the Creative Commons Attribution 4.0 License.
the Creative Commons Attribution 4.0 License.
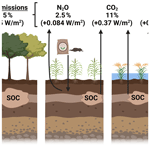
Soil is a major contributor to global greenhouse gas emissions and climate change
Ram C. Dalal
Brigid A. McKenna
Pete Smith
Peng Wang
Zhe Weng
Frederik J. T. van der Bom
Neal W. Menzies
It is unequivocal that human activities have increased emissions of greenhouse gases, that this is causing warming, and that these changes will be irreversible for centuries to millennia. Whilst previous studies have broadly examined the contribution of agriculture or land use change to anthropogenic greenhouse gas emissions, the contribution of soil itself remains unclear, with quantifying the contribution of soil in this regard being critical for developing and implementing appropriate management practices. In the present study, we used previously published datasets for carbon dioxide, nitrous oxide, and methane to determine soil-based emissions of greenhouse gases and their contribution to anthropogenic greenhouse gas emissions. We show that our near-complete reliance on soil to produce the rapidly increasing quantities of food being demanded by humans has caused soil to release profound amounts of greenhouse gases that are threatening the future climate. Indeed, net anthropogenic emissions from soil alone account for 15 % of the entire global increase in climate warming (radiative forcing) caused by well-mixed greenhouse gases, with carbon dioxide being the most important gas emitted from soil (74 % of total soil-derived warming), followed by nitrous oxide (17 %) and methane (9 %). There is an urgent need to prevent further land use change (including for biofuel production) to limit the release of carbon dioxide that results from the loss of soil organic carbon, to develop strategies to increase nitrogen fertilizer efficiency in order to reduce nitrous oxide emissions, to decrease methane from rice paddies, and to ensure that the widespread thawing of permafrost is avoided. Innovative approaches are urgently required for reducing greenhouse gas emissions from soil if we are to limit global warming to 1.5 or 2.0 °C.
- Article
(2376 KB) - Full-text XML
- BibTeX
- EndNote
Soil is multifunctional and provides a diverse range of services. One important role of soil is the production of 98.8 % of the calories consumed by humans – 12.2 % (1556 ×106 ha) of global ice-free land is used for cropping, and 24.8 % is used for grazing (FAO, 2021). Given that the vast majority of human food comes from soil, profound changes in land use over the history of agrarian society have greatly increased stresses on soil (Kopittke et al., 2019). The ongoing increasing demand for food is due to both a rapidly increasing population, having grown from 2.5 billion in 1950 to 7.8 billion in 2020 (projected to be 9.8 billion by 2050), and increasing rates of consumption per capita. There are also other demands on soil, including land use for bioenergy production, with land devoted to biofuel production increasing from 7 ×106 ha in 2000 to 32 ×106 ha in 2010 (Langeveld et al., 2013).
The reliance of humans on soil is causing the substantial release of anthropogenic greenhouse gases, especially carbon dioxide (CO2), nitrous oxide (N2O), and methane (CH4), contributing markedly to climate change. Climate change is the greatest challenge facing human society, and it is “unequivocal that human influence has warmed the atmosphere, ocean, and land” and that many of the resulting changes will be “irreversible for centuries to millennia” (IPCC, 2021). Thus, our need to rapidly increase food production from soil while simultaneously decreasing the greenhouse gas emissions associated with this production represents a “wicked problem” (Rittel and Webber, 1973). If we continue to focus solely on the role of soil in providing humans with food without recognizing, and acting upon, its profound contributions to greenhouse gas emissions and climate change, we will threaten the hospitability of our planet for millennia and will fail to recognize intergenerational equality.
Soil acts as both a source and a sink for natural and anthropogenic greenhouse gases. For example, for C, the net global input of C to soil from vegetation is ca. 61 Pg C yr−1, with a similar amount lost from soil to the atmosphere as CO2 (Lehmann and Kleber, 2015). However, the anthropogenic use of soil and a changing climate have altered this natural balance. For example, it is known that the boreal and temperate forests of the Northern Hemisphere are making an increased contribution to the terrestrial (vegetation plus soil) C sink (Canadell et al., 2021), with these systems having increased biomass production due to CO2 fertilization and lengthening growing seasons. Nevertheless, it is also known that, globally, soil contains ca. 116 Pg less C now than prior to land use change (Sanderman et al., 2017), indicating that, despite these localized regions of increased C sequestration in soil, there has been an overall net global decrease in global C stocks and, hence, a net release of CO2 to the atmosphere. In a similar manner, soil is both a source and a sink for CH4 – soil acts as a sink for ca. 30 Tg CH4 yr−1, with this representing ca. 4 % of the total CH4 emissions in 2017 (Saunois et al., 2020). However, soil is also both a natural and anthropogenic source of atmospheric CH4 – the use of soil for rice cultivation, for example, also accounts for 30 Tg CH4 yr−1 (Saunois et al., 2020). Thus, despite the ongoing critical role of soil as a sink for greenhouse gases, it is also imperative to quantify how the anthropogenic use of soil has also increased atmospheric emissions of greenhouse gases from soil. This is because the net anthropogenic increase in emissions from soil, together with emissions of greenhouse gases from other sources such as the burning of fossil fuels, also contributes to global warming and climate change.
