesh with titanium wire. Graphite felt (GF) (, bulk density of 0.10–0.15 g cm−3) was used as the cathode (Table S1 in the Supplement). Before use, it was cleaned, dried, and loaded with Fe3O4 as the ORR catalyst, as detailed in Sect. S1 in the Supplement. For characterization, we utilized a scanning electron microscope (SEM) to examine the structure and morphology of the electrode surface. In addition, we performed X-ray photoelectron spectroscopy (XPS) and energy dispersive spectroscopy (EDS) to analyze the valence state and element composition. The phase composition was determined using an X-ray diffractometer (XRD).
2.2.3 SMFC construction
A plastic box () was used as the SMFC reactor, with 1.50 kg air-dried soil and overlying water of 3.00 cm to simulate the flooded state during rice planting. The cathode was floated on the water surface, while the anode was buried (about 3.00 cm from the bottom). The cathode and anode were connected to a 2000 Ω resistor using titanium wire. The water level was kept constant by means of daily replenishment (Fig. S1 in the Supplement).
2.3 Design and operation
Three treatments were set up as shown in Fig. S1, and three parallels were set for each group. For the NMFC (non-electrode microbial fuel cell), the control group with no electrode only contains an equal amount of overlying water and paddy soil. For the OMFC (open-circuit microbial fuel cell), an open-circuit group was used with disconnected electrodes and equal amounts of overlying water and paddy soil. For the CMFC (closed-circuit microbial fuel cell), a complete closed-circuit SMFC capable of producing electricity was used, with electrodes connected by a 2000 Ω resistor and equal amounts of overlying water and paddy soil.
The experiments were conducted at 25 °C. A Raspberry Pi data acquisition system (ARMv7 architecture) was connected at both ends of the resistor of the CMFC to monitor the voltage. A multimeter was used for verification. The detailed code information can be found in Sect. S1.
Soil and water samples were taken every 5 d until day 35, and the operation continued for another 10 d until day 45 (specific sampling details are given in the Supplement). The electrochemical properties of the SMFC, including the polarization curve and power density curve, were tested using an electrochemical workstation on days 15 and 30 (Ch660e, Shanghai Chenhua Instrument Co., Ltd., Shanghai, China) (Chen et al., 2022b).
2.4 Cr migration and transformation
To determine total Cr, 0.50 g soil was subjected to acid digestion (HCl–HNO3–HClO4) before measurement using flame atomic absorption spectrometry (FAAS) (PinAAcle 900T AA Spectrometer, PerkinElmer, America). To determine Cr speciation, European Community Bureau of Reference (BCR) sequential extraction was applied to divide Cr into HOAc extractable, reducible, oxidizable, and residual fractions, with mobility and availability from high to low (Wang et al., 2020a). Also, the Cr(VI) concentration in overlying water was determined by a spectrophotometer at 540 nm, while the Cr(VI) in soil was determined using FAAS after alkaline digestion (Fan et al., 2021). Duplicates, method blanks, and standard reference materials were used for quality control. Cr recovery in standard reference materials was 92.00 %–108.00 %.
2.5 Microbial response during operation
2.5.1 Soil biochemical response
Soil dehydrogenase (DHA) activity was measured using the 2,3,5-triphenyl tetrazolium chloride (TTC) method. Urease activity was determined by the phenol sodium hypochlorite colorimetric assay. Invertase activity was determined by the 3,5-dinitro salicylic acid colorimetric assay. The acid phosphatase (ACP) activity was determined by the p-nitrophenyl disodium phosphate colorimetric assay (Wang et al., 2019, 2017).
2.5.2 Microbial community structure
The microbial community structure of the electrodes and soil was determined by high-throughput sequencing (Faust and Raes, 2012; Deng et al., 2012; Bokulich et al., 2018). Majorbio (Shanghai, China) performed 16S rRNA gene sequencing using the Illumina HiSeq platform; 0.50 g of fresh homogenized samples was used to extract the total bacterial DNA with a universal DNA Kit (Omega Bio-tek Inc., USA). After amplification and purification, the V3–V4 hypervariable regions of the bacterial 16S rRNA gene were amplified with the primer pairs 338F and 806R. After sequencing, the operational taxonomic units (OTUs) with a 97.00 % similarity cut-off were clustered using UPARSE version 7.1, and chimeric sequences were identified and removed. The taxonomy of each OTU representative sequence was analyzed by means of the RDP Classifier version 2.2 against the 16S rRNA database using a confidence threshold of 0.7. The alpha diversity, beta diversity, microbial community structure change, and environmental factor correlation analyses were conducted (Wang et al., 2023a).
2.5.3 HRG fluctuation
A microbial DNA rapid extraction kit (Sangon biotech Co., Ltd., Shanghai, China) was used to extract total DNA from fresh samples (the extraction method is shown in the Supplement). The abundance of HRGs in the surface soil of the SMFC and OMFC anodes after operation was analyzed using an SYBR Green real-time fluorescence quantitative PCR system (7500, Thermo Fisher Scientific, USA) (Wang et al., 2023c). The soil of the OMFC was used for comparison. The detected genes included HRGs (chrA, chrB, chrR, recG, nfsA, zupT, fpvA) and mobile genetic elements or MGEs (intI, tnpA02, tnpA04, tnpA05). The primer sequences are provided in Table S2. The specific detection steps were as follows: pre-denaturation at 95 °C for 30 s, denaturation at 95 °C for 5 s, and annealing and extension at 60 °C for 30 s. A total of 40 cycles were performed to make three replicates, and 16S rRNA was used as the internal reference gene. The relative gene expression results were analyzed using the 2(−ΔΔCt) method, which is commonly used for relative quantification, where ΔΔCt= (Ct target gene − Ct internal reference gene) experimental group − (Ct target gene − Ct internal reference gene) control group.
2.6 Data analysis
The experimental data were evaluated using one-way analysis of variance (ANOVA) based on three tests. The mean values and standard deviations were calculated using SPSS 22.0 software (IBM, USA). A significance level of P<0.05 was considered to be statistically significant, while P<0.01 was defined to be highly statistically significant. Graphs were plotted using Origin 2022 software.
3.1 Electrode characterization
As demonstrated in Figs. 1, 2, and S2, the raw GF had smooth surfaces with C and O as the main elements (Fig. S2). After Fe3O4 loading, black patches constituted with spherical particles appeared, bringing Fe (13.17 %) and O (19.97 %) to the GF surface (Fig. 1a–c and Table S3). XPS revealed that peaks of the cathode GF loaded with the catalyst at 710.80 and 724.40 eV were consistent with typical Fe3O4 peaks (Fig. 2a and b), indicating its successful loading. The CV curves of the cathode (Fig. 2c) presented an obvious oxidation peak at 0.85 V, indicating its excellent electrochemical performance.
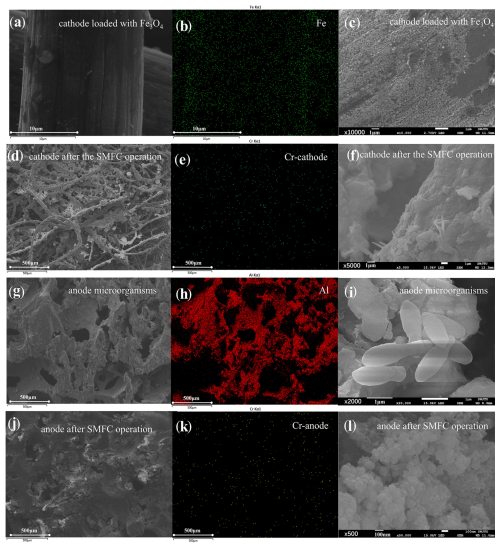
Figure 1Characterization of electrode materials before and after operation by SEM and EDS. (a–c) SEM and EDS images of cathode loaded with Fe3O4; (d–f) SEM and EDS images of cathode after the SMFC operation; (g–i) SEM and EDS images of anode microorganisms; (j–l) SEM and EDS images of the anode after SMFC operation. Note that the first column is the SEM image, the second column is the corresponding EDS, and the third column is the corresponding enlarged SEM image.
