the Creative Commons Attribution 4.0 License.
the Creative Commons Attribution 4.0 License.
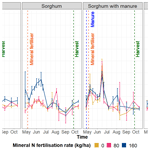
Interactions of fertilisation and crop productivity in soil nitrogen cycle microbiome and gas emissions
Laura Kuusemets
Ülo Mander
Jordi Escuer-Gatius
Alar Astover
Karin Kauer
Kaido Soosaar
Mikk Espenberg
Fertilised soils are a significant source of nitrous oxide (N2O), a highly active greenhouse gas and a stratospheric ozone depleter. Nitrogen (N) fertilisers, while boosting crop yield, also lead to N2O emissions into the atmosphere, impacting global warming. We investigated relationships between mineral N fertilisation rates and additional manure amendment with different crop types through the analysis of abundances of N cycle functional genes, soil N2O and N2 emissions, nitrogen use efficiency (NUE), soil physicochemical analysis and biomass production. Our study indicates that N2O emissions are predominantly dependent on the mineral N fertilisation rate and enhance with an increased mineral N fertilisation rate. Crop type also has a significant impact on soil N2O emissions. Higher N2O emissions were attained with the application of manure in comparison to mineral fertilisation. Manure amendment also increased the number of N cycle genes that are significant in the variations of N2O. The study indicates that N2O emissions were mainly related to nitrification in the soil. Quantification of nitrogen cycle functional genes also showed the potential role of denitrification, comammox (complete ammonia oxidation) and dissimilatory nitrate reduction to ammonium (DNRA) processes as a source of N2O. Our study did not find soil moisture to be significantly linked to N2O emissions. The results of the study provide evidence that, for wheat, a fertilisation rate of 80 kg N ha−1 is closest to the optimal rate for balancing biomass yield and N2O emissions and achieving a high NUE. Sorghum showed good potential for cultivation in temperate climates, as it showed a similar biomass yield compared to the other crop types and fertilisation rates but maintained low N2O emissions and N losses in a mineral N fertilisation rate of 80 kg N ha−1.
- Article
(1537 KB) - Full-text XML
-
Supplement
(1161 KB) - BibTeX
- EndNote
The rising demand for agricultural commodities and the management of agroecosystems are important factors contributing to global environmental problems. Increasing crop yield while reducing pollution from agricultural production is crucial (Abdalla et al., 2019; Tilman et al., 2011). Global food demand projections suggest a 50 % increase in agricultural production by 2050 (compared to 2012) to feed the rapidly growing human population (FAO, 2017). Enhancing agricultural production involves actions such as expanding agricultural land, applying more fertilisers and using water resources and fertilisers more effectively (Tian et al., 2021). In today's agricultural practices, the applied N with fertilisation is often excessive for plant needs (Robertson and Vitousek, 2009; Zhou et al., 2016). About half of the N applied to the fields is not taken up by crops (Coskun et al., 2017), which may lead to N loss in the surrounding environment. The main soil N loss mechanisms include denitrification, ammonia oxidation, N leaching, erosion of soil and ammonia (NH3) volatilisation (Thomson et al., 2012). This results in adverse ecological impacts, such as eutrophication of aquatic ecosystems and increased gaseous emissions of N into the atmosphere (Cameron et al., 2013; Liu et al., 2017; Whetton et al., 2022).
Fertilised soils are a significant source of nitrous oxide (N2O), contributing to the greenhouse effect and ozone depletion (Ravishankara et al., 2009; Shcherbak et al., 2014). N2O has 273 times higher global warming potential than carbon dioxide (CO2) over a 100-year timescale (IPCC, 2021). Even without adding N fertiliser in the current season or year, background N2O emissions (BNEs) may still occur. BNEs are caused by different N sources, including residual N in the soil from previous years' N application, deposition from the atmosphere, biological N2 fixation and mineralised N from plant residues (Gu et al., 2007; Kim et al., 2013; Abdalla et al., 2022).
The key microbial processes leading to soil N loss are nitrification and denitrification (Thomson et al., 2012). In agriculture, N fertilisers added to soil can be lost due to these processes (Saud et al., 2022). Nitrification was traditionally viewed as a two-step process carried out by separate functional groups of microorganisms oxidising ammonium (NH) sequentially to nitrite (NO) and nitrate (NO) under aerobic conditions (Kuypers et al., 2018; Koch et al., 2019; Nardi et al., 2020). However, in 2015, a significant advancement in our understanding of nitrification occurred with the discovery that a single microorganism, through the comammox (complete ammonia oxidation) process, can perform both nitrification steps (Daims et al., 2015; Van Kessel et al., 2015). Nitrification can reduce N availability for plant uptake by up to 50 %, primarily due to NO leaching and N2O emissions (Beeckman et al., 2018). Synthetic fertilisers containing NH3 offer an immediate substrate for ammonia oxidisers, thus accelerating the nitrification process (Ayiti and Babalola, 2022). Also, fertilisers that raise soil pH can significantly enhance the nitrification rate, as increasing the soil pH from 4.8 to 6.7 can boost nitrification rates by 30 times (DeForest and Otuya, 2020).
Denitrification is a microbially catalysed process under oxygen-limited conditions that is responsible for transforming NO sequentially into gaseous forms of N: nitric oxide, N2O and atmospheric N (Philippot et al., 2007; Zaman et al., 2012). The input of N fertilisers affects the soil's mineral N pool by providing larger amounts of available N for nitrification and denitrification processes, contributing to N2O emissions (Engel et al., 2010). Dissimilatory nitrate reduction to ammonium (DNRA) supplies NH to the soil, conserves bioavailable N and prevents the leaching of NO (Bai et al., 2020; Pandey et al., 2020). DNRA competes with denitrification in NO-reducing processes (Putz et al., 2018). Similarly to the denitrification and nitrification processes, DNRA can also be a source of N2O, although the quantities are modest (Rütting et al., 2011; Stremińska et al., 2012; Zaman et al., 2012). The carbon-to-nitrogen ratio () and are recognised as the main environmental factors controlling which nitrate-reducing process is favoured for DNRA, and denitrifying microbes compete for NO and carbon sources (Bai et al., 2020). DNRA is dominant in the presence of a high ratio and low NO availability, while the denitrification process favours low ratios of and (Bai et al., 2020; Pandey et al., 2020). These processes are mediated by different functional marker genes, including archaeal, bacterial and comammox amoA genes for nitrification, nrfA genes for DNRA and nosZ clades I and II and nirK and nirS genes for denitrification (Zaman et al., 2012; Hu et al., 2015; Zhang et al., 2021).
C3 photosynthesis, a dominant pathway among plants and found in wheat and barley, uses the Calvin–Benson pathway, while an alternative, the Hatch–Slack pathway, is used by C4 plants like sorghum and maize (Hibberd and Quick, 2002; Ehleringer and Cerling, 2002; Ehleringer, 1979; Ledvinka et al., 2022). In C3 plants, water loss through transpiration during CO2 uptake is a risk under hot and water-limited conditions (Joshi et al., 2022; Stevens et al., 2022). However, C4 plants, with a higher water use efficiency and a greater tolerance of hot and dry environments, make the cultivation of sorghum and other drought-tolerant plants likely to expand in regions affected by droughts (Anderson et al., 2020). Due to climate change, sorghum, as a resilient plant, is considered a novel crop for temperate Europe (Schaffasz et al., 2019). Only a limited number of studies have compared N2O emissions between different crop species. Abdalla et al. (2022) found that crop type has a significant effect (p<0.05) on the BNE values of soil. Furthermore, Bouwman et al. (2002) found that crop type has a significant influence on N2O emissions. However, a study including 372 sites showed that cover crops did not have a significant (p>0.05) effect on direct N2O emissions (Abdalla et al., 2019).
Previous studies on long-term fertilisation experiments have mostly focused on fertilisation's yield effects and changes in soil organic matter (Cvetkov and Tajnšek, 2009; Hijbeek et al., 2017; Káš et al., 2010; Spiegel et al., 2010; Tajnšek et al., 2013). Improved management of arable soils holds significant potential for mitigating greenhouse gas emissions, as agroecosystems contribute ca. 66 % of total anthropogenic N2O emissions (Davidson and Kanter, 2014; Paustian et al., 2016; Shen et al., 2021). Efficient mitigation of N loss requires a comprehensive understanding of microbial processes related to N2O emissions in agricultural soils (Davidson and Kanter, 2014; Shen et al., 2021).
The general objectives of the study were to evaluate temporal patterns of gaseous N loss, link N cycle processes to abundances of functional N cycle genes in arable soil and evaluate the performance of different crops (including novel crops in northern Europe) in terms of biomass production and N2O emissions under mineral and organic fertilisation. The following hypotheses were tested: (1) crop type significantly affects N2O emissions, (2) nitrification is the primary pathway of soil N2O production due to aerobic conditions, (3) low soil moisture results in reduced N2O losses in arable soil and (4) amendment of manure fertiliser increases soil N2O emissions and affects the abundances of functional N cycle genes.
2.1 Field experiment description
The field study was conducted in the International Organic Nitrogen Long-term Fertilisation Experiment (IOSDV; Internationaler Organischer Stickstoff Dauerdüngungs Versuch) field. The experimental site is located near Tartu, southern Estonia, in northern Europe (58°22′30′′ N, 26°39′48′′ E). The experiment was set up as a three-field crop rotation experiment in 1989 to investigate the long-term effects of mineral and organic fertilisation on responses of various crops and soil properties.