The aim of the present study was to quantify the contribution of soil to global anthropogenic greenhouse gas emissions and global climate change. To the best of our knowledge, no studies have reported this information previously for soil. Although Oertel et al. (2016) examined the rate at which greenhouse gases evolve from soil, these authors did not consider the overall net contribution of soil to climate change, while other studies have examined the contribution of agriculture more broadly (Robertson et al., 2000; Jia et al., 2019; Amundson, 2022). In a similar manner, previous studies have examined the contributions of agriculture, forestry, and other land use (AOLU) to greenhouse gas emissions. However, in order to improve management practices and to inform better decision-making processes, it is imperative that we quantify the precise sources of greenhouse gases and understand the factors causing their emissions. In this regard, here, we differentiate between “soil” and “land”, with soil being a core nested component of land, while land has a broader context consisting of soil, rocks, rivers, and vegetation (Koch et al., 2013) – it is necessary to distinguish between soil and land to understand, value, and manage soil as a discrete component of the broader landscape. Our work also complements the increasing number of studies that examine the potential of soil as a nature-based solution to CO2 removal and climate change mitigation (Smith, 2012; Paustian et al., 2016; Minasny et al., 2017; Lal et al., 2021; van Vuuren et al., 2018; Crow and Sierra, 2022). We first need to accurately quantify the substantial quantities of anthropogenic greenhouse gas emissions from soil and their contribution to climate change before we can properly estimate the potential of soil in mitigating greenhouse gas emissions. We show that soil is a major contributor to global greenhouse gas emissions and that there is a need to urgently the improve management of soil if we are to simultaneously increase food production while also limiting global climate change.
This study examined anthropogenic, soil-based emissions of greenhouse gases and their contribution to climate change. All underlying data used here were derived from previous studies (see later). For all three greenhouse gases, we examined the contribution of soil to emissions using two broad approaches. The first was to examine how the current annual net anthropogenic flux from soil compares to the total anthropogenic flux from all sources to determine the current soil-derived contributions to current greenhouse gas emissions. The second approach was to calculate the contribution of soil-based emissions to the current increase in effective radiative forcing due to anthropogenic greenhouse gases, with the increase in effective radiative forcing being due not only to current fluxes but also to historical emissions. Currently, the total increase in effective radiative forcing due to increased concentrations of well-mixed greenhouse gases (CO2, CH4, N2O, and halocarbons) is +3.32 W m−2, of which +2.16 W m−2 is due to CO2, +0.21 W m−2 is due to N2O, and +0.54 W m−2 is due to CH4 (Forster et al., 2021). The overall net increase in effective radiative forcing when taking into account all climate forcers, including those which decrease effective radiative forcing such as aerosol–cloud interactions, is +2.84 W m−2. (Forster et al., 2021). For each of these three greenhouse gases, we calculate the total anthropogenic contribution of soil to the current increase in radiative forcing by using historical data. For this, we determine the proportion of total anthropogenic emissions that have been derived from soil over time while also taking into account the atmospheric life of the gas, with this being used to calculate the proportion of the current increase in anthropogenic radiative forcing that is due to soil. For each of the three gases, the length of time over which anthropogenic emissions from soil were determined, as well as the data intensity over that period, depended upon the data sources that were available (see below).
2.1 Carbon dioxide
For CO2, to determine the anthropogenic soil-derived contributions to greenhouse gas emissions, we used the data of Sanderman et al. (2017), who modeled spatial changes in soil organic carbon (SOC) stocks over time due to agriculture. By comparing changes in total global SOC stocks as opposed to changes in net inputs or outputs from soil, we disentangle multiple confounding factors – if the global SOC stock is a given quantity lower (or higher) than the corresponding value prior to land use change, it is unambiguous that this net mass of C must have been lost to (or sequestered from) the global atmosphere due to anthropogenic use of soil despite any potential increase in SOC sequestration rates in soils of particular areas where they are acting as a net sink. Sanderman et al. (2017) used a machine-learning-based data-driven statistical model based upon soil profile observations, with this being coupled with the History Database of the Global Environment (HYDE) (Sanderman et al., 2017).