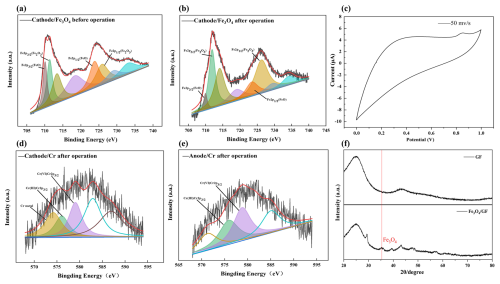
Figure 2Characterization of electrode materials. (a, b) Fe2p spectra of cathode–Fe3O4 composite cathode, (c) cyclic voltammetry (CV) curve of cathode–Fe3O4, (d, e) Cr2p spectra of GF composite cathode and anodic aluminum foam after operation, (f) XRD spectrum of the cathode–Fe3O4.
Chromium mainly exists in the III and VI oxidation states in soil. The peaks at 576.90 and 579.00 eV are typical peaks of Cr(III) and Cr(VI), respectively. After operation, the typical peaks of Cr(III) and Cr(VI) can be observed on the X-ray energy spectra of both the cathode and anode, and Cr(VI) and Cr(III) are present on the cathode and anode of the SMFC (Fig. 2d and e). The results showed that at least the valence state of Cr changed on the electrode, and Cr(VI) was reduced and fixed by the electrode (Kim et al., 2017). GF was found to be loaded with many soil elements, including Cr, Na, Mg, and Ca (Table S3). SEM also observed many microorganism cells and extracellular organic-like substances, implying the biofilm formation on the cathode (Fig. 1d–f).
As presented in Fig. S2c, the raw aluminum foam showed a rough porous structure, with mainly Al and O on the surface (Table S3). After loading EAB, many spherical and rod-shaped bacteria were observed, indicating that aluminum foam has a good capacity to carry microorganisms (Fig. 1g–i). After the operation, many millimeter-scale soil particles were embedded in the anode interspace, indicating the intense mass transfer between the anode and soil (Fig. 1j–l).
3.2 Electricity generation performance
Initially, the CMFC showed a working-circuit voltage (WCV) of 0.55 V and an open-circuit voltage (OCV) of 0.68 V (Fig. 3a). In the first week, WCV dropped quickly to 0.45 V but bounced back and stabilized at 0.75 V on day 25, implying the adaption process of the anode microbes in the soil. We reasonably concluded that, in the complex heterogeneous environment of soil, the anodic EAB needs some time to adapt to fluctuating environmental conditions facing environmental disturbances. During the SMFC operation, the anode microbial community could be gradually selected and enriched, and a stable adaptive community is formed so that the SMFC voltage tends to be stable. This conjecture is also reflected in subsequent results.
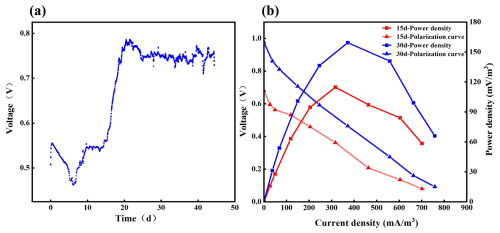
Figure 3Power generation performance of the SMFC. (a) SMFC output voltage distribution; (b) 15 d vs. 30 d polarization curves and power density curves of the SMFC.
On day 15 (OCV of 0.67 V) and day 30 (OCV of 0.97 V), a series of resistors (50–10 000 Ω) was connected to the electrodes to determine the polarization curves and power density of the SMFC. As shown in Fig. 3b, the power density increased and decreased with the elevation of external resistance. At 510 Ω, the power density reached a maximum of 114.90 mW m−3 (73.50 mW m−2) on day 15 and a maximum of 159.40 mW m−3 (102.00 mW m−2) on day 30 (Table S4). The result indicated that the electrochemical performance of the SMFC was enhanced gradually, probably due to the microbial adaption. Even after 45 d, the CMFC still had a WCV of around 0.75 V, indicating its substantial and stable electricity-producing capacity. Compared with the literature, the SMFC in the current work has an outstanding power generation capacity (Table S5).
3.3 Cr(VI) reduction and immobilization during operation
The forms and speciations of HMs determine their bioavailability and toxicity (Jia et al., 2022). After operation, Cr forms in soils were significantly changed (P<0.05) (Fig. 4). In CMFC, the acid-soluble Cr decreased substantially by 97.44 %, the oxidizable and reducible fractions did not change significantly, while the residual form of Cr increased by 88.89 % (Fig. 4a). However, in the NMFC, the acid-soluble Cr increased substantially by 61.54 % on day 35. In the OMFC, the acid-soluble Cr increased before decreasing, which was opposite to its oxidizable state. On day 35, the Cr bioavailability of the OMFC (11.90 %) and the NMFC (18.90 %) was 3866–6200-fold that of the CMFC (0.30 %). It is inferred that the electric field and the microbial communities' evolvement may lead to better Cr immobilization and may transform Cr into less toxic forms.
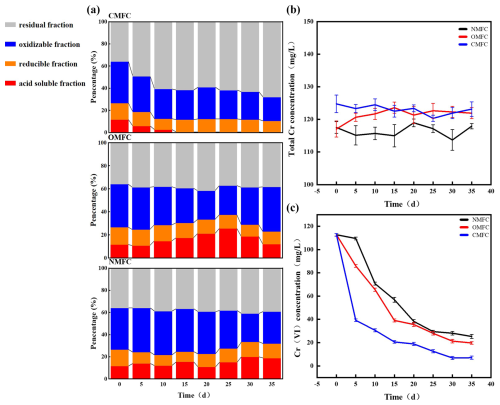
Figure 4Cr speciation and transformation during SMFC operation. (a) Percentage share of Cr in different chemical fractions in the CMFC, OMFC, and NMFC soils. Changes in soil (b) total chromium and (c) Cr(VI) concentrations during SMFC operation.
In the meantime, the Cr(VI) concentration (Fig. 4c) dropped in all the groups, and Cr(VI) in the CMFC soil was significantly lower than in the OMFC and NMFC soil (P<0.05) after the experiment. On day 35, the CMFC showed 13.59 % and 20.87 % higher Cr(VI) elimination than the OMFC and NMFC, respectively. The overlying water was initially free of Cr. During the experiment (Fig. S3), 0.21–12.72 mg L−1 Cr was determined, which could be released from the soil. A low level of Cr(VI) (less than 3.15 mg L−1) was detected but vanished later (day 15), which could be attributed to the dynamic adsorption–desorption of soil particles and electrodes.
3.4 Soil properties
3.4.1 Soil physicochemical property
The soil pH decreased in all the groups (Fig. S4a). The soil pH of the CMFC decreased fastest from the initial 7.71 to about 6.83 on day 35, with a minimum of 6.77 on day 30, which was 0.14 %–7.87 % lower than the others (P<0.05). During the experiment, oxygen in the flooded soil decreased rapidly due to microbial consumption, and acidic products (e.g., low-molecule organic acids) were produced to increase soil acidity. Microorganisms (especially EAB) decompose soil organic matter and release a large number of electrons and protons, making oxidizing substances such as nitrate and high-valence metals (Fe(III), Mn(IV), and Cr(VI)) to accept electrons for reduction, causing proton (H+) accumulation (He et al., 2016). Such a phenomenon was more intense in the CMFC due to the rapid electron transfer through wire to the cathode, leaving protons elevated near the anode.