In 2022, the average temperatures in the area were −2.0 °C in winter, 4.6 °C in spring, 18.1 °C in summer and 7.2 °C in autumn. The mean annual precipitation was 531 mm (Republic of Estonia Environment Agency, 2023) in 2022. A climate diagram for the area during the study period is in Fig. S1 in the Supplement.
The soil type is Stagnic Luvisol combined with Fragic Glossic Retisol (IUSS WG WRB, 2015). The thickness of the humus layer is 27–32 cm. The soil texture by FAO classification is sandy loam: 57.86 % sand (>0.063 mm), 33.58 % silt (0.063–0.002 mm) and 8.55 % clay (<0.002 mm). The soil bulk density was in the range of 1.5 to 1.6 g cm−3, with slightly lower values for manure treatment plots. The average pH levels in spring 2022 were 5.4 for barley plots, 5.3 for wheat plots, 5.6 for sorghum plots without manure amendment and 6.2 for sorghum plots with manure amendment.
The experiment was organised into 12 plots in a systematic block design (Fig. 1) with three sampling spots per plot. Every plot was 50 m2 in size. The crop species studied were spring barley (cultivar “Elmeri”), sorghum (Sorghum bicolor × Sorghum sudanense, cultivar “SUSU”) and spring wheat (cultivar “Mistral”). Initially, the crop rotation was potato–spring wheat–spring barley (Astover et al., 2016). In 2019, potato was replaced with a sorghum–Sudan grass hybrid.
The fertiliser treatment consisted of mineral N fertilisation and mineral fertilisation with farmyard manure amendment. All fertilisation treatments are applied continuously from the year 1989, when the experimental site was established. Three mineral N fertiliser treatment rates were studied: 0, 80 and 160 kg N ha−1. The farmyard manure rate added to the sorghum plots was 40 t ha−1 of manure (231.2 kg N ha−1). The mineral fertiliser applied was ammonium nitrate (NH4NO3) and the organic fertiliser was farmyard manure. The farmyard manure was cattle dung with straw bedding, freely fermented before use for 6–8 months in a heap. The chemical properties (C, N, P and K) of manure added in 2022 and during the last 10 years are presented in Table S1 in the Supplement. The farmyard manure treatment with mineral fertilisation was only applied to sorghum. Manure treatment is amended with solid farmyard manure (40 t ha−1) in every third year before sorghum and potato. The main management activities and timing in the field are displayed in Table S2.
2.2 Gas sampling for N2O flux analyses
The field study was conducted during the growing season from April to October 2022. Sampling took place on 15 different dates, starting on 27 April and ending on 12 October (every week until the end of June and then twice a month until the end of September). Gas samples for N2O flux analysis were collected on all 15 fieldwork days. N2O gas sampling was carried out using the static chamber method (Hutchinson and Livingston, 1993). Polyvinyl chloride chambers (Ø 50 cm, volume 65 L) were placed on top of the collars during the gas sampling. Chamber extensions were used for some treatments of sorghum on four occasions as the chambers alone were too small to accommodate the growing crops. Pre-vacuumed 50 mL glass vials were used for gas sampling. Gas samples were collected at 20 min intervals for 1 h (0, 20, 40 and 60 min). The concentration of N2O in the collected air was measured in the Biogeochemical Cycling Research Laboratory in the Department of Geography, University of Tartu, with the gas chromatograph Shimadzu GC-2014 (Kyoto, Japan) equipped with electron capture and flame ionisation detectors (Poole, 2015).
2.3 Soil sampling and physicochemical analyses
Soils were sampled for chemical and microbiological analyses six times (27 April, 9 May, 2 June, 7 July, 2 September and 12 October). Soil sampling was conducted after gas sampling. Soil samples were collected close to collars with a soil probe from the top 10 cm of the soil. Three auger samples from each point (both bulk and rhizosphere soils were sampled) were collected for one composite sample for chemical and microbiological analyses. All in all, 216 samples were collected for chemical analyses and 144 samples for microbial analyses. Until chemical and microbiological analyses, samples were stored at +4 and −20 °C, respectively. In addition to soil sampling, soil temperature (° C) at a depth of 10 cm was measured with a temperature logger (Comet Systems Ltd., Rožnov pod Radhoštem, Czech Republic) and soil moisture (m3 m−3) was recorded using water content reflectometers (model CS615, Campbell Scientific Inc., Logan, UT, USA). The soil samples were analysed for total carbon (Ctot), total nitrogen (Ntot), nitrate-nitrogen (NO-N) and ammonium-nitrogen (NH-N) concentrations in the Soil Science and Agrochemistry Laboratory of the Estonian University of Life Sciences. Ntot and Ctot analyses were done using the Dumas method (International Organization for Standardization, 1998) with dry combustion on a VarioMAX CNS elemental analyser (ELEMENTAR, Elementar Analysensysteme GmbH, Langenselbold, Germany). NO-N analyses were done according to EPA (United States Environmental Protection Agency) method 9056: determination of inorganic anions by ion chromatography. NH-N analyses were done according to Thermo Fisher Application Note 141 (AU204: Determination of Inorganic Cations and Ammonium in Environmental Waters Using a Compact Ion Chromatography System) using ion chromatography. Soil pH was measured using a glass-electrode pH meter in a 1 : 2.5 water solution. Total phosphorus (P) and potassium (K) concentrations in manure were determined through acid digestion using a sulfuric acid solution (van Reeuwijk, 2002).
The hot-water extractable C (HWEOC) represents the readily mineralising C fraction and was determined on dry soil samples using a modified method of Haynes and Francis (1993) in two steps. In the first step the soil was shaken with deionised water at room temperature for 1 h. After that the soil suspension was put into the thermostat at 80 °C for 16 h. The mixture was centrifuged for 10 min at 8000 rpm and filtered through a 0.45 µm membrane filter (25 mm diameter, nylon, Agilent®). The HWEOC concentration was determined from the extracts using the VarioMaX CNS analyser (ELEMENTAR, Elementar Analysensysteme GmbH, Langenselbold, Germany).
2.4 Total biomass
The total (above- and below-ground) biomass was measured in the maturity phase on the harvest day of each crop (Table S2). The above-ground biomass was cut from the ground level in a 0.2 m2 area near each collar. The below-ground biomass samples were taken with a soil auger (Ø 34 cm). Frasier et al. (2016) provide a more detailed description of the method used for below-ground biomass measurement. The sampling depth extended to the ploughing depth, where most of the roots are found, up to a depth of 18 cm. Samples were stored at +4 °C until the roots were washed on a sieve (mesh size 0.5 mm).
Dry matter yield was determined after drying the biomass (including roots) at 70 °C to a constant weight. The straw and grains were separated before weighing as air dry. The biomass (straw, grain and roots) was milled and the Ntot content was determined using the Dumas method with dry combustion on a VarioMAX CNS elemental analyser (ELEMENTAR, Elementar Analysensysteme GmbH, Langenselbold, Germany).
2.5 Soil microbial analyses
2.5.1 DNA extraction
DNA was extracted from 0.25 g of soil samples using the DNeasy® PowerSoil® Pro Kit (Qiagen, Hilden, Germany) following the manufacturer's instructions. The difference from the instructions was the homogenisation of samples with a homogeniser, Precellys 24 (Bertin Technologies, Montaigne-le-Bretonneux, France), for 20 s at a rate of 5000 rpm. The concentration and quality of the extracted DNA were evaluated with an Infinite 200 M spectrophotometer (Tecan AG, Männedorf, Switzerland). The extracted DNA was stored in a freezer at −20 °C.
2.5.2 Quantification of gene copies using a quantitative polymerase chain reaction (qPCR)
Quantification of the 16S rRNA genes of bacteria and archaea, along with the quantification of nitrification (bacterial, archaeal and comammox amoA), denitrification (nirS, nirK, nosZI and nosZII) and DNRA (nrfA) genes was done using qPCR. qPCR reactions were performed using the Rotor-Gene® Q thermocycler (Qiagen). The reaction mixture of 10 µL consisted of extracted DNA (1 µL), gene-specific forward and reverse primers, a Maxima SYBR Green Master mix reagent (5 µL; Thermo Fisher Scientific, Waltham, MA, USA) and distilled water. Each sample was amplified two times. All of the qPCR assays included two DNA-free negative control samples. Details of the thermal cycling conditions and the primers used are added in Table S3. The Rotor-Gene® Q software v. 2.0.2 (Qiagen) and LinRegPCR v. 2020.2 were used to assess the qPCR results. The number of gene copies was calculated using standard curve ranges, and the results were presented in gene copies per gram of dry matter (copies g dw−1). Espenberg et al. (2018) provide a more detailed description of the qPCR methodology used.
2.6 Statistical analyses and modelling
The statistical software programs Statistica (v. 7.1) and R (v. 4.0.4) were used for the statistical analyses and for visualising the data. Principal component analysis (PCA) was conducted on soil physicochemical parameters and microbiological data (abundance of functional marker genes) with the FactoMineR (Lê et al., 2008) and factoextra (Kassambara and Mundt, 2020) packages in the R software. An analysis of variance (ANOVA) with a post hoc Tukey honest significant difference (HSD) test was used (the cumulative N2O emission values meet the assumptions of the parametric test) to find statistically significant differences between different fertilisation rates and the use of manure and crop types.
Spearman's rank correlation coefficient measured the association between N2O and N2 emissions, gene abundances and environmental factors. Random forest classification analysis was conducted using Boruta v. 8.0 (Kursa and Rudnicki, 2010) to identify the gene parameters that best predicted N2O fluxes.