Using the study of Sanderman et al. (2017), we used the values reported for the cumulative loss of SOC over time (Pg C, Fig. 2 of Sanderman et al., 2017) to calculate the rate of the net decrease in SOC stocks (Pg C yr−1) and the associated net emission of CO2 (i.e., the first of the two approaches articulated above): the most recent data point of Sanderman et al. (2017) was used to determine the current annual net anthropogenic flux from soil, while the entire data set (Fig. 2 of Sanderman et al., 2017) was used to determine the total (historical) anthropogenic contribution of soil. In addition, this historical assessment of the total anthropogenic contribution of soil to the current increase in radiative forcing (i.e., our second approach articulated above) requires consideration of the atmospheric life of the gas. However, given that CO2 is chemically inert in the atmosphere, there is no single value for the atmospheric life of CO2, but some of the CO2 emitted by humans remains in the atmosphere for millennia (Forster et al., 2021). Rather, we simply determine the proportion of the cumulative anthropogenic emissions of CO2 from all sources that were due to anthropogenic emissions from soil (being from land use change and loss of SOC, as discussed later). In other words, to calculate the contribution of soil-based emissions to the current increase in effective radiative forcing due to anthropogenic greenhouse gases, we simply calculated the proportion of total historical anthropogenic CO2 emissions that have been derived from soil by determining the total cumulative net anthropogenic emissions from soil (Sanderman et al., 2017) using total cumulative anthropogenic emissions (Friedlingstein et al., 2023). Because we do not use a value for the atmospheric life for CO2, we therefore assume that historical anthropogenic emissions of CO2 from soil contribute equally to increases in radiative forcing compared to the more recent emissions of CO2 from fossil sources. In this regard, it must be noted that, although 57 % of the CO2 emitted into the atmosphere is absorbed by the ocean sink and the terrestrial sink (26 % being absorbed by the ocean sink and 31 % being absorbed by the terrestrial sink) (Friedlingstein et al., 2023), we have assumed that the proportion of CO2 absorbed by sinks is constant for both the soil-based source and fossil sources despite the soil-based source occurring over a longer period of time, with this being likely to cause an overestimation of the contribution of CO2 emissions from soil to the current increase in radiative forcing. Indeed, as discussed later, emissions from soil have increased rapidly during in the last ca. 100–200 years, while, in contrast, emissions from fossil sources have occurred primarily during the last ca. 60 to 70 years. Regardless, even for C that is absorbed by the ocean sink, although it does not remain in the atmosphere, where it contributes to climate change, it causes ocean acidification, which (like climate change) is also considered to be a critical Earth system process (Steffen et al., 2015).
2.2 Nitrous oxide
For N2O, we took a slightly different approach than that used for CO2 where we examined the changes in global SOC stocks. Rather, for N2O, we determined the proportion of total anthropogenic emissions that were due to anthropogenic emissions from soil. This provides data on the proportion of anthropogenic N2O being released to the atmosphere that is due to human use of soil. For this, we used the data available from Tian et al. (2019) and Tian et al. (2020), with these authors using process-based models that consider C, N, and water cycling to simulate soil N2O emissions. For N2O, we took into account the atmospheric life of N2O (the time to decrease to a concentration of , being 109 years; see Forster et al., 2021). Although the atmospheric life of N2O is 109 years, we are only able to calculate the proportion of total anthropogenic N2O emissions that have resulted from soil from the 1860s onwards as we are unaware of data examining emissions from soil prior to this point. Nevertheless, N2O emissions were low prior to the 1860s (Syakila and Kroeze, 2011), with the majority of N2O emissions being associated with the application of reactive N fertilizers, the usage of which increased profoundly from the 1950s and 1960s onwards (Erisman et al., 2008).
Table 1Anthropogenic, soil-based emissions of greenhouse gases and their contribution to the current total increase in effective radiative forcing due to increased concentrations of well-mixed greenhouse gases.

∗ Calculated from the proportion of total anthropogenic emissions that have been derived from soil over time while taking into account the atmospheric life of the gas, with this being used to calculate the proportion of the current increase in anthropogenic radiative forcing that is due to soil.
2.3 Methane
For CH4, we used a similar approach as for N2O – we examined the proportion of the anthropogenic CH4 emissions that have resulted from the anthropogenic use of soil as rice paddies. This does not neglect the simultaneous role of soil as a sink for CH4, but it determines the magnitude of the increase in atmospheric CH4 due to human use of soil. Given that the atmospheric life of CH4 is 11.8 years (Forster et al., 2021), we only examined CH4 emissions from 1980 onwards. We are unaware of data examining historical CH4 emissions from rice paddies. Therefore, given that current emissions of CH4 are 30 Tg yr−1 (see Saunois et al., 2020) from the 162 ×106 ha of rice paddies (FAO, 2021), to estimate historical emissions from rice paddies, we assumed that the rate of release per hectare was constant and simply adjusted emissions based on the area of rice paddies (FAO, 2021). These values for CH4 from rice paddy soil were compared to corresponding values for total anthropogenic CH4 emissions since 1980, as reported by Saunois et al. (2020) and He et al. (2020).
3.1 Carbon dioxide
Atmospheric concentrations of CO2 increased from 278 ppm in 1750 to 419 ppm in 2023, with concentrations having increased from 391 ppm in 2011 to 419 ppm in 2023 alone (Gulev et al., 2021; Friedlingstein et al., 2023). Given that the total increase in radiative forcing due to anthropogenic increases in well-mixed greenhouse gas concentrations is +3.32 W m−2, the fact that CO2 accounts for +2.16 W m−2 of this increase (Forster et al., 2021) makes CO2 the most important anthropogenic greenhouse gas, accounting for 65 % of the total increase in radiative forcing due to well-mixed greenhouse gases.
