the Creative Commons Attribution 4.0 License.
the Creative Commons Attribution 4.0 License.
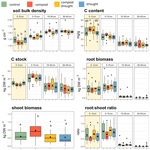
Experimental drought and soil amendments affect grassland above- and belowground vegetation but not soil carbon stocks
Daniela Guasconi
Sara A. O. Cousins
Stefano Manzoni
Nina Roth
Gustaf Hugelius
Soils are the largest terrestrial carbon (C) pool on the planet, and targeted grassland management has the potential to increase grassland C sequestration. Appropriate land management strategies, such as organic matter addition, can increase soil C stocks and improve grasslands' resilience to drought by improving soil water retention and infiltration. However, soil carbon dynamics are closely tied to vegetation responses to management and climatic changes, which affect roots and shoots differently. This study presents findings from a 3-year field experiment on two Swedish grasslands that assessed the impact of compost amendment and experimental drought on plant biomass and soil C to a depth of 45 cm. Aboveground biomass and soil C content (% C) increased compared with untreated controls in compost-amended plots; however, because bulk density decreased, there was no significant effect on soil C stocks. Experimental drought did not significantly reduce plant biomass compared to control plots, but it stunted the increase in aboveground biomass in compost-treated plots and led to changes in root traits. These results highlight the complexity of ecosystem C dynamics and the importance of considering multiple biotic and abiotic factors across spatial scales when developing land management strategies to enhance C sequestration.
- Article
(2018 KB) - Full-text XML
-
Supplement
(856 KB) - BibTeX
- EndNote
Soil management has been receiving increasing attention in the past years, with a growing awareness that soils provide vital ecosystem services and can act as carbon (C) sinks (Minasny et al., 2017; European Commission, 2020). The soil–plant system is integral to this process, as plants capture atmospheric carbon dioxide through photosynthesis and transfer C to the soil via root exudates and decaying above- and belowground plant necromass. Concerns about soil erosion and historic soil C depletion in agricultural and grassland soils (Sanderman et al., 2017; Bai and Cotrufo, 2022) have motivated the development of sustainable land management strategies, generally named “carbon farming” (Paul et al., 2023) and promoted by initiatives aimed at increasing the C stored in soils, such as the “4 per 1000” initiative (Minasny et al., 2017). These strategies also contribute to mitigating anthropogenic greenhouse gas (GHG) emissions by restoring soil organic carbon (SOC) stocks. SOC restoration can be achieved by decreasing tillage; adopting cover crops; and using soil C amendments, like compost, biochar, and manure, on croplands or grasslands (Ryals and Silver 2013; Ryals et al., 2015; Keesstra et al., 2016; Fischer et al., 2019; Garbowski et al., 2023). Soil C management via compost amendments, as in Ryals and Silver (2013), aims to facilitate the accumulation of plant-derived C SOC, which can be retained over long timescales – i.e., decades to centuries (Shi et al., 2020). If the total C inputs and accumulation in the soil exceed the total losses, C amendments can lead to C sequestration (Don et al., 2024; Moinet et al., 2023).
As the C sequestration potential is uncertain and context dependent (Paltineanu et al., 2024), it is important to investigate the effects of C amendments across a range of climatic and management conditions. Grasslands, including croplands converted to grasslands, can store considerable amounts of soil C (Johansson et al., 2023) and are, therefore, ideal systems to apply C amendments. They can act as C sinks with improved management (Conant et al., 2001) and can have higher root biomass C compared with agricultural lands, the latter of which are usually cultivated with annual crops (Beniston et al., 2014). Several studies have investigated the effects of organic amendments on aboveground biomass (Ryals et al., 2016), crop yields (Luo et al., 2018; Ahmad et al., 2009), and roots in farming systems (Hirte et al., 2021), but fewer studies have focused on noncultivated grasslands.
C amendments add C to the soil in two ways: directly, by moving plant biomass from one location to another, and indirectly, by promoting plant growth (Ryals et al., 2016). Compost is rich in organic matter, which serves as a substrate for soil microorganisms. As microbes decompose this organic matter, they release nutrients in forms that plants can readily absorb (Malik et al., 2013). In turn, higher vegetation growth can increase the natural rate of C input and, thus, potentially SOC stocks (Ryals and Silver, 2013). Indeed, model predictions suggest that compost addition on grasslands can lead to soil C sequestration via an increase in plant biomass (DeLonge et al., 2013). By improving the soil structure and reducing compaction, compost addition may also reduce the soil bulk density (Brown and Cotton, 2011). As SOC stocks are calculated by multiplying the C concentration by the bulk density, improved management may also lead to net-zero effects on the C stock, despite increased soil C contents. Considering these indirect effects requires an ecosystem-level perspective on the C sequestration potential of soils that accounts for (1) below- and aboveground vegetation contributions to soil C stocks and (2) the soil depth at which management effects are detectable. Here, we adopt this broad perspective and assess changes in C stocks in both soil and vegetation after C amendments.
Compost amendments can impact both above- and belowground plant biomass, but these plant components contribute differently to SOC storage. Root biomass and root exudates are critical to soil C formation and retention (Jackson et al., 2017), as roots are more recalcitrant to decomposition compared with shoots (Rasse et al., 2005; Gaudinski et al., 2000). However, aboveground plant biomass also impacts soil C stocks, and potential trade-offs in above- vs. belowground C allocation within the vegetation pool should be included in ecosystem C balance assessments (Hayes et al., 2017). Above- and belowground biomass may also respond differently to soil amendments (Garbowski et al., 2020). This variation is expected, as roots and shoots respond differently to changes in nutrients (Hayes et al., 2017). Therefore, an approach that accounts for above- and belowground interactions is essential to understand the proportion of plant litter contributing to soil organic matter (SOM) formation and stabilization (Cotrufo et al., 2015) and to achieve a comprehensive understanding of ecosystem C dynamics (Heimann and Reichstein, 2008).
Soil organic amendments can also help mitigate the negative effects of drought on vegetation and soil microbial communities by increasing water retention in the soil (Fischer et al., 2019; Haque et al., 2021). Future climate projections indicate an increase in extreme weather events, including longer and more frequent droughts (IPCC, 2021). These conditions may decrease vegetation growth both above- and belowground (Guasconi et al., 2023) and decrease plant carbon allocation to aboveground organs (Hasibeder et al., 2015), leading to lower C inputs to the soil and potentially decreased soil C stocks (Deng et al., 2021). The effects of organic amendments on water retention are modulated by soil texture, by the quantity and quality of soil organic matter (Rawls et al., 2003; Yang et al., 2014; Franco-Andreu et al., 2017; Sarker et al., 2022), and by their chemical composition (Franco-Andreu et al., 2017). Increased water retention can also indirectly benefit the ecosystem C balance by partly compensating for the drought-induced loss of plant biomass (Kallenbach et al., 2019; Ali et al., 2017). These expected positive effects of organic amendments are not always observed, as soil and plant communities exhibit large variability in their response to both drought (Guasconi et al., 2023; Canarini et al., 2017) and soil amendments (Gebhardt et al., 2017). This variability stems partly from the different physical properties of the soil. However, it can also be influenced by factors such as land use history and both small- and large-scale topography (Wang et al., 2020). These complexities highlight the need for more field-based data collection campaigns – in particular under experimental conditions that combine soil amendments and drought.
Here, we present the results of a field experiment designed to assess the effects of compost and experimental drought on both soil and plant biomass after three growing seasons. Changes were observed along the soil profile to a depth of 45 cm in two grasslands and at two catenary positions, i.e., at the top and at the bottom of a slope. We tested the following hypotheses:
-
Compost amendment increases the soil C content and plant growth (with both having positive effects on C stocks), while decreasing soil bulk density (which has a negative effect on C stocks); we expect that these mechanisms have counteracting effects on net soil C storage.
-
Drought has a weak negative or undetectable effect on SOC by decreasing both productivity (organic C input) and respiration (microbial decomposition of SOM).
-
Compost amendment mitigates the loss of soil moisture under drought conditions, which may alleviate plant growth reductions under these conditions.