Nitrogen use efficiency (NUE, kg DM kg−1 N−1) was calculated as the biomass production per unit of N applied (Pandey et al., 2001; Methodology, Sect. S1 in the Supplement). The N2 emissions were estimated from the measured N2O emissions using the N2 : N2O ratio, which was calculated as proposed in the DAYCENT model (Parton et al., 2001) with the equations described in Del Grosso et al. (2000) (Methodology, Sect. S2), where the N2 : N2O ratio is a function of the content of NO in the soil, CO2 emissions and the water-filled pore space (WFPS). The change in the soil N content (kg N ha−1) was calculated according to Sainju (2017) as the difference between the initial and final soil total N contents (Methodology, Sect. S3). N losses are calculated by subtracting N outputs and changes in soil N content from N inputs (Sainju, 2017; Escuer-Gatius et al., 2022; Methodology, Sect. S4).
A linear mixed-effect model (LMM) was used to investigate differences in N2O emissions and gene parameters between different crop types and fertilisation rates using the R package nlme. For N2O emissions and gene parameters, a temporal (sampling date) effect was used as a random effect. The Kruskal–Wallis test and the post hoc Tukey HSD test were used to compare the N2O and gene parameter (not meeting the assumptions of a parametric test) values between different crop types and fertilisation rates. Due to the limited number of observations in the case of total dry weight biomass, N content in biomass and cumulative N2 emissions, it was not possible to apply the LMM for statistical differences between different fertilisation rates and crop types.
3.1 Soil physicochemical characteristics and biomass production
The NH-N content in the soil decreased in most of the plots at the beginning of the study period, while the NO-N content increased (Fig. S2). Fertilised plots had higher soil Ntot, Ctot, NO-N and NH-N contents compared to non-fertilised plots according to the PCA (Figs. 2, S2 and S3). For sorghum plots without manure amendment (Fig. 2c), the NO-N and NH-N contents were more different from each other compared to sorghum plots with manure amendment, where the NO-N and NH-N contents were relatively similar (Fig. 2d). HWEOC concentrations were higher in sorghum plots with farmyard manure amendment compared to sorghum plots without manure amendment.
Soil moisture ranged from 0.02 to 0.32 m3 m−3, with an average of 0.23 m3 m−3 over the study period (Fig. S4). There were no significant correlations between soil moisture and N2O emissions (Table S4). Soil moisture was not significantly linked to gene copy numbers across all the crop types, except for nirS. A climate diagram for the area during the study period is presented in Fig. S1.
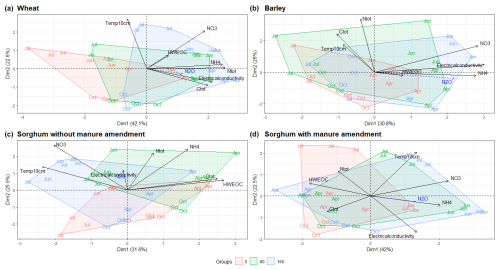
Figure 2PCA ordination plots demonstrate the grouping of fertilisation rates according to physicochemical parameters for different crop types. N2O is added as a supplementary variable. The month indicates the sampling time. Abbreviations: Ctot – total carbon content of soil; Ntot – total nitrogen content of soil; HWEOC – hot-water extractable organic carbon.
The total dry biomass of barley ranged from 2.6 to 6.4 t ha−1 and that of wheat from 4.6 to 8.5 t ha−1, depending on the mineral N fertilisation rate (Fig. 3). For sorghum without manure amendment, the total dry biomass varied between 2.3 and 7.1 t ha−1, and for sorghum with manure amendment the total dry biomass varied between 8.2 and 11.7 t ha−1.
The biomass production was higher per unit area of crop growth with higher fertiliser input (Fig. 3a). Total biomass was significantly positively correlated with Ntot (p<0.01), Ctot (p<0.05) and NO-N (p<0.001) levels in soil (Table S5). Also, a higher N fertilisation rate caused an increase in N content in the crop biomass (Fig. 3b).
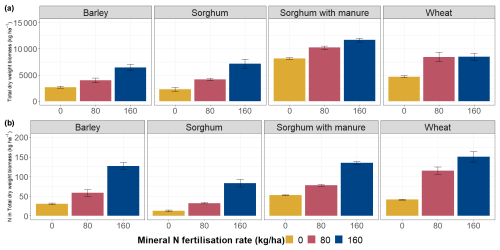
Figure 3Total dry weight (above-ground + below-ground) biomass produced per unit area according to crop types and fertilisation rates. The error bars show standard errors.
The highest values of the NUE were obtained from wheat plots and the lowest values from sorghum plots. The average NUE of wheat plots at fertilisation rate 80 was 0.84, and at fertilisation rate 160 it was 0.64. For sorghum plots with manure amendment, the NUE at mineral N fertilisation rate 0 was 0.15, at fertilisation rate 80 it was 0.16 and at fertilisation rate 160 it was 0.25. For sorghum plots without manure amendment, the average NUE at fertilisation rate 80 was 0.12, and at fertilisation rate 160 it was 0.25. The NUE for barley plots at fertilisation rate 80 was 0.35, and at fertilisation rate 160 it was 0.45. The highest estimated N losses occurred on sorghum plots with manure amendment (Table S6). In general, wheat plots at different fertilisation rates lost more N compared to sorghum plots without manure amendment. The lowest estimated N losses occurred on barley plots.
3.2 Nitrogen cycle genes
The abundances of N cycling genes on plots with different fertilisation rates and crop species show different patterns throughout the study period (Figs. S5, S6, S7 and S8). The PCA of the N cycle gene abundances showed differences between sites with different fertilisation rates (Fig. 4). There were greater differences in gene abundances between three different mineral N fertilisation rates in sorghum plots compared to barley and wheat plots (Fig. 4). For sorghum without manure amendment (Fig. 4c), archaeal 16S rRNA and nosZII gene abundances were highest for fertilisation rate 80 compared to fertilisation rates 0 and 160 (p<0.001), but for sorghum with manure amendment (Fig. 4d) the highest archaeal 16S rRNA and nosZII gene abundances were for fertilisation rate 160 compared to fertilisation rates 0 (p<0.001) and 80 (p<0.05). For all the sorghum plots, comammox amoA gene abundance was highest on non-fertilised plots. However, fertilised wheat and barley plots had a higher comammox amoA gene abundance compared to non-fertilised plots.
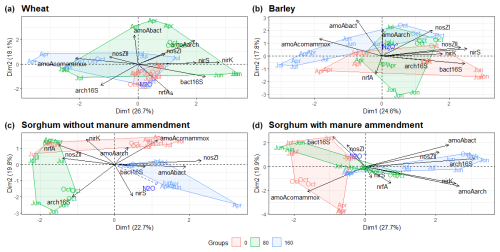
Figure 4PCA ordination plots demonstrate the grouping of fertilisation rates according to functional marker gene abundances for different crop types. N2O is added as a supplementary variable. The month shows the sampling time. Abbreviations: bact16S – bacterial 16S rRNA gene; arch16S – archaeal 16S rRNA gene; amoAbact – bacterial amoA gene; amoAarch – archaeal amoA gene; amoAcomammox – comammox amoA gene.
3.3 N2O emissions
The N2O emissions over the course of the study period show that different fertilisation rates influence N2O emissions and the highest N2O emissions tend to be emitted from the highest N fertiliser treatment (160 kg N ha−1) (Fig. 5). N2O emissions among all the crop species tended to be higher during the first part of the study period (spring and early summer). Taken together, the highest average N2O emissions for the barley plots were measured in the middle of May, for the sorghum plots without and with manure in the middle of June and for the wheat plots at the beginning of June.
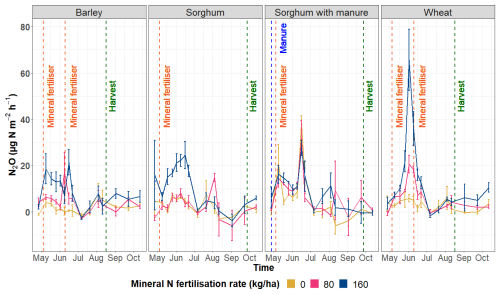
Figure 5N2O emissions (µg N m−2 h−1) according to crop types and fertilisation rates during the study period.
Throughout the study period, cumulative N2O and N2 emissions were highest in plots with the highest fertilisation rates, except for sorghum plots with manure amendment (Fig. 6a, b). For wheat and barley plots, there is a clear pattern of increasing N2O emissions with increasing fertilisation rates.
For barley plots, cumulative N2O emissions did not differ significantly between fertilisation rates 0 and 80 (Fig. 6a). However, N2O emissions on barley plots were significantly higher at fertilisation rate 160 than at fertilisation rates 0 and 80 (p<0.05). Similarly, for wheat plots, cumulative N2O emissions were also significantly higher at fertilisation rate 160 compared to fertilisation rates 0 (p<0.05) and 80 (p<0.05); however, fertilisation rates 0 and 80 did not differ significantly from each other. For plots with sorghum without manure, cumulative N2O emissions at fertilisation rate 160 were significantly higher compared to fertilisation rates 0 (p<0.05) and 80 (p<0.05). For sorghum with manure plots, cumulative N2O emissions at fertilisation rate 160 were significantly different compared to fertilisation rates 0 (p<0.05) and 80 (p<0.05).
For barley and wheat plots, the cumulative N2 emissions were higher at fertilisation rate 160 compared to fertilisation rates 0 and 80 (Fig. 6b). For sorghum plots, the cumulative N2 emissions from all three fertilisation rates were similar.