Soil is a critical reservoir of organic C (OC), storing ca. 3012 Pg of OC within the surface 2 m and ca. 1824 Pg OC in the surface 1 m (Sanderman et al., 2017). Indeed, this OC stored within soil exceeds the amount of C in the atmosphere (879 Pg) and in vegetation (600 Pg C) combined and is ca. 300 times greater than current annual emissions of C from fossil sources (9.9 Pg C; Friedlingstein et al., 2023). Importantly, not only is the total soil organic carbon (SOC) stock large, but it is also highly dynamic – each year, ca. 61 Pg of C enters soil from vegetation, while a similar amount is lost from soil to the atmosphere (almost entirely as CO2) due to mineralization of the SOC (Lehmann and Kleber, 2015). As a result, ca. 7 % of the atmospheric C pool is cycled through soil via photosynthesis every year. Due to this dynamic nature of SOC, long-term disturbances to the soil can profoundly decrease global SOC stocks. In this regard, global meta-analyses have shown that long-term cropping can reduce soil OC stocks by 30 %–60 % (Kopittke et al., 2017; Murty et al., 2002; Guo and Gifford, 2002), mainly due to lower C inputs into the soil but also due to an increase in C outputs (as both CO2 efflux and outputs of biomass in the harvested product). Given the extent of global land use change (primarily for agriculture), this loss of SOC stocks is a major global source of CO2.
For any given point in time, the net global flux of CO2 from soil is related to the rate of land use change. Prior to the year 1800, the rate of land use change was comparatively low; hence, losses of SOC during this time are also estimated to be low: < 0.05 Pg C yr−1 (50 Tg C yr−1) (Fig. 1) (Sanderman et al., 2017). However, between 1800 and 1950, rates of land use change increased ca. 15-fold (Klein Goldewijk et al., 2017), and, as a result, losses of SOC also increased from < 0.05 to > 0.3 Pg C yr−1 (equivalent to > 1.1 Pg CO2 yr−1) (Sanderman et al., 2017). Since the 1950s, rates of land use change have decreased substantially, with the associated SOC loss also decreasing to ca. 0.1 Pg C yr−1 between 1980 and 2000 (Sanderman et al., 2017). Thus, it is estimated that the current net global flux of CO2 from soil due to SOC loss is 0.1 Pg C yr−1 (Sanderman et al., 2017). In this regard, the current net global flux of CO2 from soil is greatly surpassed by fossil CO2 emissions, being 9.9 Pg C yr−1 (Friedlingstein et al., 2023). Indeed, with total emissions of 11.1 Pg C in 2022 and assuming that SOC losses are ca. 0.1 Pg C yr−1 (equivalent to 0.37 Pg CO2 yr−1, Fig. 1), the current contribution of SOC loss from land use change accounts for only ca. 0.9 % of the current annual CO2 emissions (Table 1). This is because, although the majority of land use change (measured by area) has occurred over a period of a couple hundred years and is currently decreasing, fossil CO2 emissions have largely occurred during the last half-century and continue to increase rapidly.
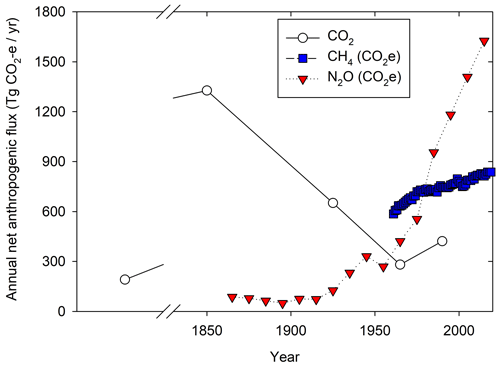
Figure 1Annual net anthropogenic fluxes of carbon dioxide (CO2), nitrous oxide (N2O), and methane (CH4) from soil expressed on a CO2-equivalent basis based on their global warming potentials for a 100-year time horizon. Data points are plotted in the middle of the measurement periods (for example, the average annual emission from the period 1800–1900 is plotted at 1850). Data for CO2 are from Sanderman et al. (2017), data for N2O are from Tian et al. (2019), and data for CH4 are from Saunois et al. (2020).
Next, we calculate the contribution of soil-based CO2 emissions to the currently observed increase in warming (radiative forcing) by determining the proportion of cumulative global C emissions that are from soil. In this regard, Sanderman et al. (2017) estimate that the total cumulative loss of SOC due to land use change, together with the associated release of CO2, is 116 Pg of C (425 Pg of CO2e), with similar values also reported by Lal (2018). In comparison, the total, cumulative, anthropogenic CO2 release from all sources in the period 1850–2022 is estimated to be 695 Pg of C (ca. 2600 Pg of CO2e) (Friedlingstein et al., 2023). Thus, we estimate that the net loss of SOC due to land use change accounts for ca. 17 % of total cumulative anthropogenic CO2 emissions (i.e., 116 Pg of C from the total emissions of 695 Pg of C). We note that this value is likely to be a slight overestimation given that it accounts for total historical SOC losses but only total anthropogenic CO2 emissions since 1850. Given that the total increase in radiative forcing due to increases in the well-mixed greenhouse gas concentrations is +3.32 W m−2, of which CO2 accounts for +2.16 W m−2 (65 % of the total), the release of CO2 due to the loss of SOC from land use change is estimated to account for 11 % (+0.37 W m−2, i.e., 17 % of +2.16 W m−2) of the total increase in radiative forcing due to anthropogenic increases in well-mixed greenhouse gases (Fig. 2, Table 1).