2.1 Site description and experimental setup
The experimental site was established in summer 2019 in the proximity of Tovetorp Research Station south of Stockholm, Sweden, and consists of two fields that were formerly cropland (hereafter referred to as “Tovetorp” and “Ämtvik”, respectively), each with an upper and a lower catenary position (hereafter referred to as “high” and “low”, respectively). Today, the land management regime consists of cow grazing and hay production (see Roth, 2023). The soil at all locations is rich in clay and ranges from silty clay to silty loam (Table S1).
At each of these four locations, four treatments – compost, drought, drought × compost, and control (ambient precipitation and no compost treatment) – were applied using three replicates. This resulted in 12 plots per location and 48 plots in total. Each plot measured 2 m ×2 m. Because the effects of already partly decomposed organic amendments can be expected to be longer-lasting than those of easily decomposable ones (Sarker et al., 2022), we applied a one-time compost treatment combined with a growing season drought and investigated the effects on the soil C stocks after three full growing seasons. The compost was made of Zea mays and had a C : N ratio of 9.8 and a δ13C value of about −15.39 ‰. After the seasonal corn harvest (August 2019), the green parts of the plants were collected in an open field. The piled material was regularly stirred to promote the composting process, and the resulting compost was collected and applied in mid-February 2020 as a thin surface layer of ca. 11 kg m−2 (wet weight), similar to the procedure described in Ryals and Silver (2013). The total amount of C added was ∼ 0.54 kg C m−2 on average. The δ13C isotope ratio of the compost was higher than that of bulk soil (−15.39 and −27.25, respectively), which means that the δ13C isotope ratios of different treatments can be used to assess if and where (in the soil) the compost material was retained after the 3 years of treatment.
The drought treatment followed the guidelines of the Drought-Net Research Coordination Network (Knapp et al., 2017; Yahdjian and Sala, 2002) and consisted of 12 rainout shelters (3 per location) with roofs made out of evenly placed V-shaped polycarbonate strips designed to exclude 60 % of the precipitation during the entire growing season (which were in place from the beginning of July to the end of October in 2019 and from the beginning of April to the end of October in 2020, 2021, and 2022). This precipitation reduction corresponds to the first quantile of the local 100-year precipitation record (Swedish Meteorological and Hydrological Institute, 2021). Each shelter covered two plots, one for the drought treatment and one for the combined drought × compost treatment. A rubber sheet was inserted in the soil around each shelter, at approximately 40 cm depth, to isolate the study plots from the ambient soil moisture. Pictures and sketches of the sites and of the experimental design are presented in Roth et al. (2023). Total annual precipitation during the study years was retrieved from the records of Tovetorp Research Station (Table S2). We note that, while the precipitation in the growing seasons of 2019 and 2022 (April through August) was roughly the same (157 and 156 mm, respectively), the 2019 sampling followed an extremely dry summer in 2018, during which the study area received only 77 mm of precipitation, about half of the precipitation compared with the 1961–1990 average (historical data from Swedish Meteorological and Hydrological Institute, 2021). Conversely, the 2022 sampling followed a very wet year in 2021, during which time the area received almost 140 % of the normal precipitation over the same time period (250 mm).
2.2 Soil and vegetation sampling and analyses
Soil and root samples were collected in three replicates from each of the four sites and treatments (one sampling per plot) at the end of the first growing season in 2019 (August–September) and again at the end of the experiment in 2022 (August and October). Samples for soil bulk density were collected with a large fixed-volume root auger with a sharpened cutting edge (8 cm diameter and 15 cm in length; Eijkelkamp, the Netherlands). Three 15 cm segments were collected sequentially using the same hole, reaching a total depth of 45 cm. Upon extraction, the cores were cut into 5 cm segments, and the bulk density was determined after drying the samples at 105 °C. Following drying, a subsample from the same core was used to calculate the soil organic matter (SOM) content via the loss on ignition at 550 °C for 4 h. A subset was further burned at 960 °C for 2 h in order to determine the presence of inorganic C, which was low (0.5 %), indicating that the total C can be considered equal to organic C (OC). Samples for total C, total N, and δ13C were taken to a depth of 1 m with a Pürckhauer soil corer (2.5 cm diameter; Eijkelkamp, the Netherlands) in 5 cm increments. The analyses for the total C and N contents and for δ13C were carried out on a subset of the samples at the Stable Isotope Facility at UC Davis (California, USA). A subset of these samples were sent to a commercial lab and used for pH measurements (measured in the commercial lab using distilled water with a Mantech AutoMax 73; Guelph, ON, Canada) and nutrient content analyses (P, Ca, Mg, and K; Avio 500 ICP optical emission spectrometer, PerkinElmer, Waltham, MA, USA) (Table S3). Soil moisture was measured every 3 weeks throughout the growing season (2019 through 2022) from one access tube (1 m long) permanently installed in each plot, using a PR2 profile probe (Delta-T Devices Ltd, Cambridge, UK). The values used in the analyses are growing season averages of volumetric soil water content (%) in the first 30 cm of each plot.
Root biomass was collected in September 2019 and in August 2022 using one soil core sampled with a root auger (8 cm diameter; Eijkelkamp, the Netherlands), by placing the auger on top of the plants, but living aboveground plant biomass and fresh litter were removed and not included in the soil samples. Samples were taken to a depth of 30 cm in all plots and to a depth of 45 cm in a subsample of 16 plots (used as a control for the maximum rooting depth), with soil cores divided into 5 cm segments. The roots were rinsed with water on a 0.5 mm mesh sieve to remove soil; placed on a transparent tray, covered with water, and scanned with a flatbed scanner (Epson Expression 10000XL, Epson Europe Electronics GmbH, Germany) at 600 dpi (grayscale); and then dried at 60 °C for 48 h to obtain the dry weight. The scanned images were analyzed with WinRHIZO (Regent Instruments, Québec, QC, Canada) to obtain the root volume, length, and diameter used to calculate the root mass density (in grams of roots per cubic centimeter of soil), specific root length (in centimeters per gram of roots), and root tissue density (in grams of roots per cubic centimeter of roots). Aboveground biomass was harvested from one-quarter (1 m2) of each plot every year in mid-July, by cutting at ground level (including moss and dead biomass; Table S4). More details of the sampling design are presented in the Supplement (Table S5).
2.3 Data analyses and statistics
Because of the sensitivity of vegetation to natural variability in precipitation (Liu et al., 2020) and the potential effects of landscape heterogeneity on both soil C dynamics and plant growth (Sharma et al., 2022; Guo et al., 2018), the analyses include testing for differences in the control plots between the start and the end of the experiment as well as quantifying the variability given by grassland and catenary position, which we expect might lead to variations in all C pools. The measured soil organic C contents (mass of C per unit mass of soil) at different depths within the soil profile were used to calculate soil C stocks (C content × bulk density × layer thickness). The soil C stocks were then normalized by soil sample thickness (kg m−3) to allow for comparisons among soil layers with different thickness. Because C contents were not measured in all samples, a regression was performed to calculate SOC from SOM data (which were available for all samples) and, thus, obtain a complete dataset:
where SOC and SOM are expressed in kilograms per square meter (kg m−2; Fig. S1).
The fraction F of compost-derived C detected in the soil in the year 2022 was calculated with a two-end-member mixing model, as in Poeplau et al. (2023):
where δ13C was measured in both compost-amended (compost or compost × drought) and control (no compost or drought × no compost) plots.
All of the results and statistical analyses are limited to the depth range of 0–45 cm. This is because the aforementioned soil depth contains the majority of the root biomass (95 % within the first 30 cm, mean ∼ 17 cm), and no effect of treatments could be detected below this range (data not shown).
All analyses were carried out in R (version 3.3.3; R Core Team, 2021), and statistical models were designed with the lmer function (lme4 package). Pairwise comparisons between categorical variables were undertaken with lsmeans (emmeans package), and P values (α=0.05) were obtained with the ANOVA function and the lmerTest package. Residuals from the models were checked graphically. Effect sizes were obtained by calculating Cohen's d with the following formula:
where and are mean values for the two groups for which the effect size is calculated, and S is the standard deviation.