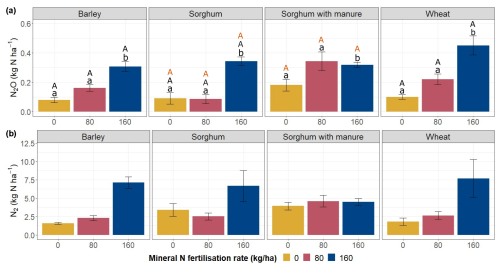
Figure 6Cumulative N2O and N2 emissions according to crop types and fertilisation rates. Error bars show standard errors. Letters above the boxes indicate statistically significant differences at the significance level p<0.05 according to a post hoc Tukey HSD test. Lower-case letters indicate comparisons within crop types. Upper-case letters indicate comparisons of the same fertilisation rate between different crop types: orange upper-case letters represent comparisons between sorghum without manure amendment and sorghum with manure amendment; black upper-case letters represent comparisons between barley, wheat and sorghum without manure amendment.
3.4 Relationships between environmental parameters, gene abundances/ratios and N emissions
Mineral N fertilisation rate (p<0.001) and crop type (p<0.05) significantly influenced N2O emissions on plots with only mineral N fertilisation (Table 1a). Manure amendment (p<0.05) and mineral N fertilisation rate (p<0.05) had a significant impact on N2O emissions from sorghum plots (Table 1b).
Table 1Results of ANOVA testing of the effects of (a) the mineral N fertilisation rate and crop type on cumulative N2O fluxes on plots with barley, wheat and sorghum without manure amendment and (b) the mineral N fertilisation rate and manure amendment on cumulative N2O fluxes on sorghum plots with and without manure amendment. Significance is indicated as – 0.001, – 0.01, * – 0.05 and ns – not significant.

Random forest classification analysis for the N2O emissions from wheat plots considered bacterial amoA, archaeal amoA, nosZI and nosZII genes to be relevant (Fig. 7). For barley plots, bacterial amoA, comammox amoA, bacterial 16S rRNA, nirK, nirS and nosZII genes were deemed important in the variations of N2O emissions. For sorghum without manure amendment plots, bacterial amoA, comammox amoA, archaeal 16S rRNA and nirK genes were considered important for the N2O emissions. For sorghum with manure amendment plots, archaeal amoA, bacterial amoA, comammox amoA, nirK, nirS, nosZII, nosZI and nrfA genes were considered important for the N2O emissions.
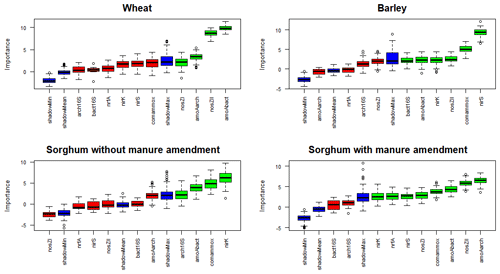
Figure 7Results of feature selection in predicting the genes that are important in the variations of N2O emissions. Important factors are indicated in green, unimportant factors in red and shadow variables (the random shadow copies of features (noise) will be created to test the feature against those copies to determine whether it is better than the noise and therefore significant) in blue. Abbreviations: bact16S – bacterial 16S rRNA gene; arch16S – archaeal 16S rRNA gene; amoAbact – bacterial amoA gene; amoAarch – archaeal amoA gene; amoAcomammox – comammox amoA gene.
The relationships between gene abundances and N2O emissions showed that the ratio of amoA to nir had a significant positive correlation with N2O emissions (ρ=0.20; p<0.001). Furthermore, the ratio of nosZ to nir was significantly positively correlated with N2O emissions (ρ=0.21; p<0.001). nirS genes were positively correlated with N2O emissions over all crop species plots (ρ=0.19; p<0.05). N2O emissions from barley plots also had a strong positive correlation with nirS gene abundance (ρ=0.58; p<0.001). On wheat plots, nosZII genes were negatively correlated with N2O emissions (; p<0.01). The correlation matrix is provided as Table S7.
The relationship between N2 emissions and nrfA genes showed that N2 emissions were negatively correlated with nrfA genes over all the crop types (; p<0.05). nosZII genes were positively correlated with N2 emissions on plots with wheat (ρ=0.85; p<0.01).
Mineral N fertilisation positively influenced biomass increase in all three crop types (Fig. 3a), with similar findings observed in other IOSDV experiments by Csitári et al. (2021) and Tajnšek et al. (2013). The results also showed a significant positive correlation between biomass production and soil NON, Ctot and Ntot contents, explaining higher biomass production in fertilised soil, as N limitation is the most influential factor constraining crop growth (Mengel and Kirkby, 2001). Furthermore, increasing mineral N fertilisation led to higher N accumulation in the biomass (Fig. 3). The higher N content in the biomass can be explained by applying N at rates that exceed crop needs for optimal yield, leading to an increase in crop protein content (Serret et al., 2008; Mengel and Kirkby, 2001).
The sorghum plots without fertilisation yielded 2.3 t ha−1, while those with manure amendment only produced an additional 5.9 t ha−1 of total dry biomass (Fig. 3a), consistent with the results from Spiegel et al. (2010). Ntot and Ctot were also higher on sorghum plots with manure amendment compared to plots with mineral fertilisation only (Fig. S3), which could explain the higher biomass production. The positive effect of manure amendment could be attributed to increased availability of nutrients. Meta-analysis by Hijbeek et al. (2017), covering 20 long-term experiments (including the IOSDV experimental site used in our study) in Europe, reported that organic input does not necessarily guarantee increased crop yields, although Hijbeek et al. (2017) also found that, in specific cases, like spring-sown cereals and sandy soils, the use of organic inputs led to an increase in crop yield.
In various ecosystems, N cycle genes have been linked to N2O emissions (Butterbach-Bahl et al., 2013; Espenberg et al., 2018; Harter et al., 2014). The significant positive correlation between the ratio of amoA to nir genes and N2O emissions (ρ=0.20; p<0.001) in our study indicates that nitrification potential was higher than denitrification potential, and N2O emissions were thereby mainly related to nitrification in the soil. Previous studies have also used the ratio of amoA to nir genes to study N cycle processes (Kazmi et al., 2023; Tang et al., 2018; Zhu et al., 2018). Additionally, an initial decrease in NH-N content in soil was observed, suggesting NH consumption (nitrification) and mineral N uptake by plants (Fig. S2). A simultaneous increase in NO-N accompanied by a decrease in NH-N was recorded, likely resulting from the nitrification production process.
nirS genes exhibited a positive correlation with N2O emissions across all the crop species plots (ρ=0.19; p<0.05), suggesting that, while nitrification is predominant, denitrification is also evident. This finding aligns with results from several other agricultural studies, which also reported a significant positive correlation between nirS genes and N2O emissions (Castellano-Hinojosa et al., 2020; Cui et al., 2016). Additionally, the ratio of nosZ to nir genes (nosZ / nir) was positively correlated with N2O emissions (ρ=0.21; p<0.001). This highlights the importance of complete denitrifiers that have a capacity to convert N2O to N2. Since N2O emissions are increasing with a high abundance of the nosZ gene, this positive correlation may also be related to N2O emissions being emitted from nitrification.
For all the plots, one or more functional marker genes related to nitrification and denitrification were identified as important in the variations of N2O emissions (Fig. 7), emphasising the significance of both processes in N2O emissions. Comammox was also recognised as an important process in N2O emissions, except on wheat plots, indicating its potentially important role. Additionally, Li et al. (2019) demonstrated an order of magnitude higher abundance of comammox Nitrospira clade A compared to ammonia-oxidising archaea and ammonia-oxidising bacteria in fertilised agricultural soil. More functional marker genes show significance in the variations of N2O with manure compared to other treatments (Fig. 7), indicating that a greater number of N cycle processes are relevant for plots with manure. Additionally, nosZI, nosZII and nirS genes were identified as important in the variations of N2O emissions for sorghum with manure amendment but not for mineral fertiliser sorghum plots, which indicates the significance of denitrification in these plots. Previous studies also suggest a higher denitrification potential from manure treatment, highlighting the importance of denitrifying microorganisms in manure-fertilised plots (Clark et al., 2012; Wan et al., 2023). The increased denitrification rate in manure-amended plots may be due to improved soil water retention promoting denitrification and increased availability of labile C content, which is the energy source for denitrifiers (Lazcano et al., 2021; Rayne and Aula, 2020). Our results also support a higher labile C content in plots with manure amendment (Fig. 2). Furthermore, sorghum plots with manure were the only ones where the nrfA gene was identified as important in N2O emissions, suggesting that manure amendment is likely enhancing the rate of the DNRA process.
The negative relationship between the nrfA gene and N2 emissions suggests that the DNRA process is not contributing to the N2 emissions. The DNRA process, which is mediated by the nrfA gene, is beneficial as it supplies the soil with NH and conserves the bioavailable N (Bai et al., 2020; Pandey et al., 2020). In addition, a significant positive correlation between nosZII genes and N2 emissions on plots with wheat indicates that there is likely a potential production of N2 due to the high abundance of nosZII genes that reduce N2O to inert N2. This is also supported by the negative correlation between nosZII genes and N2O emissions (; p<0.01) on wheat plots and indicates the nosZII genes' role in reducing N2O emissions (Graf et al., 2014). Jones et al. (2014) demonstrated that the abundance and phylogenetic diversity of the nosZII community are important factors driving the soil's N2O sink capacity.