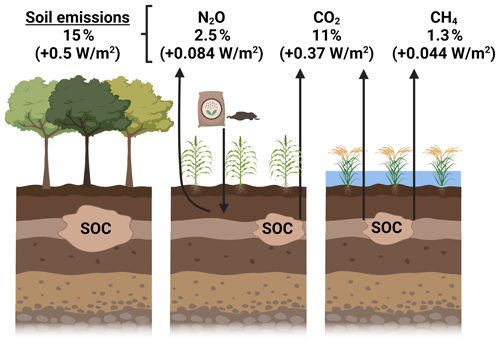
Figure 2Anthropogenic emissions of greenhouse gases (carbon dioxide (CO2), nitrous oxide (N2O), and methane (CH4)) from soil and their contribution (15 %, +0.5 W m−2) to the overall increase in warming (radiative forcing) due to well-mixed greenhouse gases (+3.32 W m−2). The vegetation on the left indicates native vegetation, a crop is shown in the center, and a rice paddy is shown on the right.
3.2 Nitrous oxide
Nitrous oxide is a greenhouse gas with a global warming potential for a 100-year time horizon that is 273 times higher than that of CO2 (Forster et al., 2021). Atmospheric concentrations of N2O increased from a concentration of 270 ppb in 1750 to a concentration of 324 ppb in 2011, increasing by a further 2.4 % to 332 ppb in 2019 (Gulev et al., 2021). Of the total increase in radiative forcing due to anthropogenic release of greenhouse gases (+3.32 W m−2), +0.21 W m−2 is due to N2O, representing 6.3 % of the total increase in radiative forcing (Forster et al., 2021).
Soil is an important source of anthropogenic N2O emissions due to the increased application of reactive N (especially as inorganic N fertilizers and animal manures) and through the increased use of leguminous crops. As with CO2 and CH4, the production of N2O in soil is a natural process, but human activities have accelerated the rate of production. Total anthropogenic emissions of N2O are estimated to be 7.3Tg N2O-N yr−1 for 2007–2016 (Tian et al., 2020), of which anthropogenic emissions from soil account for 3.7Tg N2O-N yr−1 (5.8 Tg N2O yr−1 or 1600 Tg CO2-e yr−1) (Fig. 1) (Tian et al., 2019). Thus, soil accounts for 51 % of the current anthropogenic N2O flux (Table 1). Of these anthropogenic N2O emissions from soil, croplands are of the greatest concern, accounting for 82 % of the soil-based increase resulting from the application of reactive N fertilizers (2.0Tg N2O-N yr−1), the application of manures to soil (0.6Tg N2O-N yr−1), and enhanced atmospheric N deposition to soil (0.9Tg N2O-N yr−1) (Tian et al., 2019). There has also been considerable temporal variability in the anthropogenic flux of N2O from soil, increasing from ca. 0.2Tg N2O-N yr−1 (87 Tg CO2-e yr−1) in the 1860s to ca. 1Tg N2O-N yr−1 (420 Tg CO2-e yr−1) in the 1960s before then accelerating rapidly to the current value of 3.7Tg N2O-N yr−1 (1600 Tg CO2-e yr−1, Fig. 1, Table 1).
Over the period for which calculations are possible (1860s onwards) and using the second approach outlined in the “Materials and methods” section, we calculate that 40 % of the anthropogenic N2O currently in the atmosphere results from soil-based emissions considering the fact that the atmospheric life of N2O is 109 years. With N2O accounting for 6.3 % of the total anthropogenic increase in radiative forcing and with soil accounting for 40 % of anthropogenic N2O currently in the atmosphere, we estimate that N2O emissions from soil account for 2.5 % (+0.084 W m−2) of the total anthropogenic increase in radiative forcing due to the well-mixed greenhouse gas concentrations (+3.32 W m−2) (Fig. 2).
3.3 Methane
Methane is an important greenhouse gas with a global warming potential for a 100-year time horizon that is 27.9 times higher than that of CO2 (Forster et al., 2021). From 2011 to 2019 alone, atmospheric concentrations of CH4 increased by 3.5 % from 1800 to 1866 ppb from an estimated concentration of 730 ppb in 1750 (Gulev et al., 2021). Of the total increase in radiative forcing due to the anthropogenic release of well-mixed greenhouse gases (+3.32 W m−2), CH4 accounts for +0.54 W m−2, being 16 % of the total increase (Forster et al., 2021).