The effect of the treatments was tested on all plots from the 2022 dataset. Values for root biomass and root traits were log-transformed first. The model included compost (categorical variable), drought (categorical variable), and sampling depth (continuous variable) as fixed factors and plot (nested within site) as the random factor. Cohen's d was calculated using the standard deviation of the control group. The effect of the compost amendment on the δ13C ratio was tested with a mixed linear model that included compost and depth as fixed factors and plot (nested within site) as the random factor. Changes in soil C, bulk density, and C stocks were also tested with a model using depth as the categorical variable, to assess if changes occurred at specific depths. The variability in plant biomass and soil properties across locations was tested on all data collected in 2019 and from the control plots in 2022. The model included grassland site, catenary position, and sampling depth (continuous variable) as fixed factors and year and plot as the random factors. Cohen's d was calculated using the standard deviation pooled from all groups. Temporal changes during the experiment not caused by the treatments were tested using data obtained in 2019 and 2022 from the control plots. The model included year and sampling depth (continuous variable) as fixed factors and plot (nested within site) as the random factor. Cohen's d was calculated using the standard deviation of the 2019 dataset. The variable depth was not included in the models for aboveground biomass.
The drought treatment decreased soil moisture by 16 % in the upper 0–30 cm during the growing season (Fig. S2). The effect of drought was consistent over sites, years, and seasons, and there were no statistically significant differences in the drought-driven soil moisture loss between locations; between years; or between springs (April–May), summers (June–July–August), or growing seasons (April–August). There was also no significant difference in soil moisture decrease between drought plots and drought × compost plots (Fig. S3). Additionally, the compost addition did not have any significant effect on soil pH or soil P, Ca, Mg, or K. The compost addition did, however, raise the value of δ13C in the treated plots (mean control plots = −27.44 ‰, whereas mean compost plots = −27.10 ‰; P < 0.01), and the difference was significant in the 0–5 cm, 30–35 cm, and 40–45 cm layers. The mixing model (Eq. 2) indicated that, after three growing seasons, the percentage of compost-derived C in the compost plots was 3.43 % in the 0–5 cm layer, 4.88 % in the 30–35 cm layer, and 5.51 % in the 40–45 cm layer. In the compost × drought plots, the percentage of compost-derived C was 4.55 % in the 0–5 cm layer, 6.52 % in the 30–35 cm layer, and 2.96 % in the 40–45 cm layer.
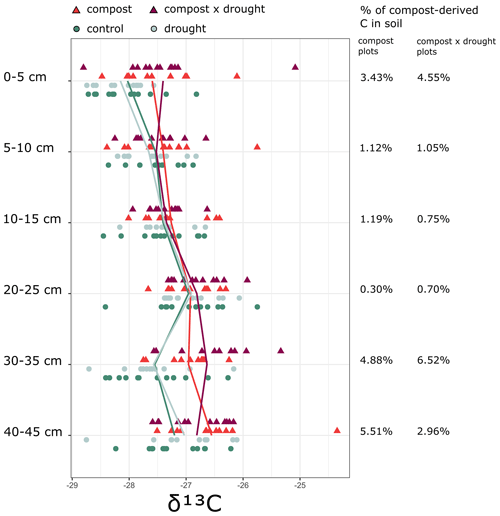
Figure 1Values of δ13C in the soil in compost-treated (triangles) and untreated (control, light green dots) plots under drought (dark red and dark blue) and ambient precipitation (orange and light green) conditions in 2022 at different depths. The percentage of compost-derived C in the soil was calculated with the isotope mixing model given in Eq. (2).
3.1 Compost and drought effects
Total soil C content (P = 0.04) and aboveground biomass (P = 0.04) increased in the compost-treated plots. The latter increased by 23 % (mean control plots = 642 g m2, SD = 129.23; mean compost plots = 788 g m2, SD = 221.7). The effect on soil C was significant only in the top 0–5 cm layer (Fig. 2), where the soil C content increased by 18 % (mean control plots C content = 29.9 mg g−1, SD = 1.03; mean compost plots = 35.3 mg g−1, SD = 0.75). Moreover, soil nitrogen (N) was higher in the top 0–5 cm layer in the compost-treated plots (mean control plots = 2.44 mg g−1, SD = 0.06; mean compost plots = 2.88 mg g−1, SD = 0.06; P<0.05), but the treatment did not significantly affect the C : N ratio. The compost treatment also decreased the bulk density by 9 % (P = 0.03) in the first 10 cm of soil (mean control plots = 1.34 g cm3, SD =0.18; mean compost plots = 1.22 g cm3, SD =0.17), but it did not affect any other variable. The increase in the soil C content under compost addition in the topsoil was offset by the reduced bulk density, so that there was no statistically significant change in the soil C stocks. However, we note that mean soil C stocks in the compost-treated (ambient precipitation) plots were 6 % higher in the first 15 cm, although this increase was not statistically significant. This increase is slightly higher than the percentage of compost-derived C found in that layer (mean control plots = 4.02 kg m2, SD =0.92; mean compost plots = 4.26 kg m2, SD =0.59).
Experimental drought had no significant overall effect on aboveground biomass. Although biomass decreased by nearly 4 % under the rainout shelters (mean control plots = 642 g m2, SD = 129.23; mean drought plots = 617 g m2, SD = 180.25), this reduction was only statistically significant in the compost-treated plots (P = 0.02). Furthermore, there was no significant difference in plant biomass between the drought-treated plots with and without compost addition.
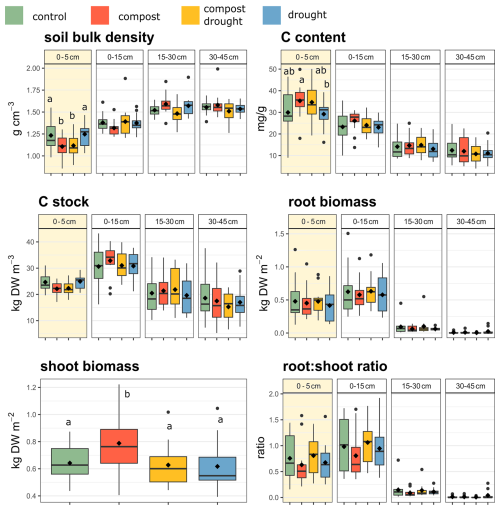
Figure 2Soil bulk density, soil carbon (C) content, soil C stock, root biomass, shoot biomass, and root : shoot ratio values at different sampling depths in 2022 (n = 12). Values are averages of all sites. The color code used in the figure is as follows: green – control; red – compost; yellow – compost × drought; blue – drought. Boxes show the mean (diamond inside the box), median (horizontal line), and interquantile range (IQR, colored box); whiskers extend to 1.5 × IQR; and dots in the graph are outliers. Different letters indicate statistically significant differences between means (P < 0.05). The yellow squares indicate the top layer (0–5 cm).
3.2 Root traits
In all drought-treated plots, we observed an increase in the root tissue density (P = 0.048), the specific root length of fine roots (P = 0.049), and the average root diameter (P = 0.045). If only roots in the top layer (0–5 cm) are considered, in addition to these patterns, the specific root length of coarse roots decreased under drought conditions (P = 0.04). In contrast, after compost addition, the root tissue density (P = 0.02) and specific root length of all roots increased (P = 0.01).
In all control plots, the soil C and root biomass were positively correlated in both the top 5–15 cm layer (5–10 cm, r = 0.42, P = 0.04; 10–15 cm, r=0.5, P = 0.01) and the whole 0–30 cm layer (0–30 cm, r = 0.63, P < 0.01). The soil C content was also positively correlated with the root : shoot ratio (5–10 cm, r = 0.44, P = 0.03; 10–15 cm, r = 0.4, P=0.052; 0–30 cm, r = 0.43, P = 0.04). In the compost-treated plots, the only significant correlation was between soil C and root biomass when considering the whole 0–30 cm layer (0–30 cm, r = 0.55, P < 0.01). The strength of the correlation did not differ between control and compost-treated plots (r = 0.22, P < 0.01 in control and compost-treated plots).
3.3 Spatial variability at the landscape scale
The soil C content, total C stock, bulk density, root biomass, and root : shoot ratio showed significant (P < 0.05) differences between catenary positions and depths, with lower C stocks at low catenary positions in the top 15 cm of soil; moreover, the soil C content and bulk density also differed significantly between grasslands (Fig. 3, Table S8). Grassland identity and the interaction between grasslands and catenary positions were the only significant predictors of aboveground biomass (Fig. 3).