Agricultural soils typically act as a source of N2O (Davidson and Kanter, 2014), as shown in this study. The three mineral N fertilisation rates investigated influenced N2O emissions, with N2O emissions increasing with a higher mineral N application rate for all three crop species (Figs. 5 and 6a). This can be attributed to higher available N levels with increased fertilisation rates for processes contributing to N2O emissions (Engel et al., 2010), as N2O emissions showed a strong positive correlation with both NO-N and NH-N levels in soil. Prior studies have also highlighted a positive relationship between soil N2O emissions and mineral N content (Sosulski et al., 2014; Yao et al., 2009; Yuan et al., 2022). Furthermore, among the investigated factors, the mineral N fertilisation rate was the primary determinant of cumulative N2O emissions (Table 1), indicating that soil N2O emissions are mainly linked to the excess N added with mineral fertiliser in the cropping system (Table S6). However, it is important to consider that the sample replicates may not be fully statistically independent, as the experiment involved only one plot per treatment combination (crop type × fertilisation rate), with three samples taken within the same plot.
Our study found that both crop type and mineral N fertilisation rate significantly influence cumulative N2O emissions, with mineral N fertilisation rate having a stronger effect (Table 1). This suggests that N2O emissions from soil are more closely related to the excess N in the cropping system than the crop type. Some studies have also shown a significant effect of crop type on N2O emissions (Bouwman et al., 2002; Kaiser and Ruser, 2000). However, a study including 372 sites also showed that cover crops did not have a significant (p>0.05) effect on N2O emissions (Abdalla et al., 2019). Manure amendment significantly impacted N2O emissions (Table 1b). Additionally, mineral fertiliser plus manure amendment showed higher soil N2O emissions compared to mineral fertiliser alone for sorghum. This can be attributed to the overall higher mineral input of N into the cropping system in mineral fertiliser plus manure plots compared to plots with mineral fertiliser only (231.2 kg N ha−1 was added extra), enhancing N2O production. In addition to providing nitrifiable N compounds, manure incorporation improves soil conditions for nitrification and denitrification by increasing moisture and adding C to the soil (Chadwick et al., 2000). While the increase in moisture with manure was not detectable from our study, it may be explained by the slow evolution of soil properties over previous years in the 33-year-long fertilisation experiment. Moreover, manure can enhance the activity of soil microbes, oxygen consumption and the development of anaerobic zones in the soil, favouring denitrification (Akiyama and Tsuruta, 2003).
Soil microbial processes leading to N2O production are influenced by soil water content, as this directly affects oxygen availability for nitrification and denitrification processes. The lowest recorded soil moisture contents for barley and wheat plots on 7 July (Fig. S4) likely explain the lowest N2O emissions on that date (Fig. 5). Previous studies on N2O emissions and soil moisture dynamics have reported a similar trend (Yamulki et al., 1995; Yuan et al., 2022). Additionally, Thapa et al. (2017) reported a reduction in N2O emissions from wheat fields, which could be due to soil salinity interfering with nitrification and denitrification processes (Dang et al., 2016). Dry soils may lead to microorganisms experiencing cell dehydration and increased soil salinity, hindering soil microbial activity and, therefore, the production of gaseous N emissions (Haj-Amor et al., 2022; Schimel, 2018), although our study did not find significant correlations between soil moisture and N2O emissions for most of the functional marker genes.
Considering climate changes and population growth, N2O management should be aligned with the future need to increase crop yield and sustain a rapidly increasing human population. Biomass production increased with fertilisation rate (Fig. 3), except for wheat plots. In our study, the biomass production on wheat plots between fertilisation rates 80 and 160 kg N ha−1 had very similar biomass values. However, long-term fertilisation experiments (IOSDV) by Káš et al. (2010) achieved the highest wheat yields from an N fertilisation rate of 160 kg N ha−1. Our study shows increasing N2O emissions at a higher fertilisation rate on wheat plots (Fig. 6a), indicating potential over-fertilisation and suggesting a fertilisation rate at 80 kg N ha−1 as the optimal fertilisation rate. In addition, the highest NUE was observed at fertilisation rate 80 kg N ha−1 for wheat (NUE = 0.84), indicating a balance between low N2O emissions and high yield. In India, Chaturvedi (2006) conducted similar fertilisation experiments with N fertilisation rates of 0, 25, 50, 75, 100 and 125 kg N ha−1 and identified the highest N input rate as optimal.
At a fertilisation rate of 160 kg N ha−1, N2O emissions increased significantly compared to lower rates, but this rate also results in a higher total dry biomass (Figs. 3 and 6). The fertilisation rate of 80 kg N ha−1 for sorghum plots with mineral N fertiliser amendment only appears optimal, with low N2O emissions and N losses (Table S6). However, for sorghum plots without manure amendment, NUE values are low (160 kg N ha−1 NUE = 0.25; 80 kg N ha−1 NUE = 0.12).
The results of our study (part of the 33-year-long IOSDV experiment) showed that the mineral N fertilisation rate was the dominant factor determining cumulative N2O emissions. Crop type also had a significant effect on N2O emissions in this study. The study observed an increase in N2O emissions with an elevated mineral N fertilisation rate, attributed to higher NO-N and NH-N levels in fertilised soil. These findings supported our hypothesis of higher N2O emissions on sorghum plots under mineral fertiliser plus manure treatment compared to mineral fertiliser treatment only. Additionally, the number of N cycle genes that are significant in the variations of N2O emissions also increased with manure amendment.
N2O emissions were mostly caused by nitrification, with potential contributions from denitrification, comammox and DNRA processes. Plots with manure amendment exhibited a greater impact of N cycle microbial processes on N2O emissions compared to plots with other crop types. Soil moisture showed no correlation with N2O emissions and most of the functional marker gene abundances. Nonetheless, the lowest N2O emissions and functional marker gene abundances were recorded during periods of low soil moisture, suggesting a decrease in N2O under such conditions.
For wheat, a high NUE value and low N2O emissions, coupled with a relatively high crop yield, suggest that a fertilisation rate of 80 kg N ha−1 is optimal. Similarly, on sorghum plots with only mineral N fertiliser amendment, a fertilisation rate of 80 kg N ha−1 resulted in low N2O emissions and N losses considering comparable biomass production with other crop types.
The code will be made available in response to a reasonable request.
The data presented in this paper are available upon reasonable request to the corresponding author.
The supplement related to this article is available online at: https://doi.org/10.5194/soil-11-1-2025-supplement.
ME, AA and ÜM designed the experiment and developed the methodology. LK and JEG carried out the fieldwork. LK analysed the results, performed the data visualisation and wrote the original manuscript. JEG and ME participated in the data analyses and assisted with the paper editing. LK, ME, JEG, KK, AA, ÜM and KS were involved in revising the paper for submission and contributed to its improvement.
The contact author has declared that none of the authors has any competing interests.
Publisher's note: Copernicus Publications remains neutral with regard to jurisdictional claims made in the text, published maps, institutional affiliations, or any other geographical representation in this paper. While Copernicus Publications makes every effort to include appropriate place names, the final responsibility lies with the authors.
This article is part of the special issue “Trade-offs and synergies of soil carbon sequestration and environmental impacts: implications for agricultural management”. It is a result of the EGU 2023, Vienna, Austria, 23–28 April 2023.
We thank Triin Teesalu for assisting with the soil sampling for the chemical analyses. Thanks go to Tõnu Tõnutare and Kristi Kõva for conducting the soil chemical analysis. We would also like to acknowledge Avo Toomsoo for operating the fertilisation experiment and maintaining the study field.
The study was supported by the Estonian Research Council (grant nos. PRG352 and PRG2032), the European Research Council (ERC) under grant agreement no. 101096403 (MLTOM23415R), the European Commission through the HORIZON-WIDERA “Living Labs for Wetland Forest Research” Twinning project (grant no. 101079192) and the European Regional Development Fund (Centres of Excellence EcolChange, TK131, and AgroCropFuture, TK200).
This paper was edited by Cristina Aponte and reviewed by three anonymous referees.
Abdalla, M., Hastings, A., Cheng, K., Yue, Q., Chadwick, D., Espenberg, M., Truu, J., Rees, R. M., and Smith, P.: A critical review of the impacts of cover crops on nitrogen leaching, net greenhouse gas balance and crop productivity, Glob. Change Biol., 25, 2530–2543, https://doi.org/10.1111/gcb.14644, 2019.
Abdalla, M., Shang, Z., Espenberg, M., Cui, X., Mander, Ü., and Smith, P.: Impacts of crop type, management and soil quality indicators on background nitrous oxide emissions (BNE) from Chinese croplands: A quantitative review and analysis, Environmental Science: Atmospheres, 2, 563–573, https://doi.org/10.1039/D2EA00033D, 2022.
Akiyama, H. and Tsuruta, H.: Effect of organic matter application on N2O, NO, and NO2 fluxes from an Andisol field, Global Biogeochem. Cy., 17, 1100, https://doi.org/10.1029/2002GB002016, 2003.
Anderson, R., Bayer, P. E., and Edwards, D.: Climate change and the need for agricultural adaptation, Curr. Opin. Plant Biol., 56, 197–202, https://doi.org/10.1016/j.pbi.2019.12.006, 2020.
Astover, A., Szajdak, L. W., and Kõlli, R.: Impact of Long-Term Agricultural Management and Native Forest Ecosystem on the Chemical and Biochemical Properties of Retisols' Organic Matter, in: Bioactive Compounds in Agricultural Soils, edited by: Szajdak, L. W., 149–171, Springer International Publishing, https://doi.org/10.1007/978-3-319-43107-9_7, 2016.