In all of the groups, electrical conductivity (EC) increased rapidly from the initial 1.55 ms cm−1 before stabilizing (Fig. S4b), which was maximized by 2.60, 2.40, and 2.40 ms cm−1 in the CMFC, OMFC, and NMFC (P>0.05), respectively. The rapid increase in EC could be attributed to the inundation that increased the soluble salt content of the soils. The electromigration in the MFC electric field may also increase soil mass transfer and positively affect soil electrical conductivity (Zhang et al., 2020).
3.4.2 Soil biochemical response
Soil enzyme activity is an important index to evaluate soil environmental change. Soil enzymes, being biocatalysts involved in biochemical reactions, play an important role in nutrient mineralization, decomposition of organic matter, and nutrient cycling (Chen et al., 2024; Liu et al., 2018). The DHA activity increased significantly in all three groups (2244.00 %–3138.00 % higher than the initial value) and did so continuously (P<0.05) (Fig. S5a). Under flooding, microbial activity changed from aerobic to anaerobic, leading to a sharp decline in soil redox potential, accompanied by the stimulation of soil DHA (Sardans and Peñuelas, 2005). During operation, urease activity in the CMFC showed a gradual increase (2.70 %–12.40 % higher than day 0 on days 10–35), while, in the OMFC and NMFC, it showed a slight decrease (6.10 %–7.10 % lower than day 0 on days 5–35) (Fig. S5b). The SMFC electric field and Fe(II) promote extracellular electron transfer (EET) (Chen et al., 2023a), which promotes the enrichment of ammonia–nitrogen-transforming bacteria in soil, which could have caused the higher urease activity in the CMFC than in the NMFC and OMFC (P<0.05). Soil invertase activity decreased initially but increased later for the CMFC and OMFC but showed an opposite trend for the NMFC. After operation, the CMFC had a significantly higher invertase activity than others (P<0.05) (Fig. S5c). Soil ACP showed a similar trend with urease, with the CMFC continuously increasing by 13.20 %–48.90 % and being considerably higher than the OMFC and NMFC (Fig. S5d). The dynamic measurement of soil enzyme activity in the SMFC helps us to understand and analyze the changing state of soil micro-ecology.
3.5 SMFC operations reshaped soil microbial communities
Microbial community structures in the electrodes were analyzed, with the obtainment of 15 dominant phyla and 50 dominant genera (>1.00 %). Overall, Firmicutes (73.93 %), Proteobacteria (62.53 %), and Chloroflexi (21.73 %) were found to be the dominant phyla, while Bacteroides (21.48 %), Enterococcus (17.26 %), and Hyphomicrobium (15.34 %) were the dominant genera.
The alpha diversity analysis indicated a significant difference in the microbial community among the samples (Fig. 5). The higher chao1 index in soils than in the electrodes demonstrated higher microbial richness. Most of the alpha indices in the OMFC-A and OMFC-C (see definitions in Appendix A) were significantly higher than in the CMFC-A and CMFC-C (see definitions in Appendix A), demonstrating a higher microbial richness and diversity in the OMFC. The results indicated the different microbial evolution patterns in different electrodes and the selection effect of the electricity field during CMFC operation. The Venn diagram (Fig. S6) found no OTU coincidence among the samples, indicating their obvious specificity. In comparison, 2130 OTUs were shared by the CMFC-S, OMFC-S, and NMFC-S (see definitions in Appendix A), indicating the similarity of the soil microbial community (Fig. S6b). A total of 45 OTUs were shared by the Raw-A, CMFC-A, and OMFC-A, accounting for 45.92 %, 1.95 %, and 1.16 %, respectively, and indicating the successful colonization and development of the preloaded EAB.
3.5.1 Soil microbial community reshaping on phylum level
At the phylum level (Fig. 6a), before the operation, the anode was dominated by Firmicutes (73.93 %), Bacteroidota (22.74 %), and Proteobacteria (1.27 %). After the operation, Firmicutes and Bacteroidota decreased by 49.83 % and 66.84 % in the CMFC-A and by 73.20 % and 26.52 % in the OMFC-A, respectively, while Proteobacteria increased by 1698.43 % in the CMFC-A and by 475.59 % in the OMFC-A. Besides this, many other phyla emerged, including Acidobacteriota (9.41 %–21.40 %), Actinobacteriota (0.23 %–9.52 %), Halanaerobiaeota (1.48 %–4.77 %), Myxococcota (1.51 %–3.93 %), and Chloroflexi (0.01 %–2.57 %), indicating the increased microbial diversity, probably due to the penetration of soil indigenous microbes.
The cathode was free of microorganisms initially. However, many phyla were observed after the operation. The CMFC-C was dominated by Proteobacteria (62.53 %), Actinobacteriota (6.24 %), Planctomycetota (6.04 %), Chloroflexi (5.88 %), and Bacteroidota (3.81 %), while the OMFC-C was dominated by Proteobacteria (47.93 %), Chloroflexi (9.75 %), Cyanobacteria (8.30 %), Acidobacteriota (8.01 %), Actinobacteriota (3.97 %), Myxococcota (3.92 %), and Gemmatimonadota (2.87 %). The Proteobacteria phylum was rich in EAB, and its advantage in both electrodes of the CMFC indicated that the SMFC operation was favorable for EAB colonization and development. All the soils were dominated by Chlorobacteria, Acidobacteria, Proteobacteria, Bacteroidetes, and Myxococcota, and the difference was not significant.
3.5.2 Soil microbial community reshaping on genus level
At the genus level (Fig. 6b), MFC operation presented a selection effect, with Terrisporobacter increasing from 0.81 % to 13.71 % and Bacteroides decreasing from 12.48 % to 0.53 % in the CMFC anode. Compared with the Raw-A, many EAB in the CMFC-A decreased, including Clostridium_sensu_stricto_5 (from 12.99 % to 0.05 %), Clostridium_sensu_stricto_15 (from 4.70 % to 0.47 %), and Enterococcus (from 17.26 % to 0.03 %) (Choi, 2022; Zhang et al., 2023).
However, Desulfotomaculum in the CMFC-A increased to 3.32 % compared with 0.003 % in the soil (CMFC-S). Besides this, soil indigenous bacteria, including Ramlibacter, Methyloversatilis, and Acinetobacter, were colonized in the anode and elevated 4.89–1579.00-fold compared with soil. Nevertheless, multiple dominant genera in the soils decreased more in the CMFC-A than in the OMFC-A. For example, SBR1031, Bacteroidetes_vadinHA17, and Anaerolinea increased significantly in soil but less so in the CMFC-A and OMFC-A. The electric field action, to a certain extent, helped the anode to resist external microbial intrusion to ensure the stability of the anodic microbial community.
During operation, the prolonged interaction between the soil and water phases resulted in the gradually evolving unique biofilm structure of the cathode. For instance, Hyphomicrobium (3.56 %–15.34 % in soils), an aerobic chemoheterotroph capable of degrading a wide range of organics, accounted for 15.34 % and 3.56 % of the CMFC-C and OMFC-C, respectively (He et al., 2019). Hydrogenophaga, a gram-negative bacteria capable of denitrification and Cr(VI) reduction, accounted for 2.07 % of the CMFC-C (Wang et al., 2022). Meanwhile, the SMFC operation caused the enrichment of several resistant bacteria. Subgroup_7, a typical HM-tolerant bacterium (Li et al., 2023a), was enriched in both cathode and soil. Acinetobacter and Limnohabitans, also tolerators that carry HRGs and ARGs, were found to be 4.31 % and 3.03 % in the CMFC-A (Dahal et al., 2023; Zhang et al., 2021).