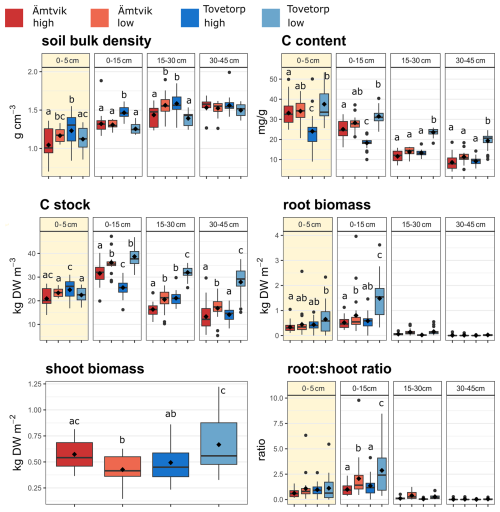
Figure 3Soil bulk density, soil carbon (C) content, soil C stock, root biomass, shoot biomass, and root : shoot ratio values at different sampling depths at the four sites, excluding treatments. The data consist of average values from 2019 (all plots, n = 48) and 2022 (only control plots, n = 12). The color code used in the figure is as follows: red – Ämtvik high catenary position; orange – Ämtvik low catenary position; blue – Tovetorp high catenary position; light blue – Tovetorp low catenary position. Boxes show the mean (diamond inside the box), median (horizontal line), and interquantile range (IQR, colored box); whiskers extend to 1.5 × IQR; and dots in the graph are outliers. Different letters indicate statistically significant differences between means (P < 0.05). The yellow squares indicate the top layer (0–5 cm).
3.4 Temporal changes during the 2019–2022 period
Aboveground biomass, root biomass, and soil C also differed significantly between sampling years (P < 0.05; Fig. 4, Table S9). The largest change was observed in aboveground biomass, which was 53 % higher in 2022 compared with 2019 (from 419.68 g m−2, SD = 137.45, to 642.23 g m−2, SD = 129.23). Conversely, the total soil C content and root biomass in the first 15 cm decreased by 21.5 % (from 29.7 mg g−1, SD = 0.73, to 23.3 mg g−1, SD = 0.71) and 38.7 % (from 1017.95 g m−2, SD = 955.16, to 623.65 g m−2, SD = 65.19), respectively.
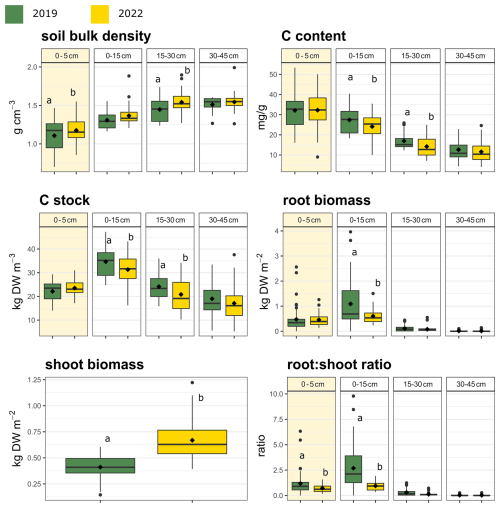
Figure 4Soil bulk density, soil carbon (C) content, soil C stock, root biomass, shoot biomass, and root : shoot ratio values at different sampling depths in 2019 and 2022 (control plots, n = 12). Values are means for all plots. The color code used in the figure is as follows: green – 2019; yellow – 2022. Boxes show the mean (diamond inside the box), median (horizontal line), and interquantile range (IQR, colored box); whiskers extend to 1.5 × IQR; and dots in the graph are outliers. Different letters indicate statistically significant differences between means (P < 0.05). The yellow squares indicate the top layer (0–5 cm).
4.1 Compost effects on soil C and plant growth
The total soil C content increased after compost application; however, because bulk density was also reduced, there was no significant increase in soil C stocks (partly confirming our first hypothesis), despite higher mean soil C stocks in the compost-treated plots in the first 15 cm of soil. This difference was lower than the C addition (∼ 0.54 kg C m−2) because of respiration. Compost is partly decomposed organic matter and, thus, more chemically recalcitrant than fresh grass residues. As a result, its effects on SOC accrual can be persistent over several years (Sarker et al., 2022), even after a single application (Ryals and Silver, 2013). Despite evidence that compost amendments can lead to SOC accumulation within 2 years of application (Gravuer et al., 2019), it is likely that the effect of our treatment on soil properties and soil C will persist beyond the 2022 sampling. This is also supported by the isotope tracing (Fig. 1), indicating that at least a fraction of the compost-derived C is still present in the soil after three growing seasons. The significant increase in aboveground biomass 3 years after compost application could partly be explained by the persistence of favorable plant growing conditions promoted by compost amendment, such as increased plant-available N in the soil. This mechanism was invoked by Oladeji et al. (2020) and may interact with precipitation-related interannual variability in plant growth (Sala et al., 2012). Our results suggest that compost treatments might benefit the ecosystem C balance indirectly through increased biomass production, while others have argued that compost can also extend the growing season (Fenster et al., 2023). These interactions between land management, vegetation growth, and plant-derived C inputs also underline the importance of including vegetation dynamics when assessing the effectiveness of C management. Our compost addition treatment did not lead to a significant increase in soil C stocks, but it did result in a lower net C loss from the grassland, mediated by an increase in plant biomass. In fact, there was a tendency toward higher SOC stocks (although this was not statistically significant), and the magnitude of such changes was higher than the amount of compost-derived C remaining in that layer. This suggests that the increase in soil C is not only stemmed from the amendment itself but also from increased plant C inputs. However, longer-term studies are necessary to (1) understand whether SOC saturation limits the effectiveness of compost amendments in sustaining these gains over time (Moinet et al., 2023) and (2) account for loss of C elsewhere, where compost is produced.
Compost enhanced aboveground biomass growth but not root growth, possibly in response to the increased nutrient supply (Bloom et al., 1985; Poorter and Nagel, 2000), thereby only partly confirming our first hypothesis. In broader terms, this suggests that the compost treatment led plants to preferentially allocate resources that would otherwise have be allocated to nutrient acquisition belowground to aboveground organs (Cleland et al., 2019). Nevertheless, enhanced root tissue density and increased specific root length in the top 0–5 cm layer suggest that the root response to organic amendments is manifested in more subtle changes in root traits related to nutrient acquisition (Bardgett et al., 2014), rather than in net root biomass production.
Microbial activity and microbial biomass can be higher after compost addition (Sarker et al., 2022; Gravuer et al., 2019). Here, the limited effects of the compost treatment on soil C stocks suggest that the potential C accrual introduced due to increased plant productivity might have been offset by increased microbial respiration (promoted by either compost or enhanced rhizodeposition of more productive plants) (Borken et al., 2002; Janzen, 2006). Finally, the significant spatiotemporal variability in both soil C and vegetation biomass observed in the control dataset suggests that treatment effects might be site-specific (Garbowski et al., 2020), and management plans seeking to increase C accrual should consider the potentially interactive effects of several biotic and abiotic factors, such as plant community composition, soil type, and climate. For instance, C stocks are typically higher at lower catenary positions (Johansson et al., 2023; Fig. 3), and the aboveground biomass increase in our experiment was highest at the site with the greatest abundance of grasses (Table S4).
4.2 Drought effects on soil moisture, soil C, and plant growth
Drought treatments reduced soil moisture and aboveground plant biomass, but they did not significantly decrease root biomass (Table S7), indicating preferential biomass allocation and resource investment to belowground organs under experimental drought conditions. Plant growth is very sensitive to yearly fluctuations and even the intra-annual distribution of precipitation (Knapp and Smith, 2001; Porporato et al., 2006). Because our analyses are based on only two temporal data points (2019 and 2022), it is difficult to assess whether drought reduced plant turnover, defined as the ratio of standing biomass to net primary productivity (NPP). As there was some natural variability in the annual precipitation (see Sect. 2), it is possible that a legacy effect of this variability may have affected plant growth (Sala et al., 2012), particularly aboveground (Fig. 4), as aboveground growth is more sensitive than root biomass to yearly fluctuations in water availability (Zhang et al., 2021). In particular, legacy effects of the 2018 drought could have hampered growth in 2019, as aboveground vegetation in the control plots increased by more than 50 % between 2019 and 2022. Conversely, the high summer precipitation in 2021 could have buffered the effects of the experimental drought in 2022, leading to overall weak drought effects (Sala et al., 2012).