Ayiti, O. E. and Babalola, O. O.: Factors Influencing Soil Nitrification Process and the Effect on Environment and Health, Frontiers in Sustainable Food Systems, 6, 821994, https://doi.org/10.3389/fsufs.2022.821994, 2022.
Bai, R., Fang, Y.-T., Mo, L.-Y., Shen, J.-P., Song, L.-L., Wang, Y.-Q., Zhang, L.-M., and He, J.-Z.: Greater promotion of DNRA rates and nrfA gene transcriptional activity by straw incorporation in alkaline than in acidic paddy soils, Soil Ecol. Lett., 2, 255–267, https://doi.org/10.1007/s42832-020-0050-6, 2020.
Beeckman, F., Motte, H., and Beeckman, T.: Nitrification in agricultural soils: Impact, actors and mitigation, Curr. Opin. Biotech., 50, 166–173, https://doi.org/10.1016/j.copbio.2018.01.014, 2018.
Bouwman, A. F., Boumans, L. J. M., and Batjes, N. H.: Modeling global annual N2O and NO emissions from fertilized fields, Global Biogeochem. Cy., 16, 28-1–28-9, https://doi.org/10.1029/2001GB001812, 2002.
Butterbach-Bahl, K., Baggs, E. M., Dannenmann, M., Kiese, R., and Zechmeister-Boltenstern, S.: Nitrous oxide emissions from soils: How well do we understand the processes and their controls?, Philos. T. R. Soc. B, 368, 20130122, https://doi.org/10.1098/rstb.2013.0122, 2013.
Cameron, K. C., Di, H. J., and Moir, J. L.: Nitrogen losses from the soil/plant system: A review, Ann. Appl. Biol., 162, 145–173, https://doi.org/10.1111/aab.12014, 2013.
Castellano-Hinojosa, A., Correa-Galeote, D., González-López, J., and Bedmar, E. J.: Effect of nitrogen fertilisers on nitrous oxide emission, nitrifier and denitrifier abundance and bacterial diversity in closed ecological systems, Appl. Soil Ecol., 145, 103380, https://doi.org/10.1016/j.apsoil.2019.103380, 2020.
Chadwick, D. R., Pain, B. F., and Brookman, S. K. E.: Nitrous Oxide and Methane Emissions following Application of Animal Manures to Grassland, J. Environ. Qual., 29, 277–287, https://doi.org/10.2134/jeq2000.00472425002900010035x, 2000.
Chaturvedi, I.: Effects of different nitrogen levels on growth, yield and nutrient uptake of wheat (Triticum aestivum L.), Int. J. Agr. Sci., 2, 372–374, 2006.
Clark, I. M., Buchkina, N., Jhurreea, D., Goulding, K. W. T., and Hirsch, P. R.: Impacts of nitrogen application rates on the activity and diversity of denitrifying bacteria in the Broadbalk Wheat Experiment, Philos. T. R. Soc. B, 367, 1235–1244, https://doi.org/10.1098/rstb.2011.0314, 2012.
Coskun, D., Britto, D. T., Shi, W., and Kronzucker, H. J.: Nitrogen transformations in modern agriculture and the role of biological nitrification inhibition, Nat. Plants, 3, 17074, https://doi.org/10.1038/nplants.2017.74, 2017.
Csitári, G., Tóth, Z., and Kökény, M.: Effects of Organic Amendments on Soil Aggregate Stability and Microbial Biomass in a Long-Term Fertilization Experiment (IOSDV), Sustainability, 13, 9769, https://doi.org/10.3390/su13179769, 2021.
Cui, P., Fan, F., Yin, C., Song, A., Huang, P., Tang, Y., Zhu, P., Peng, C., Li, T., Wakelin, S. A., and Liang, Y.: Long-term organic and inorganic fertilization alters temperature sensitivity of potential N2O emissions and associated microbes, Soil Biol. Biochem., 93, 131–141, https://doi.org/10.1016/j.soilbio.2015.11.005, 2016.
Cvetkov, M. and Tajnšek, A.: Soil organic matter changes according to the application of organic and mineral fertilizers within long-term experiments, Acta Agriculturae Slovenica, 93, 311–320, https://doi.org/10.14720/aas.2009.93.3.15076, 2009.
Daims, H., Lebedeva, E. V., Pjevac, P., Han, P., Herbold, C., Albertsen, M., Jehmlich, N., Palatinszky, M., Vierheilig, J., Bulaev, A., Kirkegaard, R. H., Von Bergen, M., Rattei, T., Bendinger, B., Nielsen, P. H., and Wagner, M.: Complete nitrification by Nitrospira bacteria, Nature, 528, 504–509, https://doi.org/10.1038/nature16461, 2015.
Dang, D. M., Macdonald, B., Warneke, S., and White, I.: Available carbon and nitrate increase greenhouse gas emissions from soils affected by salinity, Soil Res., 55, 47–57, https://doi.org/10.1071/SR16010, 2016.
Davidson, E. A. and Kanter, D.: Inventories and scenarios of nitrous oxide emissions, Environ. Res. Lett., 9, 105012, https://doi.org/10.1088/1748-9326/9/10/105012, 2014.
DeForest, J. L. and Otuya, R. K.: Soil nitrification increases with elevated phosphorus or soil pH in an acidic mixed mesophytic deciduous forest, Soil Biol. Biochem., 142, 107716, https://doi.org/10.1016/j.soilbio.2020.107716, 2020.
Del Grosso, S. J., Parton, W. J., Mosier, A. R., Ojima, D. S., Kulmala, A. E., and Phongpan, S.: General model for N2O and N2 gas emissions from soils due to dentrification, Global Biogeochem. Cy., 14, 1045–1060, https://doi.org/10.1029/1999GB001225, 2000.
Ehleringer, J. R.: Photosynthesis and Photorespiration: Biochemistry, Physiology, and Ecological Implications, HortScience, 14, 217–222, https://doi.org/10.21273/HORTSCI.14.3.217, 1979.
Ehleringer, J. R. and Cerling, T. E.: C3 and C4 photosynthesis, edited by: Munn, T., Encyclopedia of Global Environmental Change, John Wiley & Sons, Ltd, Chichester, 186–190, 2002.
Engel, R., Liang, D. L., Wallander, R., and Bembenek, A.: Influence of urea fertiliser placement on nitrous oxide production from a silt loam soil, J. Environ. Qual., 39, 115–125, https://doi.org/10.2134/jeq2009.0130, 2010.
Escuer-Gatius, J., Lõhmus, K., Shanskiy, M., Kauer, K., Vahter, H., Mander, Ü., Astover, A., and Soosaar, K.: Critical points for closing the carbon and nitrogen budgets in a winter rapeseed field, Nutr. Cycl. Agroecosys., 122, 289–311, https://doi.org/10.1007/s10705-022-10202-8, 2022.
Espenberg, M., Truu, M., Mander, Ü., Kasak, K., Nõlvak, H., Ligi, T., Oopkaup, K., Maddison, M., and Truu, J.: Differences in microbial community structure and nitrogen cycling in natural and drained tropical peatland soils, Sci. Rep., 8, 4742, https://doi.org/10.1038/s41598-018-23032-y, 2018.
FAO: The future of food and agriculture: Trends and challenges, Food and Agriculture Organization of the United Nations, FAO, http://www.fao.org/3/a-i6583e.pdf (last access: 9 January 2024), 2017.
Frasier, I., Noellemeyer, E., Fernández, R., and Quiroga, A.: Direct field method for root biomass quantification in agroecosystems, MethodsX, 3, 513–159, https://doi.org/10.1016/j.mex.2016.08.002, 2016.
Graf, D. R. H., Jones, C. M., and Hallin, S.: Intergenomic Comparisons Highlight Modularity of the Denitrification Pathway and Underpin the Importance of Community Structure for N2O Emissions, PLoS One, 9, e114118, https://doi.org/10.1371/journal.pone.0114118, 2014.
Gu, J., Zheng, X., Wang, Y., Ding, W., Zhu, B., Chen, X., Wang, Y., Zhao, Z., Shi, Y., and Zhu, J.: Regulatory effects of soil properties on background N2O emissions from agricultural soils in China, Plant Soil, 295, 53–65, https://doi.org/10.1007/s11104-007-9260-2, 2007.
Haj-Amor, Z., Araya, T., Kim, D-G, Bouri, S., Lee, J., Ghiloufi, W., Yang, Y., Kang, H., Jhariya, M. K., Banerjee, A., and Lal, R.: Soil salinity and its associated effects on soil microorganisms, greenhouse gas emissions, crop yield, biodiversity and desertification: A review, Sci. Total Environ., 843, 156946, https://doi.org/10.1016/j.scitotenv.2022.156946, 2022.
Harter, J., Krause, H.-M., Schuettler, S., Ruser, R., Fromme, M., Scholten, T., Kappler, A., and Behrens, S.: Linking N2O emissions from biochar-amended soil to the structure and function of the N-cycling microbial community, ISME J., 8, 660–674, https://doi.org/10.1038/ismej.2013.160, 2014.
Haynes, R. J. and Francis, G. S.: Changes in microbial biomass C, soil carbohydrate composition and aggregate stability induced by growth of selected crop and forage species under field conditions, J. Soil Sci., 44, 665–675, https://doi.org/10.1111/j.1365-2389.1993.tb02331.x, 1993.
Hibberd, J. M. and Quick, W. P.: Characteristics of C4 photosynthesis in stems and petioles of C3 flowering plants, Nature, 415, 451–454, https://doi.org/10.1038/415451a, 2002.