Soil contributes to CH4 emissions primarily when waterlogged (Jiang et al., 2019). The release of CH4 from soil occurs due to biogenic processes, being due to the anaerobic decomposition of organic matter. This release of CH4 from waterlogged soil occurs both naturally (wetlands and swamps) and due to the anthropogenic use of soil. For these anthropogenic CH4 emissions, flooded rice paddies are almost entirely responsible, with rice paddies flooded to control weeds and to improve yields. Rice forms a staple food for much of the global population, with rice paddies accounting for 162 ×106 ha of land and with rice providing an average of 18.0 % of all calories consumed by humans (FAO, 2021).
Total global CH4 emissions are estimated to be 576 Tg CH4 yr−1, of which 359 Tg CH4 yr−1 is from anthropogenic sources, being 60 % of the total (Saunois et al., 2020). Considering only soil-based sources, for natural emissions of CH4, wetlands and swamps account for 148 Tg CH4 yr−1, being 26 % of the total global CH4 emissions and ca. 40 % of natural sources (Saunois et al., 2020). However, for anthropogenic soil-based emissions, rice paddies are critically important, accounting for 30 Tg CH4 yr−1 (Fig. 1) (Saunois et al., 2020). Thus, for current anthropogenic fluxes of 359 Tg CH4 yr−1, soil in rice paddies accounts for 8 % (30 Tg CH4 yr−1, being 840 Tg CO2-e yr−1) of the total anthropogenic emissions of CH4 (Table 1) (Saunois et al., 2020).
Based upon calculations from 1980 and using the second approach outlined in the “Materials and methods” section, we calculate that 8.2 % of the anthropogenic CH4 currently in the atmosphere results from soil-based emissions, considering the atmospheric life of CH4 (11.8 years). Given that CH4 accounts for 16 % of the total anthropogenic increase in radiative forcing (above) and given that soil accounts for 8.2 % of the anthropogenic CH4 currently in the atmosphere, we estimate that CH4 emissions from soil account for 1.3 % (+0.044 W m−2) of the total anthropogenic increase in radiative forcing due to elevated greenhouse gas concentrations (+3.32 W m−2) (Fig. 2, Table 1).
3.4 Discussion
Soil makes a substantial contribution to net anthropogenic emissions of CO2, N2O, and CH4, both historically and currently (Figs. 1 and 2, Table 1). We highlight the legacy effect of historical and current human activities involving soil on climate change. Emission of CO2 from soil alone accounts for 11 % (+0.37 W m−2) of the total increase in global warming (radiative forcing) due to well-mixed greenhouse gases, with N2O also accounting for 2.5 % (+0.084 W m−2) and with CH4 accounting for 1.3 % (+0.044 W m−2). Thus, we estimate that the anthropogenic use of soil accounts for 15 % (+0.5 W m−2) of the total increase in warming (radiative forcing) due to anthropogenic emissions of the well-mixed greenhouse gases, with CO2 therefore accounting for 74 % of this soil-based increase, N2O accounting for 17 %, and CH4 accounting for 8.9 % (Fig. 2). However, there has been substantial temporal variation – for centuries, CO2 dominated net fluxes of anthropogenic greenhouse gases from soil, but, comparatively recently, both CH4 and N2O have overtaken CO2, with N2O emissions now being of particular concern (Fig. 1). Urgent actions are required to protect the future climate by limiting greenhouse gas emissions from soil, such as the broad, overarching approaches indicated in Fig. 3. However, achieving these broad goals while simultaneously meeting human demands for food and other products and also considering socio-economic objectives will require the development of new and innovative approaches through to the use of incentives to encourage the adoption of existing approaches by landholders (Nkonya et al., 2016).
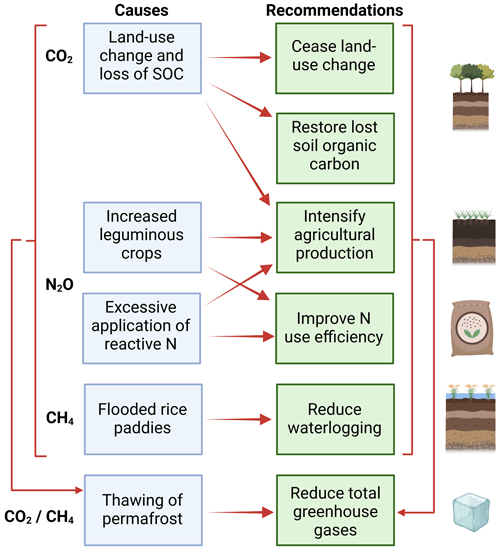
Figure 3Actions required to protect the future climate by limiting greenhouse gas emissions from soil. Note that “thawing of permafrost” is related to the magnitude of greenhouse gas emissions from soil due to anthropogenic management (such as by the loss of SOC from land use change or excessive application of reactive N), but any changes in management practices that reduce emissions of these greenhouse gases can also reduce the magnitude of this thawing and the associated release of greenhouse gases associated with the thawing of permafrost.