The drought treatment had a relatively small impact on plant biomass and roots in particular (Fig. 2). Because we do not know which plant species the sampled roots belong to, we cannot make any conclusions related to the belowground drought responses of different plant functional groups (Zhang et al., 2017; Mackie et al., 2019; Zhong et al., 2019). However, we note that, although the magnitude of the drought did not differ between locations and soil physical properties were similar across sites, drought effects differed across locations (Fig. S4). Therefore, we can hypothesize that differences in the plant communities account for at least some of the spatial heterogeneity observed in our study, as was observed by Garbowski et al. (2020). Moreover, while drought effects on root biomass were marginal, the drought treatment did increase both the root tissue density and average root diameter. Climate is a strong predictor of root trait variation (Freschet et al., 2017), and higher root tissue density is correlated with resource-conservative acquisition strategies (Bardgett et al., 2014) and a longer root lifespan (Ryser, 1996), suggesting some degree of drought adaptation in our plant communities.
Adopting a standardized drought experimental design improves comparability, but partial rainout shelters will still allow for a substantial amount of precipitation to pass through, potentially raising soil moisture above the wilting point. Experimental droughts also fail to account for reduced air humidity, which may underestimate the negative responses of plant biomass to drought in field experiments (Kröel-Dulay et al., 2022), and for increased temperatures, which often occur in combination with natural droughts. These methodological limitations might explain why we observed minor drought effects on vegetation. To understand the ecosystem-level implications of drought, soil C changes need to be considered as well. Dry conditions likely decrease heterotrophic respiration, as microbial activity is inhibited due to both physiological mechanisms, such as osmoregulation diverting efforts from resource acquisition to survival, and physical mechanisms, like the slower transport of substrates in dry soils (as the water films around soil particles shrink and pore connectivity is lost) (Moyano et al., 2013; Schimel, 2018). However, heterotrophic respiration increases again after soil rewetting, leading to disproportionally large C emissions during the short post-rewetting period (Canarini et al., 2017; Barnard et al., 2020). Because the drought plots with added compost had a higher fraction of compost-labeled isotopes compared with the non-drought plots in the topsoil (Fig. 1), this would imply that any soil C emission pulses at rewetting were not sufficient to compensate for the possibly lowered microbial activity during the soil moisture dry-downs. As a result, in our experiment, drought had no effects on soil C contents and stocks, as per our second hypothesis, although it slightly reduced soil bulk density (in a pretreatment vs. post-treatment comparison; data not shown), possibly in relation to shrinkage in dry soil.
4.3 Interactive effects of compost and drought
While previous studies have indicated increased soil water retention after soil amendments (Franco-Andreu et al., 2017; Ali et al., 2017), compost-treated drought plots in our study did not have higher soil moisture than the untreated drought plots 3 years after compost application (Fig. S3), which leads us to reject our third hypothesis. As the negative effects of drought on aboveground biomass were weak, they were not visibly compensated for by compost addition. On the contrary, the experimental drought eliminated the biomass increase detected in the compost-treated plots under ambient rainfall conditions, overriding the positive effects of the increased C and N provided through the compost. This suggests that the vegetation response in our experiment does not only depend on nutrient addition and interannual variability in precipitation but also likely on plant physiological processes related to water availability (Bista et al., 2018) and on the ability of soil microbes to render the nutrients available for plant uptake (which also depends on soil water). Interestingly however, while both compost and drought tended to reduce root biomass, there was a tendency for a higher root : shoot ratio in the plots with combined compost and drought treatment (Fig. 2). While our results from the compost-treated plots show that plants may reduce their belowground biomass investment relative to aboveground growth when adding organic matter, this mechanism appeared to work differently under drought conditions, during which time plants may shift C allocation belowground to aid in water acquisition (Eziz et al., 2017; Guswa, 2010). This improved capacity for soil water absorption could offset any compost-induced increase in the soil water retention capacity. However, as our experiment did not include drought recovery, it is not known if this change would persist after the end of the experimental drought.
We explored how drought and compost amendment affect soil properties and above- and belowground plant biomass within a grassland ecosystem. Compost amendment and drought had distinct effects on plant shoot and root growth, revealing the presence of trade-offs in their responses to environmental change. The compost treatment led to an increase in biomass in shoots but not in roots and, ultimately, did not result in an increase in soil C stocks. Drought did not significantly affect plant biomass, but it led to changes in root traits and stunted the compost-induced increase in plant growth measured in plots under ambient precipitation conditions. These findings improve our understanding of C dynamics in grasslands by illustrating the different components of plant and soil properties affected by compost amendment. We also observed significant spatiotemporal variability in vegetation and soil C dynamics over the study period, which may be driven by differences in topography, land use, and plant community composition and by temporal variability in precipitation.
The data used in this paper can be accessed via the Bolin Centre Database: https://doi.org/10.17043/guasconi-2025-soil-properties-1 (Guasconi et al., 2025).
The supplement related to this article is available online at https://doi.org/10.5194/soil-11-233-2025-supplement.
SC, DG, GH, SM, and NR: conceptualization and methodology; DG and NR: field investigation and lab work; DG: statistical analysis; DG: writing – original draft; DG, SC, GH, SM, and NR: writing – review and editing.
The contact author has declared that none of the authors has any competing interests.
Publisher’s note: Copernicus Publications remains neutral with regard to jurisdictional claims made in the text, published maps, institutional affiliations, or any other geographical representation in this paper. While Copernicus Publications makes every effort to include appropriate place names, the final responsibility lies with the authors.
The authors wish to thank Tovetorp Research Station and the land owners of Ämtvik for allowing us to work on their grasslands; Linnea Ström, Louis Hunninck, Luca Durstevitz, Marie Förg, Olov Hammarström, Victor Eriksson, and Willeke A’Campo for help with sampling; and Lukas Rimondini, Torge Gerwin, and Sean Burke for help in the laboratory.
This research has been supported by the Bolin Centre for Climate Research and by the Carl Mannerfelt Foundation
The publication of this article was funded by the Swedish Research Council, Forte, Formas, and Vinnova.
This paper was edited by Luisella Celi Celi and reviewed by Benedikt Boppre and two anonymous referees.
Ahmad, A. A., Fares, A., Paramasivam, S., Elrashidi, M. A., and Savabi, R. M.: Biomass and nutrient concentration of sweet corn roots and shoots under organic amendments application, J. Environ. Sci. Heal. B, 44, 742–754, https://doi.org/10.1080/03601230903163921, 2009.
Ali, S., Rizwan, M., Qayyum, M. F., Ok, Y. S., Ibrahim, M., Riaz, M., Arif, M. S., Hafeez, F., Al-Wabel, M. I., and Shahzad, A. N.: Biochar soil amendment on alleviation of drought and salt stress in plants: a critical review, Environ. Sci. Pollut. Res., 24, 12700–12712, https://doi.org/10.1007/s11356-017-8904-x, 2017.
Bai, Y. and Cotrufo, M. F.: Grassland soil carbon sequestration: Current understanding, challenges, and solutions, Science, 377, 603–608, https://doi.org/10.1126/science.abo2380, 2022.
Bardgett, R. D., Mommer, L., and De Vries, F. T.: Going underground: root traits as drivers of ecosystem processes, Trend. Ecol. Evol., 29, 692–699, https://doi.org/10.1016/j.tree.2014.10.006, 2014.
Barnard, R. L., Blazewicz, S. J., and Firestone, M. K.: Rewetting of soil: Revisiting the origin of soil CO2 emissions, Soil Biol. Biochem., 147, 107819, https://doi.org/10.1016/j.soilbio.2020.107819, 2020.