The increase in iron in soil and water due to the use of Fe3O4 as a cathode catalyst may be responsible for the enrichment of Terrisporobacter and Anaeromyxobacter in the CMFC-A and OMFC-A. They were found to be closely associated with the Fe3+ reduction in gain energy in various environments (Lin et al., 2007; Wang et al., 2020b).
3.5.3 Soil metal resistance gene variation
Under Cr(VI) stress, certain microbes would utilize pathways like specific or non-specific Cr(VI) reduction, free radical detoxification, and DNA damage repair to survive in toxic environments (Morais et al., 2011). Using qPCR analysis, the abundance of typical HRGs and MGEs in the anodic soils was determined (Fig. 7a), among which chrA, chrB, and chrR, are typical HRGs involved in the Cr response, while intI, tnpA02, and tnpA05 are common mobile genetic elements (MGEs) that varied greatly during operation (P<0.05). Compared with the OMFC and NMFC, chrA in the CMFC increased by 237.83 % and 3414.34 %, chrB increased by 141.52 % and 153.63 %, chrR increased by 221.86 % and 839.41 %, intI increased by 151.77 % and 167.91 %, tnpA02 increased by 331.86 % and 1118.97 %, and tnpA05 increased by 416.91 % and 99.54 %. Changes in soil resistance characteristics during SMFC operation may be directly or indirectly caused by environmental factors and resistant microbial communities.
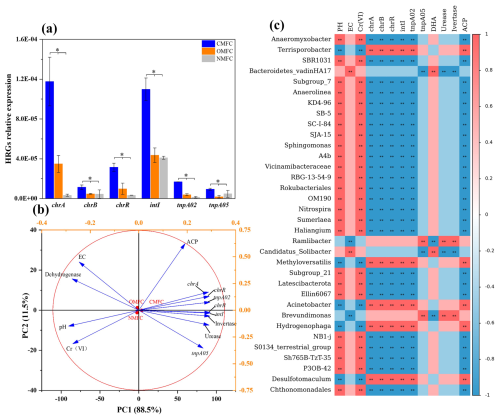
Figure 7HRG variation and correlation analysis of dominant bacteria, HRG, and soil enzymatic activity. (a) qPCR results of HRG changes in soil around anode soil after SMFC operation (chrA, chrB, chrR, intI, tnpA02, tnpA05). Different letters denote significant differences among treatments (P<0.05). (b) Principal component analysis (PCA) of the SMFC soil physicochemical properties with enzyme activity, HRGs, and native bacterial genera. (c) Spearman's correlation heatmap (mean relative abundance>1 %). according to least-square difference (LSD) test (mean ± SE, n=3); .
The elevation of HRGs and MGEs could be due to the enrichment of multiple metal-resistant bacteria (MRB) such as Acinetobacter, Limnohabitans, and Brevundimonas. Moreover, the anodic Desulfotomaculum, which accounted for 3.23 % of CMFC-A, is a typical sulfate-reducing bacterium (SRB) that produces H2S, a natural signaling molecule that contributes to tolerance triggering, maintenance, and diffusion through community sensing, which facilitates HRG elevation through HGT (Shatalin et al., 2021). Besides, Cr(VI)-reducing bacteria like Hydrogenophaga (1.31 % in the CMFC-A) may also up-regulate the Cr reductase gene chrR (Sundarraj et al., 2023).
Furthermore, pH changes may also affect soil resistance characteristics. Liu et al. (2023c) observed that the abundance of multi-drug efflux pump genes in the acid soil was significantly positively correlated with soil acidity. The intensified proton generation and accumulation in the CMFC could have led to the HRG elevation. In addition, HM toxicity exerts direct selective pressure, which affects microbial community structures and their functioning, leading to the thriving of tolerators like Desulfotomaculum sp., Hydrogenophaga, and Methylophilus (Hernández-Ramírez et al., 2018) and, hence, the spontaneous HRG elevation (Wang et al., 2023c).
3.6 Correlation analysis
To visually analyze the correlation between bacterial communities and environmental factors, Spearman correlation analysis and principal component analysis (PCA) were conducted (Fig. 7). Spearman correlation analysis (Fig. 7c) isolated four main bacterial genera clusters, which further revealed the correlation of environmental factors (pH, EC), soil enzyme activities (DHA, urease, invertase, ACP), Cr(VI), and HRGs (chrA, chrB, intI, tnpA02, tnpA05) with the microbial community.
Cluster 1 (Terrisporobacter, Methyloversatilis, Acinetobacter, Hydrogenophaga, and Desulfotomaculum) was positively correlated with HRGs (chrA, chrB, intI, tnpA02) and ACP (P<0.01) but negatively correlated with pH and Cr(VI) (P<0.01), suggesting that cluster 1 may contribute to HGT and HRG enrichment. Cluster 2 (Anaeromyxobacter, Subgroup_7, Anaerolinea, SB-5, Sphingomonas, etc.) was negatively correlated (P<0.01) with HRGs (chrA, chrB, intI, tnpA02) and ACP but positively correlated with pH and Cr(VI) (P<0.01). Cluster 3 (Bacteroidetes_vadinHA17 and Candidatus_Solibacter) was positively correlated (P<0.01) with soil EC and DHA. Cluster 4 (Ramlibacter and Brevundimonas) was positively correlated with urease and invertase but negatively correlated with EC.
PCA analysis (Fig. 7b) also indicated a close correlation between environmental factors, in which soil pH, EC, Cr(VI) concentration, and DHA were positively correlated with each other but negatively correlated with urease, ACP, invertase, and HRGs. In particular, Cr(VI) was significantly negatively correlated with HRGs (P<0.01), which could be partially explained by the thriving of the tolerators and the Cr(VI) reduction during SMFC operation.
In this study, Cr(VI) reduction, microbial community variation, and HRG fate in the SMFC were investigated for the first time. The results proved that the SMFC was an effective method to eliminate Cr(VI) (93.76 %), immobilize Cr (97.44 %), and generate electricity (0.97 V).
Microorganisms have developed efficient detoxification strategies to counteract the toxic effects of heavy-metal stress (Rouch et al., 1995; Tan et al., 2020). In the SMFC system, Cr(VI) reduction was a synergic result of adsorption and/or biosorption (including the adsorption of anode and cathode materials, surface catalyst adsorption, and microbial membrane adsorption), bio-electrochemistry reduction, and microbial reduction (including intracellular sequestration, export, reduced permeability, extracellular sequestration, and extracellular detoxification) (Fig. 8). The pre-loading of Fe3O4 and EAB on the electrodes significantly improved Cr(VI) reduction and power generation by accelerating SMFC stabilization. The detailed explanation is as follows.
The electricity-producing process of the SMFC can inhibit HMs' release and migration in soil (Zhu et al., 2019; Feng et al., 2024). In this study, Cr forms changed greatly from acid-soluble to a more stable residual fraction with low toxicity. The Fe3O4-modified cathode not only directly adsorbs or reduces Cr(VI) due to the high specific area and ferrous iron but also enhances the electrochemical effect of the system. The electrons derived from the anodic microbial metabolism can directly reduce Cr(VI) in the soil to Cr(III), while part of them is transmitted to the cathode, where Cr(VI) in the overlying water competes with oxygen as electron acceptors and complete the current loop (Thapa et al., 2022).