3.5 Cease land use change, including for bioenergy production
It is apparent that the release of CO2 from soil due to the loss of SOC following land use change has had the largest adverse effect on atmospheric greenhouse gas concentrations, contributing 11 % (+0.37 W m−2) to the total increase in warming due to well-mixed greenhouse gases. Much of this release of CO2 from soil due to land use change is historical, having peaked between 1800 and 1900 (Fig. 1), with current emissions of CO2 from the loss of SOC being dominated by ongoing land use change in “new-world” countries such as Brazil and Argentina (Sanderman et al., 2017). It is important to note that, although the human population has increased rapidly since the 1900s, the associated increase in food production has largely not come from area expansion (land use change) but rather from improving yields per unit area – the Green Revolution. Thus, these data demonstrate that urgent emphasis must be placed on ensuring that future land use change is ceased to limit further release of CO2. In particular, land use change for bioenergy production, which results in the substantial, long-term release of CO2 from SOC loss, should be targeted. Indeed, it has been estimated that the clearing of land to produce food-based biofuels creates a C debt by releasing 17–420 times more CO2 than the annual reductions that the biofuels would provide by displacing fossil fuels (Fargione et al., 2008).
Cessation of land use change is not only important for preventing further loss of SOC but is also vital to protect areas where vegetation and soils are currently acting as a net sink for atmospheric CO2 – the “terrestrial sink”. In this regard, the boreal and temperate forests of the Northern Hemisphere make the largest contribution to the terrestrial C sink (Canadell et al., 2021), with increased biomass production in these systems being largely driven by CO2 fertilization and lengthening growing seasons. This is in agreement with predictions of global SOC stocks, with large areas of land, especially the boreal forests of the Northern Hemisphere, having a net SOC gain (Sanderman et al., 2017). Nevertheless, a potential discrepancy remains regarding the importance of SOC within the terrestrial sink. Specifically, although studies estimate that the quantity of C captured within the terrestrial C sink is currently larger than that which is lost due to land use change (Canadell et al., 2021; Jia et al., 2019; Friedlingstein et al., 2023), leading to “increased vegetation and soil carbon” (IPCC, 2001), studies focusing primarily on SOC stocks report that net global SOC stocks are still decreasing at an average rate of ca. 0.1 Pg C yr−1 (Sanderman et al., 2017). In this regard, it is possible that, although elevated CO2 may increase C within vegetation, there may not necessarily be a corresponding increase in SOC (Sulman et al., 2019), for example, due to C destabilization (Bailey et al., 2019). Regardless, soil remains a net source of CO2 when historical emissions are included, and, hence, protecting the remaining terrestrial ecosystems is vital not only to prevent the loss of SOC that results from land use change but also because many of these systems are currently acting as a net C sink, as evidenced by their increasing SOC stocks.
3.6 Intensify agricultural production further while also increasing nitrogen use efficiency
Since the 1950s and 1960s, food production has generally increased by improving yields per unit area (intensification) rather than through area expansion. Although many factors have contributed to this improved productivity, a rapid increase in the use of reactive N fertilizers as part of the Green Revolution has been critical. Indeed, through the industrial production of reactive N fertilizers, the number of humans supported per hectare of arable land increased from 1.9 to 4.3 persons from 1908 to 2008 and with 30 %–50 % of the increase in crop yield having been achieved though the application of N fertilizers (Erisman et al., 2008; Stewart et al., 2005).
It is this agricultural intensification, supported by increasing rates of reactive N inputs, that has allowed rates of land use change to slow since the 1950s, with this, in turn, decreasing CO2 emissions from SOC loss (Fig. 1). However, while these inputs of reactive N into croplands have enabled a decrease in CO2 emissions from soil, the application of this N has concomitantly caused a rapid increase in N2O emissions (Fig. 1). Thus, while agricultural intensification has decreased the emissions of CO2 from soil, it has come at the expense of increasing N2O emissions – a potent greenhouse gas (Fig. 1).
Limiting future land use change while simultaneously increasing global food production will require even further intensification of agriculture (Kopittke et al., 2019). Thus, given the already rapidly increasing emissions of N2O from soil, it is imperative that strategies should be targeted through sustainable intensification (Pretty and Bharucha, 2014; Pretty et al., 2018) and that they should be developed and implemented to increase N use efficiency and decrease N2O emissions. This can be achieved by more closely aligning N supply with plant demand, such as through the repeated (multiple, strategic) applications of N fertilizer during the growing season, through the development of improved genotypes with higher N use efficiency, and through the use of slow-release fertilizers (Snyder et al., 2014). Increasing N use efficiency also has the additional advantages of decreasing soil acidification and environmental eutrophication while also having the additional agronomic benefit of increasing farmers' profitability. Of course, care must also be taken to ensure that this intensification of production (in order to limit future land use change) does not increase overall C losses, with changes in C gains and losses depending upon a range of factors such as agronomic practices, environmental conditions, and soil properties.