Beniston, J. W., DuPont, S. T., Glover, J. D., Lal, R., and Dungait, J. A. J.: Soil organic carbon dynamics 75 years after land-use change in perennial grassland and annual wheat agricultural systems, Biogeochemistry 120, 37–49, https://doi.org/10.1007/s10533-014-9980-3, 2014.
Bista, D. R., Heckathorn, S. A., Jayawardena, D. M., Mishra, S., and Boldt, J. K.: Effects of Drought on Nutrient Uptake and the Levels of Nutrient-Uptake Proteins in Roots of Drought-Sensitive and -Tolerant Grasses, Plants, 7, 28, https://doi.org/10.3390/plants7020028, 2018.
Bloom, A. J., Chapin, F. S., and Mooney, H. A.: Resource limitation in plants – an economic analogy, Annu. Rev. Ecol. Syst., 16, 363–392, https://doi.org/10.1146/annurev.es.16.110185.002051, 1985.
Borken, W., Muhs, A., and Beese, F.: Application of compost in spruce forests: effects on soil respiration, basal respiration and microbial biomass, Ecol. Manag., 159, 49–58, https://doi.org/10.1016/S0378-1127(01)00709-5, 2002.
Brown, S. and Cotton, M.: Changes in Soil Properties and Carbon Content Following Compost Application: Results of On-farm Sampling, Compost Sci. Util., 19, 87–96, https://doi.org/10.1080/1065657X.2011.10736983, 2011.
Canarini, A., Kiær, L. P., and Dijkstra, F. A.: Soil carbon loss regulated by drought intensity and available substrate: A meta-analysis, Soil Biol. Biochem., 112, 90–99, https://doi.org/10.1016/j.soilbio.2017.04.020, 2017.
Cleland, E., Lind, E., DeCrappeo, N., DeLorenze, E., Wilkins, R., Adler, P., Bakker, J. D., Brown, C. S., Davies, K. F., Esch, E., Firn, J., Gressard, S., Gruner, D. S., Hagenah, N., Harpole, W. S., Hautier, Y., Hobbie, S. E., Hofmockel, K. S., Kirkman, K., Knops, J., Kopp, C. W., La Pierre, K. J., MacDougall, A., McCulley, R. L., Melbourne, B. A., Moore, J. L., Prober, S. M., Riggs, C., Risch, A. C., Schuetz, M., Stevens, C., Wragg, P. D., Wright, J., Borer E. T., and Seabloom, E. W.: Belowground Biomass Response to Nutrient Enrichment Depends on Light Limitation Across Globally Distributed Grasslands, Ecosystems, 22, 1466–1477, https://doi.org/10.1007/s10021-019-00350-4, 2019.
Conant, R. T., Paustian, K., and Elliott, E. T.: Grassland management and conversion into grassland: effects on soil carbon, Ecol. Appl., 11, 343–355, https://doi.org/10.1890/1051-0761(2001)011[0343:GMACIG]2.0.CO;2, 2001
Cotrufo, M., Soong, J., Horton, A. J., Campbell, E. E., Haddix, M. L., Wall, D. H., and Parton, W. J.: Formation of soil organic matter via biochemical and physical pathways of litter mass loss, Nat. Geosci., 8, 776–779, https://doi.org/10.1038/ngeo2520, 2015.
DeLonge, M. S., Ryals, R., and Silver, W. L.: A Lifecycle Model to Evaluate Carbon Sequestration Potential and Greenhouse Gas Dynamics of Managed Grasslands, Ecosystems, 16, 962–979, https://doi.org/10.1007/s10021-013-9660-5, 2013.
Deng, L., Peng, C., Kim, D.-G., Li, J., Liu, Y., Hai, X., Liu, Q., Huang, C., Shangguan, Z., and Kuzyakov, Y.: Drought effects on soil carbon and nitrogen dynamics in global natural ecosystems, Earth-Sci. Rev., 214, 103501, https://doi.org/10.1016/j.earscirev.2020.103501, 2021.
Don, A., Seidel, F., Leifeld, J., Kätterer, T., Martin, M., Pellerin, S., Emde, D., Seitz, D., and Chenu, C.: Carbon sequestration in soils and climate change mitigation – Definitions and pitfalls, Glob. Change Biol., 30, e16983, https://doi.org/10.1111/gcb.16983, 2024.
European Commission: Directorate-General for Health and Food Safety, From farm to fork: our food, our health, our planet, our future: the European Green Deal, Publications Office of the European Union, https://data.europa.eu/doi/10.2875/653604 (last access: 16 January 2025), 2020.
Eziz, A., Yan, Z., Tian, D., Han, W., Tang, Z., and Fang, J.: Drought effect on plant biomass allocation: a meta-analysis, Ecol. Evol., 7, 11002–11010, https://doi.org/10.1002/ece3.3630, 2017.
Fenster, T. L. D., Torres, I., Zeilinger, A., Chu, H., and Oikawa, P.: Compost amendment to a grazed California annual grassland increases gross primary productivity due to a longer growing season, J. Geophys. Res.-Biogeo., 128, e2023JG007621, https://doi.org/10.1029/2023JG007621, 2023.
Fischer, B. M. C., Manzoni, S., Morillas, L., Garcia, M., Johnson, M., and Lyon, S. W.: Improving agricultural water use efficiency with biochar – A synthesis of biochar effects on water storage and fluxes across scales, Sci. Total Environ., 657, 853–862, https://doi.org/10.1016/j.scitotenv.2018.11.312, 2019.
Franco-Andreu, L., Gomez, I., Parrado, J., Garcia, C., Hernandez, T., and Tejada, M.: Soil biology changes as a consequence of organic amendments subjected to a severe drought, Land Degrad. Dev., 28, 897–905, https://doi.org/10.1002/ldr.2663, 2017.
Freschet, G. T., Valverde-Barrantes, O. J., Tucker, C. M., Craine, J. M., McCormack, M. L., Violle, C., Fort, F., Blackwood, C. B., Urban-Mead, K. R., Iversen, C. M., Bonis, A., Comas, L. H., Cornelissen, J. H. C., Dong, M., Guo, D., Hobbie, S. E., Holdaway, R. J., Kembel, S. W., Makita, N., Onipchenko, V. G., Picon-Cochard, C., Reich, P. B., Riva, E. G., Smith, S. W., Soudzilovskaia, N. A., Tjoelker, M. G., Wardle, D. A., and Roumet, C.: Climate, soil and plant functional types as drivers of global fine-root trait variation, J. Ecol., 105, 1182–1196, https://doi.org/10.1111/1365-2745.12769, 2017.
Garbowski, T., Bar-Michalczyk, D., Charazińska, S., Grabowska-Polanowska, B., Kowalczyk, A., and Lochyński, P.: An overview of natural soil amendments in agriculture, Soil Till. Res., 225, 105462, https://doi.org/10.1016/j.still.2022.105462, 2023.
Garbowski, M., Brown, C. S., and Johnston, D. B.: Soil Amendment Interacts with Invasive Grass and Drought to Uniquely Influence Aboveground versus Belowground Biomass in Aridland Restoration, Restor. Ecol., 28, A13–A23, 2020.
Gaudinski, J. B., Trumbore, S. E., Davidson, E. A., and Zheng, S.: Soil carbon cycling in a temperate forest: radiocarbon-based estimates of residence times, sequestration rates and partitioning of fluxes, Biogeochemistry, 51, 33–69, https://doi.org/10.1023/A:1006301010014, 2000.
Gebhardt, M., Fehmi, J. S., Rasmussen, C., and Gallery, R. E.: Soil amendments alter plant biomass and soil microbial activity in a semi-desert grassland, Plant Soil, 419, 53–70, https://doi.org/10.1007/s11104-017-3327-5, 2017.
Gravuer, K., Gennet, S., and Throop, H. L.: Organic amendment additions to rangelands: A meta-analysis of multiple ecosystem outcomes, Glob. Change Biol., 25, 1152–1170, https://doi.org/10.1111/gcb.14535, 2019.
Guasconi, D., Manzoni, S., and Hugelius, G.: Climate-dependent responses of root and shoot biomass to drought duration and intensity in grasslands – a meta-analysis, Sci. Total Environ., 903, 166209, https://doi.org/10.1016/j.scitotenv.2023.166209, 2023.