Hijbeek, R., van Ittersum, M. K., ten Berge, H. F. M., Gort, G., Spiegel, H., and Whitmore, A. P.: Do organic inputs matter – a meta-analysis of additional yield effects for arable crops in Europe, Plant Soil, 411, 293–303, https://doi.org/10.1007/s11104-016-3031-x, 2017.
Hu, H.-W., Chen, D., and He, J.-Z.: Microbial regulation of terrestrial nitrous oxide formation: Understanding the biological pathways for prediction of emission rates, FEMS Microbiol. Rev., 39, 729–749, https://doi.org/10.1093/femsre/fuv021, 2015.
Hutchinson, G. L. and Livingston, G. P.: Use of chamber systems to measure trace gas fluxes, Asa. Spec. P., 55, 63–78, https://doi.org/10.2134/asaspecpub55.c4, 1993.
International Organization for Standardization: Determination of total nitrogen content by dry combustion (“elemental analysis”), ISO Standard No. 13878:1998, https://www.iso.org/standard/23117.html (last access: 9 January 2024), 1998.
IPCC: Climate Change 2021: The Physical Science Basis. Contribution of Working Group I to the Sixth Assessment Report of the Intergovernmental Panel on Climate Change, edited by: Masson-Delmotte, V., Zhai, P., Pirani, A., Connors, S. L., Péan, C., Berger, S., Caud, N., Chen, Y., Goldfarb, L., Gomis, M. I., Huang, M., Leitzell, K., Lonnoy, E., Matthews, J. B. R., Maycock, T. K., Waterfield, T., Yelekçi, O., Yu, R., and Zhou, B., Cambridge University Press, Cambridge, United Kingdom and New York, NY, USA, 2391 pp., https://doi.org/10.1017/9781009157896, 2021.
IUSS WG WRB: World Reference Base for Soil Resources 2014 (update 2015), IUSS Working Group WRB, International Soil Classification System for Naming Soils and Creating Legends for Soil Maps, World Soil Resources Report No. 106, Rome, Food and Agriculture Organisation of the United Nations, FAO, https://openknowledge.fao.org/server/api/core/ (last access: 18 December 2024), 2015.
Jones, C. M., Spor, A., Brennan, F. P., Breuil, M-C., Bru, D., Lemanceau, P., Griffiths, B., Hallin, S., and Philippot, L.: Recently identified microbial guild mediates soil N2O sink capacity, Nature Climate Change, 4, 801–805, https://doi.org/10.1038/nclimate2301, 2014.
Joshi, J., Stocker, B. D., Hofhansl, F., Zhou, S., Dieckmann, U., and Prentice, I. C.: Towards a unified theory of plant photosynthesis and hydraulics, Nat. Plants, 8, 1304–1316, https://doi.org/10.1038/s41477-022-01244-5, 2022.
Kaiser, E.-A. and Ruser, R.: Nitrous oxide emissions from arable soils in Germany—An evaluation of six long-term field experiments, J. Plant Nutr. Soil Sc., 163, 249–259, https://doi.org/10.1002/1522-2624(200006)163:3<249::AID-JPLN249>3.0.CO;2-Z, 2000.
Káš, M., Haberle, J., and Matějková, S.: Crop productivity under increasing nitrogen rates and different organic fertilization systems in a long-term IOSDV experiment in the Czech Republic, Arch. Agron. Soil Sci., 56, 451–461, https://doi.org/10.1080/03650340903369392, 2010.
Kassambara, A. and Mundt, F.: Factoextra: Extract and Visualise the Results of Multivariate Data Analyses. R Package Version 1.0.7, https://CRAN.R-project.org/package=factoextra (last access: 18 August 2023), 2020.
Kazmi, F. A., Espenberg, M., Pärn, J., Masta, M., Ranniku, R., Thayamkottu, S., and Mander, Ü.: Meltwater of freeze-thaw cycles drives N2O-governing microbial communities in a drained peatland forest soil, Biol. Fert. Soils, https://doi.org/10.1007/s00374-023-01790-w, online first, 2023.
Kim, D.-G., Giltrap, D., and Hernandez-Ramirez, G.: Background nitrous oxide emissions in agricultural and natural lands: A meta-analysis, Plant Soil, 373, 17–30, https://doi.org/10.1007/s11104-013-1762-5, 2013.
Koch, H., van Kessel, M. A. H. J., and Lücker, S.: Complete nitrification: Insights into the ecophysiology of comammox Nitrospira, Appl. Microbiol. Biot., 103, 177–189, https://doi.org/10.1007/s00253-018-9486-3, 2019.
Kursa, M. B. and Rudnicki, W. R.: Feature Selection with the Boruta Package, J. Stat. Softw., 36, 1–13, https://doi.org/10.18637/jss.v036.i11, 2010.
Kuypers, M. M. M., Marchant, H. K., and Kartal, B.: The microbial nitrogen-cycling network, Nat. Rev. Microbiol., 16, 263–276, https://doi.org/10.1038/nrmicro.2018.9, 2018.
Lazcano, C., Zhu-Barker, X., and Decock, C.: Effects of Organic Fertilizers on the Soil Microorganisms Responsible for N2O Emissions: A Review, Microorganisms, 9, 983, https://doi.org/10.3390/microorganisms9050983, 2021.
Lê, S., Josse, J., and Husson, F.: FactoMineR: An R Package for Multivariate Analysis, J. Stat. Softw., 25, 1–18, https://doi.org/10.18637/jss.v025.i01, 2008.
Ledvinka, H. D., Toghyani, M., Tan, D. K. Y., Khoddami, A., Godwin, I. D., and Liu, S. Y.: The Impact of Drought, Heat and Elevated Carbon Dioxide Levels on Feed Grain Quality for Poultry Production, Agriculture, 12, 1913, https://doi.org/10.3390/agriculture12111913, 2022.
Li, C., Hu, H.-W., Chen, Q.-L., Chen, D., and He, J.-Z.: Comammox Nitrospira play an active role in nitrification of agricultural soils amended with nitrogen fertilizers, Soil Biol. Biochem., 138, 107609, https://doi.org/10.1016/j.soilbio.2019.107609, 2019.
Liu, S., Wang, J. J., Tian, Z., Wang, X., and Harrison, S.: Ammonia and greenhouse gas emissions from a subtropical wheat field under different nitrogen fertilisation strategies, J. Environ. Sci., 57, 196–210, https://doi.org/10.1016/j.jes.2017.02.014, 2017.
Estonian Land Board: https://xgis.maaamet.ee/xgis2/page/app/maainfo (last access: 9 January 2024), 2023.
Mengel, K. and Kirkby, E. A.: Principles of plant nutrition, 5th edn., Kluwer Academic Publishers, the Netherlands, https://doi.org/10.1007/978-94-010-1009-2, 2001.
Nardi, P., Laanbroek, H. J., Nicol, G. W., Renella, G., Cardinale, M., Pietramellara, G., Weckwerth, W., Trinchera, A., Ghatak, A., and Nannipieri, P.: Biological nitrification inhibition in the rhizosphere: Determining interactions and impact on microbially mediated processes and potential applications, FEMS Microbiol. Rev., 44, 874–908, https://doi.org/10.1093/femsre/fuaa037, 2020.
Pandey, C. B., Kumar, U., Kaviraj, M., Minick, K. J., Mishra, A. K., and Singh, J. S.: DNRA: A short-circuit in biological N-cycling to conserve nitrogen in terrestrial ecosystems, Sci. Total Environ., 738, 139710, https://doi.org/10.1016/j.scitotenv.2020.139710, 2020.
Pandey, R. K., Maranville, J. W., and Bako, Y.: Nitrogen Fertilizer Response and Use Efficiency for Three Cereal Crops in Niger, Commun. Soil Sci. Plan., 32, 1465–1482, https://doi.org/10.1081/CSS-100104206, 2001.
Parton, W. J., Holland, E. A., Del Grosso, S. J., Hartman, M. D., Martin, R. E., Mosier, A. R., Ojima, D. S., and Schimel, D. S.: Generalized model for NOx and N2O emissions from soils, J. Geophys. Res.-Atmos., 106, 17403–17419, https://doi.org/10.1029/2001JD900101, 2001.
Paustian, K., Lehmann, J., Ogle, S., Reay, D., Robertson, G. P., and Smith, P.: Climatesmart soils, Nature, 532, 49–57, https://doi.org/10.1038/nature17174, 2016.
Philippot, L., Hallin, S., and Schloter, M.: Ecology of Denitrifying Prokaryotes in Agricultural Soil, Adv. Agron., 96, 249–305, https://doi.org/10.1016/S0065-2113(07)96003-4, 2007.
Poole, C. F.: Ionization-based detectors for gas chromatography, J. Chromatogr. A, 1421, 137–153, https://doi.org/10.1016/j.chroma.2015.02.061, 2015.
Putz, M., Schleusner, P., Rütting, T., and Hallin, S.: Relative abundance of denitrifying and DNRA bacteria and their activity determine nitrogen retention or loss in agricultural soil, Soil Biol. Biochem., 123, 97–104, https://doi.org/10.1016/j.soilbio.2018.05.006, 2018.
Ravishankara, A. R., Daniel, J. S., and Portmann, R. W.: Nitrous Oxide (N2O): The Dominant Ozone-Depleting Substance Emitted in the 21st Century, Science, 326, 123–125, https://doi.org/10.1126/science.1176985, 2009.
Rayne, N. and Aula, L.: Livestock Manure and the Impacts on Soil Health: A Review, Soil Syst., 4, 64, https://doi.org/10.3390/soilsystems4040064, 2020.