In addition, microorganisms can also directly or indirectly reduce or fix Cr. Biosorption, sulfide, and hydroxide precipitation are the main immobilization mechanisms of HMs by microorganisms (Ma et al., 2024). For example, Desulfotomaculum sp., a typical SRB, enriched to 3.23 % in the CMFC-A, may produce sulfide ions by reducing alienated sulfate, thus forming highly insoluble metal sulfides to fix Cr through microorganism-induced sulfide precipitation (MISP). Hydrogenophaga, which dominated in both electrodes, was a known Cr-reducing bacteria. Some of the iron-reducing bacteria present in this study (e.g., Anaeromyxobacter and Terrisporobacter) may also contribute to Cr(VI) reduction by participating in the Fe cycle through EET, while the ferrous iron reduces Cr(VI). Additionally, the CMFC in this work contains many genera capable of transforming nitrogen. For example, Hyphomicrobium dominated in the CMFC-C (15.34 %); this is a typical denitrifying bacterium and can effectively reduce nitrate and nitrite (Ernst et al., 2021). Methylophilus, which is a methylotrophic microorganism, accounted for 2.93 % of the CMFC-A but was much lower in other groups (Yang et al., 2020). The bacteria mentioned above were found to have a high urease-producing ability, and their enrichment not only improves soil urease activity and nutrient cycling but also immobilizes Cr through microorganism-induced carbonate precipitation (MICP) (Qian et al., 2017).
In addition to the reduction of Cr during SMFC operation, under electrochemical selection and HM stress, the microbial community gradually evolved, with higher richness and diversity, along with the HRG enrichment and nutrient-cycling variation. Firstly, some soil indigenous bacteria were much lower in the CMFC-A than in the OMFC-A, indicating that the electric field contributed to the anode stability by preventing external bacteria intrusion and that it is less vulnerable to environmental fluctuations. The microbial community change is significantly related to HRG enrichment. Many EAB and MRB are significantly enriched, for example, Desulfotomaculum, an SRB with a dual role of electro-production and HM reduction (Jiang et al., 2020; Yin et al., 2021). Other examples also include cumulative-resistant bacteria like Acinetobacter and Limnohabitans, which are only enriched in the CMFC-A (Dahal et al., 2023; Al-Jabri et al., 2018). Their enrichment directly causes vertical gene transfer and HRG elevation. The Cr forms, soil physicochemical properties, soil enzyme activities, and micro-ecological structure mirrored each other, helping to understand Cr transformation patterns and target the key factors affecting metal resistance changes.
HM existence in soil can induce HGT occurrence and cause ARG elevation, which has become a major concern (Chen et al., 2023b; Fu et al., 2023). Sub-lethal levels of metal ions can increase mutation rates and enrich de novo mutants with significant resistance to multiple antibiotics (Li et al., 2019). This study focused on the toxic alleviation of a single HM (Cr) in the SMFC, during which tolerator accumulation caused considerable HRG enrichment. The SMFC is an eco-friendly and cost-effective technology for the in situ bioremediation of contaminated soil and/or sediment and for the powering of environmental sensors in remote areas. It has the potential to be used as a novel early-warning system for soil environmental hazards. Nevertheless, before the commercialization of large-scale applications in the field, significant efforts should be made to reveal the HRG enrichment mechanism during SMFC operation, and attention should be paid to ARG change under HM contamination or HM–antibiotic co-contamination.
During SMFC operation, the close interaction among the soil physicochemical properties, enzyme activities, resistance genes, and microbial community structures determined the performance. The pre-loading of Fe3O4 in the cathode and of EAB in the anode greatly contributed to power production and Cr(VI) elimination. Anodic microbial metabolism, cathodic redox, and the MFC electric field reduced or immobilized Cr(VI) to eliminate its risk. The enrichment of multiple MRB, such as Acinetobacter, Limnohabitans, and Desulfotomaculum, resulted in HRG elevation, which contributes to microbial adaptation and functioning but brings about concerns for future applications. This study provides a reference for the remediation of HM-contaminated soil using MFC, which is conducive to promoting the practical application of bio-electrochemical technology in the field.
SMFC | Soil microbial fuel cell |
HRGs | Heavy-metal resistance genes |
HMs | Heavy metals |
HGT | Horizontal gene transfer |
EAB | Electrochemical active bacteria |
GF | Graphite felt |
ORR | Oxygen reduction reaction |
WCV | Working-circuit voltage |
OCV | Open-circuit voltage |
ARGs | Antibiotic-resistant genes |
CMFC-A | Anode of the closed-circuit group |
OMFC-A | Anode of the open-circuit group |
CMFC-C | Cathode of the closed-circuit group |
OMFC-C | Cathode of the open-circuit group |
CMFC-S | Soil of the closed-circuit group |
OMFC-S | Soil of the open-circuit group |
NMFC-S | Soil of the non-electrode control group |
The code data that support the findings of this study are available in the Supplement of this article.
The data that support the findings of this study are available on request from the corresponding author. The data are not publicly available due to privacy or ethical restrictions.
The supplement related to this article is available online at https://doi.org/10.5194/soil-11-323-2025-supplement.
HN: conceptualization, investigation, writing, review and editing; XL: investigation and visualization; PL: investigation and visualization; HQ: methodology; LJ: writing (review and editing); SM: writing (review and editing); MW: writing (review); FX: funding acquisition and supervision; HX: funding acquisition and supervision; CW: funding acquisition and supervision.
The contact author has declared that none of the authors has any competing interests.
Publisher's note: Copernicus Publications remains neutral with regard to jurisdictional claims made in the text, published maps, institutional affiliations, or any other geographical representation in this paper. While Copernicus Publications makes every effort to include appropriate place names, the final responsibility lies with the authors.
We thank all anonymous reviewers for their thoughtful and constructive input that helped to improve the paper. We thank Weizhen Fang from the Analysis & Testing Center and Cuijuan Wang from the School of Chemistry, Southwest Jiaotong University, for the technical support. We thank Lijuan Zhang from SCI-Go (https://www.sci-go.com/home, last access: 18 April 2025) for the XPS analysis.
This work was financially supported by the Sichuan Science and Technology Program (grant nos. 2024NSFSC0384 and 2025ZNSFSC0200), the Science and Technology Projects of Xizang Autonomous Region, China (grant no. XZ202501ZY0148), the Government Guides Local Science and Technology Development Project of the Tibetan Autonomous Region of China (grant no. XZ202401YD0001), the China Postdoctoral Science Foundation (grant no. 2022M712630), the grants from the Guizhou Provincial Department of Science and Technology (grant no. E2DF028), and the National Natural Science Foundation of China (grant no. 42207021).
This paper was edited by Rafael Clemente and reviewed by three anonymous referees.
Al-Jabri, Z., Zamudio, R., Horvath-Papp, E., Ralph, J. D., AL-Muharrami, Z., Rajakumar, K., and Oggioni, M. R.: Integrase-Controlled Excision of Metal-Resistance Genomic Islands in Acinetobacter baumannii, Genes-Basel, 9, 366, https://doi.org/10.3390/genes9070366, 2018.
Ashbolt Nicholas, J., Amézquita, A., Backhaus, T., Borriello, P., Brandt Kristian, K., Collignon, P., Coors, A., Finley, R., Gaze William, H., Heberer, T., Lawrence John, R., Larsson, D. G. J., McEwen Scott, A., Ryan James, J., Schönfeld, J., Silley, P., Snape Jason, R., Van den Eede, C., and Topp, E.: Human Health Risk Assessment (HHRA) for Environmental Development and Transfer of Antibiotic Resistance, Environ. Health Persp., 121, 993–1001, https://doi.org/10.1289/ehp.1206316, 2013.