Guasconi, D., Roth, N., Cousins, S. A. O., Manzoni, S., and Hugelius, G.: Soil physical, chemical and biological properties in two Swedish grasslands between 2019 and 2022, Dataset version 1, Bolin Centre Database [data set], https://doi.org/10.17043/guasconi-2025-soil-properties-1, 2025.
Guo, T., Weise, H., Fiedler, S., Lohmann, D., and Tietjen, B.: The role of landscape heterogeneity in regulating plant functional diversity under different precipitation and grazing regimes in semi-arid savannas, Ecol. Model., 379, 1–9, https://doi.org/10.1016/j.ecolmodel.2018.04.009, 2018.
Guswa, A. J.: Effect of plant uptake strategy on the water-optimal root depth: effect of plant uptake strategy on root, Water Resour. Res., 46, W09601, https://doi.org/10.1029/2010WR009122, 2010.
Haque, A. N. A., Uddin, M. K., Sulaiman, M. F., Amin, A. M., Hossain, M., Zaibon, S., and Mosharrof, M.: Assessing the Increase in Soil Moisture Storage Capacity and Nutrient Enhancement of Different Organic Amendments in Paddy Soil, Agriculture, 11, 44, https://doi.org/10.3390/agriculture11010044, 2021.
Hasibeder, R., Fuchslueger, L., Richter, A., and Bahn, M.: Summer drought alters carbon allocation to roots and root respiration in mountain grassland, New Phytol., 205, 1117–1127, https://doi.org/10.1111/nph.13146, 2015.
Hayes, M. A., Jesse, A., Basam, T., Reef, R., Keuskamp, J. A., and Lovelock, C. E.: The contrasting effects of nutrient enrichment on growth, biomass allocation and decomposition of plant tissue in coastal wetlands, Plant Soil, 416, 193–204, 2017.
Heimann, M. and Reichstein, M.: Terrestrial ecosystem carbon dynamics and climate feedbacks, Nature, 451, 289–292, https://doi.org/10.1038/nature06591, 2008.
Hirte, J., Walder, F., Hess, J., Büchi, L., Colombi, T., van der Heijden, M. G., and Mayer, J.: Enhanced root carbon allocation through organic farming is restricted to topsoils, Sci. Total Environ., 755, 143551, https://doi.org/10.1016/j.scitotenv.2020.143551, 2021.
IPCC: Intergovernmental Panel on Climate Change: Climate Change 2021 – The Physical Science Basis: Working Group I Contribution to the Sixth Assessment Report of the Intergovernmental Panel on Climate Change, Cambridge University Press, 2023.
Jackson, R. B., Lajtha, K., Crow, S. E., Hugelius, G., Kramer, M. G., and Piñeiro, G.: The ecology of soil carbon: pools, vulnerabilities, and biotic and abiotic controls, Annu. Rev. Ecol. Evol. Syst., 48, 419–445, https://doi.org/10.1146/annurev-ecolsys-112414-054234, 2017.
Janzen, H. H.: The soil carbon dilemma: Shall we hoard it or use it?, Soil Biol. Biochem., 38, 419–424, https://doi.org/10.1016/j.soilbio.2005.10.008, 2006.
Johansson, A., Livsey, J., Guasconi, D., Hugelius, G., Lindborg, R., and Manzoni, S.: Long-term soil organic carbon changes after cropland conversion to grazed grassland in Southern Sweden, Soil Use Manag., 40, e13004, https://doi.org/10.1111/sum.13004, 2023.
Kallenbach, C. M., Conant, R. T., Calderón, F., and Wallenstein, M. D.: A novel soil amendment for enhancing soil moisture retention and soil carbon in drought-prone soils, Geoderma, 337, 256–265, https://doi.org/10.1016/j.geoderma.2018.09.027, 2019.
Keesstra, S. D., Bouma, J., Wallinga, J., Tittonell, P., Smith, P., Cerdà, A., Montanarella, L., Quinton, J. N., Pachepsky, Y., van der Putten, W. H., Bardgett, R. D., Moolenaar, S., Mol, G., Jansen, B., and Fresco, L. O.: The significance of soils and soil science towards realization of the United Nations Sustainable Development Goals, SOIL, 2, 111–128, https://doi.org/10.5194/soil-2-111-2016, 2016.
Knapp, A. K. and Smith, M. D.: Variation among biomes in temporal dynamics of aboveground primary production, Science, 291, 481–484, https://doi.org/10.1126/science.291.5503.481, 2001.
Knapp, A. K., Avolio, M. L., Beier, C., Carroll, C. J. W., Collins, S. L., Dukes, J. S., Fraser, L. H., Griffin‐Nolan, R. J., Hoover, D. L., Jentsch, A., Loik, M. E., Phillips, R. P., Post, A. K., Sala, O. E., Slette, I. J., Yahdjian, L., and Smith, M. D.: Pushing precipitation to the extremes in distributed experiments: Recommendations for simulating wet and dry years, Glob. Change Biol., 23, 1774–1782, https://doi.org/10.1111/gcb.13504, 2017.
Kröel-Dulay, G., Mojzes, A., Szitár, K., Bhan, M., Batáry, P., Beier, C., Bilton, M., De Boeck, H. J., Dukes, J. S., Estiarte, M., Holub, P., Jentsch, A., Kappel Schmidt, I., Kreyling, J., Reinsch, S., Steenberg Larsen, K., Sternberg, M., Tielbörger, K., Tietema, A., Vicca, S., and Peñuelas, J.: Field experiments underestimate aboveground biomass response to drought, Nat. Ecol. Evol., 6, 540–545, https://doi.org/10.1038/s41559-022-01685-3, 2022.
Liu, J., Ma, X., Duan, Z., Jiang, J., Reichstein, M., and Jung, M.: Impact of temporal precipitation variability on ecosystem productivity, Wiley Interdisciplinary Review Water, 7, 1–22, https://doi.org/10.1002/wat2.1481, 2020.
Luo, G., Li, L., Friman, V.-P., Guo, J., Guo, S., Shen, Q., and Ling, N.: Organic amendments increase crop yields by improving microbe-mediated soil functioning of agroecosystems: A meta-analysis, Soil Biol. Biochem., 124, 105–115, https://doi.org/10.1016/j.soilbio.2018.06.002, 2018.
Mackie, K. A., Zeiter, M., Bloor, J. M. G., and Stampfli, A.: Plant functional groups mediate drought resistance and recovery in a multisite grassland experiment, Edited by Franciska de Vries, J. Ecol., 107, 937–949, https://doi.org/10.1111/1365-2745.13102, 2019.
Malik, M. A., Khan, K. S., and Marschner, P.: Fayyaz-ul-Hassan: Microbial biomass, nutrient availability and nutrient uptake by wheat in two soils with organic amendments, J. Soil Sci. Plant Nut., 13, 955–66, https://doi.org/10.4067/s0718-95162013005000075, 2013.
Minasny, B., Malone, B. P., McBratney, A. B., Angers, D. A., Arrouays, D., Chambers, A., Chaplot, V., Chen, Z.-S., Cheng, K., Das, B. S., Field, D. J., Gimona, A., Hedley, C. B., Hong, S. Y., Mandal, B., Marchant, B. P., Martin, M., McConkey, B. G., Mulder, V. L., O'Rourke, S., Richer-de-Forges, A. C., Odeh, I., Padarian, J., Paustian, K., Pan, G., Poggio, L., Savin, I., Stolbovoy, V., Stockmann, U., Sulaeman, Y., Tsui, C.-C., Vågen, T.-G., van Wesemael, B., and Winowiecki, L.: Soil carbon 4 per mille, Geoderma, 292, 59–86, https://doi.org/10.1016/j.geoderma.2017.01.002, 2017.
Moinet, G. Y. K., Hijbeek, R., van Vuuren, D. P., and Giller, K. E.: Carbon for soils, not soils for carbon, Glob. Change Biol., 29, 2384–2398, https://doi.org/10.1111/gcb.16570, 2023.
Moyano, F. E., Manzoni, S., and Chenu, C.: Responses of soil heterotrophic respiration to moisture availability: An exploration of processes and models, Soil Biol. Biochem., 59, 72–85, https://doi.org/10.1016/j.soilbio.2013.01.002, 2013.