Republic of Estonia Environment Agency: Meteorological Yearbook of Estonia 2022, https://kaur.maps.arcgis.com/sharing/rest/content/items/1c8b8f59e7184619b4455b2beceacd6a/data (last access: 18 August 2023), 2023.
Robertson, G. P. and Vitousek, P. M.: Nitrogen in Agriculture: Balancing the Cost of an Essential Resource, Annu. Rev. Env. Resour., 34, 97–125, https://doi.org/10.1146/annurev.environ.032108.105046, 2009.
Rütting, T., Boeckx, P., Müller, C., and Klemedtsson, L.: Assessment of the importance of dissimilatory nitrate reduction to ammonium for the terrestrial nitrogen cycle, Biogeosciences, 8, 1779–1791, https://doi.org/10.5194/bg-8-1779-2011, 2011.
Sainju, U. M.: Determination of nitrogen balance in agroecosystems, MethodsX, 4, 199–208, https://doi.org/10.1016/j.mex.2017.06.001, 2017.
Saud, S., Wang, D., and Fahad, S.: Improved Nitrogen Use Efficiency and Greenhouse Gas Emissions in Agricultural Soils as Producers of Biological Nitrification Inhibitors, Front. Plant Sci., 13, 854195, https://doi.org/10.3389/fpls.2022.854195, 2022.
Schaffasz, A., Windpassinger, S., Friedt, W., Snowdon, R., and Wittkop, B.: Sorghum as a Novel Crop for Central Europe: Using a Broad Diversity Set to Dissect Temperate-Adaptation, Agronomy, 9, 535, https://doi.org/10.3390/agronomy9090535, 2019.
Schimel, J. P.: Life in Dry Soils: Effects of Drought on Soil Microbial Communities and Processes, Annu. Rev. Ecol. Evol. S., 49, 409–432, https://doi.org/10.1146/annurev-ecolsys-110617-062614, 2018.
Serret, M. D., Ortiz-Monasterio, I., Pardo, A., and Araus, J. L.: The effects of urea fertilisation and genotype on yield, nitrogen use efficiency, δ15N and δ13C in wheat, Ann. Appl. Biol., 153, 243–257, https://doi.org/10.1111/j.1744-7348.2008.00259.x, 2008.
Shcherbak, I., Millar, N., and Robertson, G. P.: Global metaanalysis of the nonlinear response of soil nitrous oxide (N2O) emissions to fertilizer nitrogen, P. Natl. Acad. Sci. USA, 111, 9199–9204, https://doi.org/10.1073/pnas.1322434111, 2014.
Shen, H., Shiratori, Y., Ohta, S., Masuda, Y., Isobe, K., and Senoo, K.: Mitigating N2O emissions from agricultural soils with fungivorous mites, ISME J., 15, 8, https://doi.org/10.1038/s41396-021-00948-4, 2021.
Sosulski, T., Szara, E., Stępien, W., and Szymańska, M.: Nitrous oxide emissions from the soil under different fertilization systems on a long-term experiment, Plant Soil Environ., 60, 481–488, https://doi.org/10.17221/943/2013-PSE, 2014.
Spiegel, H., Dersch, G., Baumgarten, A., and Hösch, J.: The International Organic Nitrogen Long-term Fertilisation Experiment (IOSDV) at Vienna after 21 years, Arch. Agron. Soil Sci., 56, 405–420, https://doi.org/10.1080/03650341003645624, 2010.
Stevens, N., Bond, W., Feurdean, A., and Lehmann, C. E. R.: Grassy Ecosystems in the Anthropocene, Annu. Rev. Environ. Res., 47, 261–289, https://doi.org/10.1146/annurev-environ-112420-015211, 2022.
Stremińska, M. A., Felgate, H., Rowley, G., Richardson, D. J., and Baggs, E. M.: Nitrous oxide production in soil isolates of nitrate-ammonifying bacteria, Env. Microbiol. Rep., 4, 66–71, https://doi.org/10.1111/j.1758-2229.2011.00302.x, 2012.
Tajnšek, A., Čergan, Z., and Čeh, B.: Results of the long-term field experiment IOSDV Jable at the beginning of the 21st century, Arch. Agron. Soil Sci., 59, 1099–1108, https://doi.org/10.1080/03650340.2012.697996, 2013.
Tang, Y., Yu, G., Zhang, X., Wang, Q., Ge, J., and Liu, S.: Changes in nitrogen-cycling microbial communities with depth in temperate and subtropical forest soils, Appl. Soil Ecol., 124, 218–228, https://doi.org/10.1016/j.apsoil.2017.10.029, 2018.
Thapa, R., Chatterjee, A., Wick, A., and Butcher, K.: Carbon Dioxide and Nitrous Oxide Emissions from Naturally Occurring Sulfate-Based Saline Soils at Different Moisture Contents, Pedosphere, 27, 868–876, https://doi.org/10.1016/S1002-0160(17)60453-3, 2017.
Thomson, A. J., Giannopoulos, G., Pretty, J., Baggs, E. M., and Richardson, D. J.: Biological sources and sinks of nitrous oxide and strategies to mitigate emissions, Philos. T. R. Soc. B, 367, 1157–1168, https://doi.org/10.1098/rstb.2011.0415, 2012.
Tian, X., Engel, B. A., Qian, H., Hua, E., Sun, S., and Wang, Y.: Will reaching the maximum achievable yield potential meet future global food demand?, J. Clean. Prod., 294, 126285, https://doi.org/10.1016/j.jclepro.2021.126285, 2021.
Tilman, D., Balzer, C., Hill, J., and Befort, B. L.: Global food demand and the sustainable intensification of agriculture, P. Natl. Acad. Sci. USA, 108, 20260–20264, https://doi.org/10.1073/pnas.1116437108, 2011.
Van Kessel, M. A. H. J., Speth, D. R., Albertsen, M., Nielsen, P. H., Op Den Camp, H. J. M., Kartal, B., Jetten, M. S. M., and Lücker, S.: Complete nitrification by a single microorganism, Nature, 528, 555–559, https://doi.org/10.1038/nature16459, 2015.
Van Reeuwijk, L. P.: Procedures for soil analysis, Technical Paper No. 9, 6th Edn., FAO/ISRIC, Wageningen, the Netherlands, 120 pp., 2002.
Wan, Z., Wang, L., Huang, G., Rasul, F., Awan, M. I., Cui, H., Liu, K., Yu, X., Tang, H., Wang, S., and Xu, H.: NirS and nosZII bacterial denitrifiers as well as fungal denitrifiers are coupled with N2O emissions in long-term fertilized soils, Sci. Total Environ., 897, 165426, https://doi.org/10.1016/j.scitotenv.2023.165426, 2023.
Whetton, R. L., Harty, M. A., and Holden, N. M.: Communicating Nitrogen Loss Mechanisms for Improving Nitrogen Use Efficiency Management, Focused on Global Wheat, Nitrogen, 3, 213–246, https://doi.org/10.3390/nitrogen3020016, 2022.
Yamulki, S., Goulding, K. W. T., Webster, C. P., and Harrison, R. M.: Studies on no and N2O fluxes from a wheat field, Atmos. Environ., 29, 1627–1635, https://doi.org/10.1016/1352-2310(95)00059-8, 1995.
Yao, Z., Zhou, Z., Zheng, X., Xie, B., Mei, B., Wang, R., Butterbach-Bahl, K., and Zhu, J.: Effects of organic matter incorporation on nitrous oxide emissions from rice-wheat rotation ecosystems in China, Plant Soil, 327, 315–330, https://doi.org/10.1007/s11104-009-0056-4, 2009.
Yuan, J., Yan, L., Li, G., Sadiq, M., Rahim, N., Wu, J., Ma, W., Xu, G., and Du, M.: Effects of conservation tillage strategies on soil physicochemical indicators and N2O emission under spring wheat monocropping system conditions, Sci. Rep., 12, 7066, https://doi.org/10.1038/s41598-022-11391-6, 2022.
Zaman, M., Nguyen, M. L., Šimek, M., Nawaz, S., Khan, M. J., Babar, M. N., and Zaman, S.: Emissions of Nitrous Oxide (N2O) and Di-Nitrogen (N2) from the Agricultural Landscapes, Sources, Sinks, and Factors Affecting N2O and N2 Ratios, in: Greenhouse Gases-Emission, Measurement and Management, editedby: Liu, G., InTech Europe: Rijeka, Croatia, https://doi.org/10.5772/32781, 2012.
Zhang, Q., Wu, Z., Zhang, X., Duan, P., Shen, H., Gunina, A., Yan, X., and Xiong, Z.: Biochar amendment mitigated N2O emissions from paddy field during the wheat growing season, Environmental Pollution, 281, 117026, https://doi.org/10.1016/j.envpol.2021.117026, 2021.
Zhou, M., Zhu, B., Brüggemann, N., Dannenmann, M., Wang, Y., and Butterbach-Bahl, K.: Sustaining crop productivity while reducing environmental nitrogen losses in the subtropical wheat-maize cropping systems: A comprehensive case study of nitrogen cycling and balance, Agr. Ecosyst. Environ., 231, 1–14, https://doi.org/10.1016/j.agee.2016.06.022, 2016.
Zhu, W., Wang, C., Hill, J., He, Y., Tao, B., Mao, Z., and Wu, Q.: A missing link in the estuarine nitrogen cycle?: Coupled nitrification-denitrification mediated by suspended particulate matter, Sci. Rep., 8, 2282, https://doi.org/10.1038/s41598-018-20688-4, 2018.