Bokulich, N. A., Kaehler, B. D., Rideout, J. R., Dillon, M., Bolyen, E., Knight, R., Huttley, G. A., and Gregory Caporaso, J.: Optimizing taxonomic classification of marker-gene amplicon sequences with QIIME 2's q2-feature-classifier plugin, Microbiome, 6, 90, https://doi.org/10.1186/s40168-018-0470-z, 2018.
Chen, C., Fang, Y., and Zhou, D.: Selective pressure of PFOA on microbial community: Enrichment of denitrifiers harboring ARGs and the transfer of ferric-electrons, Water Res., 233, 119813, https://doi.org/10.1016/j.watres.2023.119813, 2023a.
Chen, M., Cai, Y., Li, G., Zhao, H., and An, T.: The stress response mechanisms of biofilm formation under sub-lethal photocatalysis, Appl. Catal. B-Environ., 307, 121200, https://doi.org/10.1016/j.apcatb.2022.121200, 2022a.
Chen, P., Zhang, T., Chen, Y., Ma, H., Wang, Y., Liu, W., Wang, Y., Zhou, G., Qing, R., Zhao, Y., Xu, H., Hao, L., Wang, C., and Xu, F.: Integrated Chamber-free Microbial Fuel Cell for Wastewater Purification and Bioenergy Generation, Chem. Eng. J., 442, 136091, https://doi.org/10.1016/j.cej.2022.136091, 2022b.
Chen, X., Du, Z., Song, X., Wang, L., Wei, Z., Jia, L., and Zhao, R.: Evaluating the occurrence frequency of horizontal gene transfer induced by different degrees of heavy metal stress, J. Clean. Prod., 382, 135371, https://doi.org/10.1016/j.jclepro.2022.135371, 2023b.
Chen, Y., Zuo, M., Yang, D., He, Y., Wang, H., Liu, X., Zhao, M., Xu, L., Ji, J., Liu, Y., and Gao, T.: Synergistically Effect of Heavy Metal Resistant Bacteria and Plants on Remediation of Soil Heavy Metal Pollution, Water Air Soil Poll., 235, 296, https://doi.org/10.1007/s11270-024-07100-w, 2024.
Choi, S.: Electrogenic Bacteria Promise New Opportunities for Powering, Sensing, and Synthesizing, Small, 18, 2107902, https://doi.org/10.1002/smll.202107902, 2022.
Coetzee, J. J., Bansal, N., and Chirwa, E. M. N.: Chromium in Environment, Its Toxic Effect from Chromite-Mining and Ferrochrome Industries, and Its Possible Bioremediation, Expos. Health, 12, 51–62, https://doi.org/10.1007/s12403-018-0284-z, 2020.
Cong, Y., Shen, L., Wang, B., Cao, J., Pan, Z., Wang, Z., Wang, K., Li, Q., and Li, X.: Efficient removal of Cr(VI) at alkaline pHs by sulfite/iodide/UV: Mechanism and modeling, Water Res., 222, 118919, https://doi.org/10.1016/j.watres.2022.118919, 2022.
Dahal, U., Paul, K., and Gupta, S.: The multifaceted genus Acinetobacter: from infection to bioremediation, J. Appl. Microbiol., 134, lxad145, https://doi.org/10.1093/jambio/lxad145, 2023.
Deng, Y., Jiang, Y.-H., Yang, Y., He, Z., Luo, F., and Zhou, J.: Molecular ecological network analyses, BMC Bioinformatics, 13, 113, https://doi.org/10.1186/1471-2105-13-113, 2012.
Dimova, M., Iutynska, G., Yamborko, N., Dordevic, D., and Kushkevych, I.: Possible Processes and Mechanisms of Hexachlorobenzene Decomposition by the Selected Comamonas testosteroni Bacterial Strains, Processes, 10, 2170, https://doi.org/10.3390/pr10112170, 2022.
Ernst, C., Kayastha, K., Koch, T., Venceslau, S. S., Pereira, I. A. C., Demmer, U., Ermler, U., and Dahl, C.: Structural and spectroscopic characterization of a HdrA-like subunit from Hyphomicrobium denitrificans, FEBS J., 288, 1664–1678, https://doi.org/10.1111/febs.15505, 2021.
Fan, C., Qian, J., Yang, Y., Sun, H., Song, J., and Fan, Y.: Green ceramsite production via calcination of chromium contaminated soil and the toxic Cr(VI) immobilization mechanisms, J. Clean. Prod., 315, 128204, https://doi.org/10.1016/j.jclepro.2021.128204, 2021.
Fan, Q., Fan, L., Quach, W.-M., Zhang, R., Duan, J., and Sand, W.: Application of microbial mineralization technology for marine concrete crack repair: A review, J. Building Engineering, 69, 106299, https://doi.org/10.1016/j.jobe.2023.106299, 2023.
Farkas, D., Proctor, K., Kim, B., Avignone Rossa, C., Kasprzyk-Hordern, B., and Di Lorenzo, M.: Assessing the impact of soil microbial fuel cells on atrazine removal in soil, J. Hazard. Mater., 478, 135473, https://doi.org/10.1016/j.jhazmat.2024.135473, 2024.
Faust, K. and Raes, J.: Microbial interactions: from networks to models, Nat. Rev. Microbiol., 10, 538–550, https://doi.org/10.1038/nrmicro2832, 2012.
Feng, H., Jin, A., Yin, X., Hong, Z., Ding, Y., Zhao, N., Chen, Y., and Zhang, Y.: Enhancing biocathode denitrification performance with nano-Fe3O4 under polarity period reversal, Environ. Res., 241, 117641, https://doi.org/10.1016/j.envres.2023.117641, 2024.
Fu, Y., Zhu, Y., Dong, H., Li, J., Zhang, W., Shao, Y., and Shao, Y.: Effects of heavy metals and antibiotics on antibiotic resistance genes and microbial communities in soil, Process Saf. Environ., 169, 418–427, https://doi.org/10.1016/j.psep.2022.11.020, 2023.
Guo, S., Xiao, C., Zhou, N., and Chi, R.: Speciation, toxicity, microbial remediation and phytoremediation of soil chromium contamination, Environ. Chem. Lett., 19, 1413–1431, https://doi.org/10.1007/s10311-020-01114-6, 2021.
Gupta, S., Patro, A., Mittal, Y., Dwivedi, S., Saket, P., Panja, R., Saeed, T., Martínez, F., and Yadav, A. K.: The race between classical microbial fuel cells, sediment-microbial fuel cells, plant-microbial fuel cells, and constructed wetlands-microbial fuel cells: Applications and technology readiness level, Sci. Total Environ., 879, 162757, https://doi.org/10.1016/j.scitotenv.2023.162757, 2023.
Gustave, W., Yuan, Z.-F., Li, X., Ren, Y.-X., Feng, W.-J., Shen, H., and Chen, Z.: Mitigation effects of the microbial fuel cells on heavy metal accumulation in rice (Oryza sativa L.), Environ. Pollut., 260, 113989, https://doi.org/10.1016/j.envpol.2020.113989, 2020.
Hamdan, H. Z. and Salam, D. A.: Sediment microbial fuel cells for bioremediation of pollutants and power generation: a review, Environ. Chem. Lett., 21, 2761–2787, https://doi.org/10.1007/s10311-023-01625-y, 2023.
He, Q., Gui, J., Liu, D., Li, X., Li, P., and Quan, S.: Research progress of soil property's changes and its impacts on soil cadmium activity in flooded paddy field, J. Agro-Environ. Sci., 35, 2260–2268, https://doi.org/10.11654/jaes.2016-0892, 2016.