Oladeji, O., Tian, G., Lindo, P., Kumar, K., Cox, A., Hundal, L., Zhang, H., and Podczerwinski, E.: Nitrogen release and plant available nitrogen of composted and un-composted biosolids, Water Environ. Res., 92, 631–640, https://doi.org/10.1002/wer.1260, 2020.
Paltineanu, C., Dumitru, S., Vizitiu, O., Mocanu, V., Lăcătusu, A.-R., Ion, S., and Domnariu, H.: Soil organic carbon and total nitrogen stocks related to land use and basic environmental properties – assessment of soil carbon sequestration potential in different ecosystems, Catena, 246, 108435, https://doi.org/10.1016/j.catena.2024.108435, 2024.
Paul, C., Bartkowski, B., Dönmez, C., Don, A., Mayer, S., Steffens, M., Weigl, S., Wiesmeier, M., Wolf, A., and Helming, K.: Carbon farming: Are soil carbon certificates a suitable tool for climate change mitigation?, J. Environ. Manag., 330, 117142, https://doi.org/10.1016/j.jenvman.2022.117142, 2023.
Poeplau, C., Begill, N., Liang, Z., and Schiedung, M.: Root litter quality drives the dynamic of native mineral-associated organic carbon in a temperate agricultural soil, Plant Soil, 491, 439–456, https://doi.org/10.1007/s11104-023-06127-y, 2023.
Poorter, H. and Nagel, O.: The role of biomass allocation in the growth response of plants to different levels of light, CO2, nutrients and water: a quantitative review, Funct. Plant Biol., 27, 1191, https://doi.org/10.1071/PP99173_CO, 2000.
Porporato, A., Vico, G., and Fay, P. A.: Superstatistics of hydro-climatic fluctuations and interannual ecosystem productivity, Geophys. Res. Lett., 33, L15402, https://doi.org/10.1029/2006GL026412, 2006.
R Core Team: R: A language and environment for statistical computing, R Foundation for Statistical Computing, Vienna, Austria, https://www.R-project.org/ (last access: 16 January 2025), 2022.
Rasse, D. P., Rumpel, C., and Dignac, M. F.: Is soil carbon mostly root carbon? Mechanisms for a specific stabilization, Plant Soil, 269, 341–356, https://doi.org/10.1007/s11104-004-0907-y, 2005.
Rawls, W. J., Pachepsky, Y. A., Ritchie, J. C., Sobecki, T. M., and Bloodworth, H.: Effect of soil organic carbon on soil water retention, Geoderma, 116, 61–76, https://doi.org/10.1016/S0016-7061(03)00094-6, 2003.
Roth, N.: Grasslands in a changing climate – Summer drought and winter warming effects on grassland vegetation, Doctoral thesis, Stockholm University, ISBN: 978-91-8014-558-9, 2023.
Roth, N., Kimberley, A., Guasconi, D., Hugelius, G., and Cousins, S. A. O.: Floral resources in Swedish grasslands remain relatively stable under an experimental drought and are enhanced by soil amendments if regularly mown, Ecol. Solut. Evid., 4, e12231, https://doi.org/10.1002/2688-8319.12231, 2023.
Ryals, R. and Silver, W. L.: Effects of organic matter amendments on net primary productivity and greenhouse gas emissions in annual grasslands, Ecol. Appl., 23, 46–59, https://doi.org/10.1890/12-0620.1, 2013.
Ryals, R., Hartman, M. D., Parton, W. J., DeLonge, M. S., and Silver, W. L.: Long-term climate change mitigation potential with organic matter management on grasslands, Ecol. Appl., 25, 531–545, https://doi.org/10.1890/13-2126.1, 2015.
Ryals, R., Eviner, V. T., Stein, C., Suding, K. N., and Silver, W. L.: Grassland compost amendments increase plant production without changing plant communities, Ecosphere, 7, e01270, https://doi.org/10.1002/ecs2.1270, 2016.
Ryser, P.: The importance of tissue density for growth and life span of leaves and roots: a comparison of five ecologically contrasting grasses, Funct. Ecol., 10, 717–723, https://doi.org/10.2307/2390506, 1996.
Sala, O. E., Gherardi, L. A., Reichmann, L., Jobbágy, E., and Peters, D.: Legacies of precipitation fluctuations on primary production: theory and data synthesis, Philos. T. R. Soc. B, 367, 3135–3144, https://doi.org/10.1098/rstb.2011.0347, 2012.
Sanderman, J., Hengl, T., and Fiske, G. J.: Soil Carbon Debt of 12,000 Years of Human Land Use, P. Natl. Acad. Sci. USA, 114, 9575–9580, 2017.
Sarker, T. C., Zotti, M., Fang, Y., Giannino, F., Mazzoleni, S., Bonanomi, G., Cai, Y., and Chang, S. X.: Soil Aggregation in Relation to Organic Amendment: a Synthesis, J. Soil Sci. Plant Nutr., 22, 2481–2502, https://doi.org/10.1007/s42729-022-00822-y, 2022.
Schimel, J. P.: Life in Dry Soils: Effects of Drought on Soil Microbial Communities and Processes, in: Annual Review of Ecology, Evolution, and Systematics, edited by: Futuyma, D. J., Annu. Rev. Ecol. Evol. S., 49, 409–432, https://doi.org/10.1146/annurev-ecolsys-110617-062614, 2018.
Sharma, S., Singh, P., Chauhan, S., and Choudhary, O. P.: Landscape position and slope aspects impacts on soil organic carbon pool and biological indicators of a fragile ecosystem in high-altitude cold arid region, J. Soil Sci. Plant Nutr., 22, 2612–2632, https://doi.org/10.1007/s42729-022-00831-x, 2022.
Shi, Z., Allison, S. D., He, Y., Levine, P. A., Hoyt, A. M., Beem-Miller, J., Zhu, Q., Wieder, W. R., Trumbore, S., and Randerson, J.: The age distribution of global soil carbon inferred from radiocarbon measurements, Nat. Geosci., 13, 555–559, https://doi.org/10.1038/s41561-020-0596-z, 2020.
Swedish Meteorological and Hydrological Institute: Normal årsmedeltemperatur, https://www.smhi.se/data/meteorologi/kartor/normal/arsmedeltemperatur-normal (last access: 16 January 2025), 2022.
Wang, Y., Shao, M., Sun, H., Fu, Z., Fan, J., Hu, W., and Fang, L.: Response of deep soil drought to precipitation, land use and topography across a semiarid watershed, Agr. Forest Meteorol., 282/283, 107866, https://doi.org/10.1016/j.agrformet.2019.107866, 2020.
Yahdjian, L. and Sala, O. E.: A rainout shelter design for intercepting different amounts of rainfall, Oecologia, 133, 95–101, https://doi.org/10.1007/s00442-002-1024-3, 2002.
Yang, F., Zhang, G.-L., Yang, J.-L., Li, D.-C., Zhao, Y.-G., Liu, F., Yang, R.-M., and Yang, F.: Organic matter controls of oil water retention in an alpine grassland and its significance for hydrological processes, J. Hydrol., 519, 3086–3093, https://doi.org/10.1016/j.jhydrol.2014.10.054, 2014.
Zhang, C. and Xi, N.: Precipitation Changes Regulate Plant and Soil Microbial Biomass Via Plasticity in Plant Biomass Allocation in Grasslands: A Meta-Analysis, Front. Plant Sci., 12, 614968, https://doi.org/10.3389/fpls.2021.614968, 2021.
Zhang, F., Quan, Q., Song, B., Sun, J., Chen, Y., Zhou, Q., and Niu, S.: Net primary productivity and its partitioning in response to precipitation gradient in an alpine meadow, Sci. Rep., 7, 15193, https://doi.org/10.1038/s41598-017-15580-6, 2017.
Zhong, M., Song, J., Zhou, Z., Ru, J., Zheng, M., Li, Y., Hui, D., and Wan, S.: Asymmetric responses of plant community structure and composition to precipitation variabilities in a semi-arid steppe, Oecologia, 191, 697–708, https://doi.org/10.1007/s00442-019-04520-y, 2019.