He, S., Guo, H., He, Z., Yang, C., Yu, T., Chai, Q., and Lu, L.: Interaction of Lolium perenne and Hyphomicrobium sp. GHH enhances the removal of 17α-ethinyestradiol (EE2) from soil, J. Soil. Sediment., 19, 1297–1305, https://doi.org/10.1007/s11368-018-2116-y, 2019.
Hernández-Ramírez, K. C., Reyes-Gallegos, R. I., Chávez-Jacobo, V. M., Díaz-Magaña, A., Meza-Carmen, V., and Ramírez-Díaz, M. I.: A plasmid-encoded mobile genetic element from Pseudomonas aeruginosa that confers heavy metal resistance and virulence, Plasmid, 98, 15–21, https://doi.org/10.1016/j.plasmid.2018.07.003, 2018.
Irankhah, S., Abdi Ali, A., Mallavarapu, M., Soudi, M. R., Subashchandrabose, S., Gharavi, S., and Ayati, B.: Ecological role of Acinetobacter calcoaceticus GSN3 in natural biofilm formation and its advantages in bioremediation, Biofouling, 35, 377–391, https://doi.org/10.1080/08927014.2019.1597061, 2019.
Jia, J., Bai, J., Xiao, R., Tian, S., Wang, D., Wang, W., Zhang, G., Cui, H., and Zhao, Q.: Fractionation, source, and ecological risk assessment of heavy metals in cropland soils across a 100 year reclamation chronosequence in an estuary, South China, Sci. Total Environ., 807, 151725, https://doi.org/10.1016/j.scitotenv.2021.151725, 2022.
Jiang, Y., Shang, Y., Gong, T., Hu, Z., Yang, K., and Shao, S.: High concentration of Mn2+ has multiple influences on aerobic granular sludge for aniline wastewater treatment, Chemosphere, 240, 124945, https://doi.org/10.1016/j.chemosphere.2019.124945, 2020.
Kim, C., Lee, C. R., Song, Y. E., Heo, J., Choi, S. M., Lim, D.-H., Cho, J., Park, C., Jang, M., and Kim, J. R.: Hexavalent chromium as a cathodic electron acceptor in a bipolar membrane microbial fuel cell with the simultaneous treatment of electroplating wastewater, Chem. Eng. J., 328, 703–707, https://doi.org/10.1016/j.cej.2017.07.077, 2017.
Li, C., Huang, H., Gu, X., Zhong, K., Yin, J., Mao, J., Chen, J., and Zhang, C.: Accumulation of heavy metals in rice and the microbial response in a contaminated paddy field, J. Soil. Sediment., 24, 644–656, https://doi.org/10.1007/s11368-023-03643-3, 2023a.
Li, X., Gu, A. Z., Zhang, Y., Xie, B., Li, D., and Chen, J.: Sub-lethal concentrations of heavy metals induce antibiotic resistance via mutagenesis, J. Hazard. Mater., 369, 9–16, https://doi.org/10.1016/j.jhazmat.2019.02.006, 2019.
Li, Y., Chen, Y., Chen, Y., Qing, R., Cao, X., Chen, P., Liu, W., Wang, Y., Zhou, G., Xu, H., Hao, L., Wang, C., Li, S., Zhu, Y., Haderlein, S., and Xu, F.: Fast deployable real-time bioelectric dissolved oxygen sensor based on a multi-source data fusion approach, Chem. Eng. J., 475, 146064, https://doi.org/10.1016/j.cej.2023.146064, 2023b.
Li, Y., Lin, J., Wu, Y., Jiang, S., Huo, C., Liu, T., Yang, Y., and Ma, Y.: Transformation of exogenous hexavalent chromium in soil: Factors and modelling, J. Hazard. Mater., 480, 135799, https://doi.org/10.1016/j.jhazmat.2024.135799, 2024.
Lin, B., Hyacinthe, C., Bonneville, S., Braster, M., Van Cappellen, P., and Röling, W. F. M.: Phylogenetic and physiological diversity of dissimilatory ferric iron reducers in sediments of the polluted Scheldt estuary, Northwest Europe, Environ. Microbiol., 9, 1956–1968, https://doi.org/10.1111/j.1462-2920.2007.01312.x, 2007.
Liu, H., Xu, F., Xie, Y., Wang, C., Zhang, A., Li, L., and Xu, H.: Effect of modified coconut shell biochar on availability of heavy metals and biochemical characteristics of soil in multiple heavy metals contaminated soil, Sci. Total Environ., 645, 702–709, https://doi.org/10.1016/j.scitotenv.2018.07.115, 2018.
Liu, S., Pu, S., Deng, D., Huang, H., Yan, C., Ma, H., and Razavi, B. S.: Comparable effects of manure and its biochar on reducing soil Cr bioavailability and narrowing the rhizosphere extent of enzyme activities, Environ. Int., 134, 105277, https://doi.org/10.1016/j.envint.2019.105277, 2020.
Liu, S., Feng, Y., and Li, H.: Degradation mechanism of saliferous compounding heavy metals-organic wastewater by manganese and iron cycling in the microbial fuel cell, Chem. Eng. J., 473, 145389, https://doi.org/10.1016/j.cej.2023.145389, 2023a.
Liu, X.-C., Zhang, K.-X., Song, J.-S., Zhou, G.-N., Li, W.-Q., Ding, R.-R., Wang, J., Zheng, X., Wang, G., and Mu, Y.: Tuning Fe3O4 for sustainable cathodic heterogeneous electro-Fenton catalysis by acetylated chitosan, P. Natl. Acad. Sci. USA, 120, e2213480120, https://doi.org/10.1073/pnas.2213480120, 2023b.
Liu, Z., Zhao, Y., Zhang, B., Wang, J., Zhu, L., and Hu, B.: Deterministic Effect of pH on Shaping Soil Resistome Revealed by Metagenomic Analysis, Environ. Sci. Technol., 57, 985–996, https://doi.org/10.1021/acs.est.2c06684, 2023c.
Ma, S., Mao, S., Shi, J., Zou, J., Zhang, J., Liu, Y., Wang, X., Ma, Z., and Yu, C.: Exploring the synergistic interplay of sulfur metabolism and electron transfer in Cr(VI) and Cd(II) removal by Clostridium thiosulfatireducens: Genomic and mechanistic insights, Chemosphere, 352, 141289, https://doi.org/10.1016/j.chemosphere.2024.141289, 2024.
Mandal, S., Sarkar, B., Bolan, N., Ok, Y. S., and Naidu, R.: Enhancement of chromate reduction in soils by surface modified biochar, J. Environ. Manage., 186, 277–284, https://doi.org/10.1016/j.jenvman.2016.05.034, 2017.
Men, C., Liu, R., Xu, F., Wang, Q., Guo, L., and Shen, Z.: Pollution characteristics, risk assessment, and source apportionment of heavy metals in road dust in Beijing, China, Sci. Total Environ., 612, 138–147, https://doi.org/10.1016/j.scitotenv.2017.08.123, 2018.
Morais, P. V., Branco, R., and Francisco, R.: Chromium resistance strategies and toxicity: what makes Ochrobactrum tritici 5bvl1 a strain highly resistant, BioMetals, 24, 401–410, https://doi.org/10.1007/s10534-011-9446-1, 2011.
Morales-Benítez, I., Montoro-Leal, P., García-Mesa, J. C., López Guerrero, M. M., and Vereda Alonso, E.: New magnetic chelating sorbent for chromium speciation by magnetic solid phase extraction on-line with inductively coupled plasma optical emission spectrometry, Talanta, 256, 124262, https://doi.org/10.1016/j.talanta.2023.124262, 2023.
Qian, X., Fang, C., Huang, M., and Achal, V.: Characterization of fungal-mediated carbonate precipitation in the biomineralization of chromate and lead from an aqueous solution and soil, J. Clean. Prod., 164, 198–208, https://doi.org/10.1016/j.jclepro.2017.06.195, 2017.
Rani, L., Kaushal, J., and Lal Srivastav, A.: Biochar as sustainable adsorbents for chromium ion removal from aqueous environment: a review, Biomass Convers. Biorefin., 14, 6083–6096, https://doi.org/10.1007/s13399-022-02784-8, 2022.
Rouch, D. A., Lee, B. T. O., and Morby, A. P.: Understanding cellular responses to toxic agents: a model for mechanism-choice in bacterial metal resistance, J. Ind. Microbiol., 14, 132–141, https://doi.org/10.1007/BF01569895, 1995.
Sardans, J. and Peñuelas, J.: Drought decreases soil enzyme activity in a Mediterranean Quercus ilex L. forest, Soil Biol. Biochem., 37, 455–461, https://doi.org/10.1016/j.soilbio.2004.08.004, 2005.
Shatalin, K., Nuthanakanti, A., Kaushik, A., Shishov, D., Peselis, A., Shamovsky, I., Pani, B., Lechpammer, M., Vasilyev, N., Shatalina, E., Rebatchouk, D., Mironov, A., Fedichev, P., Serganov, A., and Nudler, E.: Inhibitors of bacterial H2S biogenesis targeting antibiotic resistance and tolerance, Science, 372, 1169–1175, https://doi.org/10.1126/science.abd8377, 2021.
Sundarraj, S., Sudarmani, D. N. P., Samuel, P., and Sevarkodiyone, S. P.: Bioremediation of hexavalent chromium by transformation of Escherichia coli DH5α with chromate reductase (ChrR) genes of Pseudomonas putida isolated from tannery effluent, J. Appl. Microbiol., 134, lxac019, https://doi.org/10.1093/jambio/lxac019, 2023.
Tan, H., Wang, C., Zeng, G., Luo, Y., Li, H., and Xu, H.: Bioreduction and biosorption of Cr(VI) by a novel Bacillus sp. CRB-B1 strain, J. Hazard. Mater., 386, 121628, https://doi.org/10.1016/j.jhazmat.2019.121628, 2020.
Thapa, B. S., Kim, T., Pandit, S., Song, Y. E., Afsharian, Y. P., Rahimnejad, M., Kim, J. R., and Oh, S.-E.: Overview of electroactive microorganisms and electron transfer mechanisms in microbial electrochemistry, Bioresource Technol., 347, 126579, https://doi.org/10.1016/j.biortech.2021.126579, 2022.
van Hoek, A. H., Mevius, D., Guerra, B., Mullany, P., Roberts, A. P., and Aarts, H. J.: Acquired Antibiotic Resistance Genes: An Overview, Front. Microbiol., 2, 203, https://doi.org/10.3389/fmicb.2011.00203, 2011.
Wang, C., Zhou, Z., Liu, H., Li, J., Wang, Y., and Xu, H.: Application of acclimated sewage sludge as a bio-augmentation/bio-stimulation strategy for remediating chlorpyrifos contamination in soil with/without cadmium, Sci. Total Environ., 579, 657–666, https://doi.org/10.1016/j.scitotenv.2016.11.044, 2017.
Wang, C., Li, Y. Z., Tan, H., Zhang, A. K., Xie, Y. L., Wu, B., and Xu, H.: A novel microbe consortium, nano-visible light photocatalyst and microcapsule system to degrade PAHs, Chem. Eng. J., 359, 1065–1074, https://doi.org/10.1016/j.cej.2018.11.077, 2019.
Wang, C., Tan, H., Li, H., Xie, Y., Liu, H., Xu, F., and Xu, H.: Mechanism study of Chromium influenced soil remediated by an uptake-detoxification system using hyperaccumulator, resistant microbe consortium, and nano iron complex, Environ. Pollut., 257, 113558, https://doi.org/10.1016/j.envpol.2019.113558, 2020a.
Wang, C., Jia, Y., Li, J., Wang, Y., Niu, H., Qiu, H., Li, X., Fang, W., and Qiu, Z.: Effect of bioaugmentation on tetracyclines influenced chicken manure composting and antibiotics resistance, Sci. Total Environ., 867, 161457, https://doi.org/10.1016/j.scitotenv.2023.161457, 2023a.
Wang, C., Jia, Y., Li, J., Li, P., Wang, Y., Yan, F., Wu, M., Fang, W., Xu, F., and Qiu, Z.: Influence of microbial augmentation on contaminated manure composting: metal immobilization, matter transformation, and bacterial response, J. Hazard. Mater., 441, 129762, https://doi.org/10.1016/j.jhazmat.2022.129762, 2023b.
Wang, K., Jia, R., Li, L., Jiang, R., and Qu, D.: Community structure of Anaeromyxobacter in Fe(III) reducing enriched cultures of paddy soils, J. Soil. Sediment., 20, 1621–1631, https://doi.org/10.1007/s11368-019-02529-7, 2020b.
Wang, Q., Song, X., Wei, C., Jin, P., Chen, X., Tang, Z., Li, K., Ding, X., and Fu, H.: In situ remediation of Cr(VI) contaminated groundwater by ZVI-PRB and the corresponding indigenous microbial community responses: a field-scale study, Sci. Total Environ., 805, 150260, https://doi.org/10.1016/j.scitotenv.2021.150260, 2022.
Yaashikaa, P. R., Kumar, P. S., Jeevanantham, S., and Saravanan, R.: A review on bioremediation approach for heavy metal detoxification and accumulation in plants, Environ. Pollut., 301, 119035, https://doi.org/10.1016/j.envpol.2022.119035, 2022.
Yang, Y., Wang, H., Zheng, Y., Zhu, B., Wu, X., and Zhao, F.: Extracellular electron transfer of Methylophilus methylotrophs, Process Biochem., 94, 313–318, https://doi.org/10.1016/j.procbio.2020.05.001, 2020.
Yin, Y., Chen, Y., and Wang, J.: Co-fermentation of sewage sludge and algae and Fe2+ addition for enhancing hydrogen production, Int. J. Hydrogen Energ., 46, 8950–8960, https://doi.org/10.1016/j.ijhydene.2021.01.009, 2021.
Zhang, J., Liu, Y., Sun, Y., Wang, H., Cao, X., and Li, X.: Effect of soil type on heavy metals removal in bioelectrochemical system, Bioelectrochemistry, 136, 107596, https://doi.org/10.1016/j.bioelechem.2020.107596, 2020.
Zhang, Y., Shen, G., Hu, S., He, Y., Li, P., and Zhang, B.: Deciphering of antibiotic resistance genes (ARGs) and potential abiotic indicators for the emergence of ARGs in an interconnected lake-river-reservoir system, J. Hazard. Mater., 410, 124552, https://doi.org/10.1016/j.jhazmat.2020.124552, 2021.
Zhang, Y., Zheng, S., Hao, Q., Wang, O., and Liu, F.: Respiratory electrogen Geobacter boosts hydrogen production efficiency of fermentative electrotroph Clostridium pasteurianum, Chem. Eng. J., 456, 141069, https://doi.org/10.1016/j.cej.2022.141069, 2023.
Zhu, J., Zhang, T., Zhu, N., Feng, C., Zhou, S., and Dahlgren, R. A.: Bioelectricity generation by wetland plant-sediment microbial fuel cells (P-SMFC) and effects on the transformation and mobility of arsenic and heavy metals in sediment, Environ. Geochem. Hlth., 41, 2157–2168, https://doi.org/10.1007/s10653-019-00266-x, 2019.