3.7 Decreases in the methane flux from soil can rapidly decrease radiative forcing
Although soil-based emissions of CH4 contribute a more modest 1.3 % (+0.044 W m−2) to radiative forcing (Figs. 1 and 2), decreasing the rate of CH4 emission from soil would yield a comparatively rapid decrease in radiative forcing given the fact that the atmospheric life of CH4 is only 11.8 years. In this regard, decreasing the emission of 30 Tg CH4 yr−1 from the soil of the 162 ×106 ha of rice paddies globally can potentially be achieved by reducing the period of time that the soil is waterlogged, with midseason drainage and intermittent irrigation being known to reduce CH4 emissions by up to 90 % (Souza et al., 2021; Islam et al., 2018). A range of other approaches have also been reported to be effective at reducing CH4 emissions from rice paddies (Nikolaisen et al., 2023; Hussain et al., 2015). For example, organic amendments such as straw should only be applied during aerobic conditions (during the off-season) rather than during on-season application (Nikolaisen et al., 2023). Such approaches are critical in maintaining yield while also decreasing CH4 emissions from soil (Smith et al., 2021). Furthermore, in many areas, strategies that will reduce CH4 emission from flooded rice culture will also deliver the benefit of increased water use efficiency.
3.8 Avoiding future thawing of permafrost
There is increasing concern regarding the release of CO2 due to the accelerating thawing of permafrost C in the Arctic and sub-Arctic. This permafrost contains ca. 1035 Pg of C up to 3 m depth (Schuur et al., 2015), with a warming climate causing increased thawing of the permafrost and the associated release of CO2 (and CH4). Indeed, it is estimated that ca. 92 Pg of C (ranging from 37 to 174 Pg of C) is susceptible to release as greenhouse gases in the present century (IPCC, 2019). Of these future C emissions from permafrost, it is expected that CO2 emissions will account for ca. 98 % of the C, with CH4 being expected to account for 2.3 % of future emissions (Schuur et al., 2013). While this proportion projected to be released as CH4 is comparatively small (2.3 %), given that CH4 has a global warming potential for a 100-year time horizon that is 27.9 times higher than that of CO2, this equates to an increased warming potential of this permafrost C of 35 %–48 % when accounting for the increased potency of the CH4 (Schuur et al., 2015). Thus, although the release of CO2 due to the loss of SOC from land use change has now slowed, the predicted release of CO2 from the thawing of permafrost (ca. 90 Pg of C) during the present century alone is of similar magnitude to the cumulative emissions of CO2 over the last >1000 years (116 Pg of C, Sanderman et al., 2017) due to progressive land use change, with this being a positive carbon–climate feedback. Thus, limiting the extent of future climate change is essential in preventing the profound release of CO2 and CH4 from permafrost.
3.9 Restore a portion of the soil organic carbon that has been lost historically
Of the 116 Pg of C that has been lost from soils historically (Sanderman et al., 2017), a portion can be restored by implementing best soil management practices on croplands and grazing lands. Smith et al. (2020) estimate a technical potential for soil carbon sequestration of up to 2.2 Pg C yr−1 globally, with an economic potential of 0.4–0.7 Pg C yr−1 (Smith et al., 2008). Assuming that a new equilibrium is reached in 20 years, as per IPCC Good Practice Guidance, this gives a cumulative maximum technical potential of 44 Pg C and an economic potential of 8–14 Pg C for restored SOC.
3.10 A broad portfolio of responses is required, including actions not related directly to soil
Solving the challenges associated with anthropogenic greenhouse gas emissions from soil will be highly complex, with the first step being to describe and quantify the underlying problems (as we have done in the present study) so that mitigation approaches can be targeted and specific. To this point, we have discussed soil-based approaches that address the individual challenges (such as land use change, improving N use efficiency, and so forth). However, it is clear that a multitude of responses will be required and not single approaches in isolation.
Additionally, we cannot solely focus on the biophysical issues as discussed above, but consideration must also be given to socio-economic and institutional aspects, which are also critically important (Nkonya et al., 2016). For example, although a range of management practices and technologies already exist to address some of these problems, many are not implemented fully, often due to economic drivers and the desire to maximize profits and minimize costs (Boardman et al., 2003). Consider, for example, that N fertilizer efficiency can often be improved by the use of slow-release sources or by the application of a larger number of smaller doses of N fertilizer to more closely match plant demand (compared to a lower number of larger doses), but the increased costs associated with such practices mean that they are often not implemented to the extent possible. In a similar manner, rural poverty is also an important driving factor, with many of the rural poor farming highly marginal lands where the necessity to provide food for their families overrides concerns for soil degradation and environmental harm.
There are also a range of other non-soil-based approaches that are not considered in detail here that could also make substantial contributions. For example, it is estimated that one-third of all food produced for human consumption is lost and wasted each year (Ishangulyyev et al., 2019), and, hence, reducing wastage could substantially reduce the area of land required for food production.
3.11 Limitations and uncertainties of the study
In the present study, we have gathered the best available global estimates of greenhouse gas emissions from soil from across multiple studies (Sanderman et al., 2017; Tian et al., 2019; Saunois et al., 2020), with each of these studies having various assumptions and uncertainties that are carried forward.
First, we acknowledge that the data presented within these previous studies have uncertainties and that these uncertainties influence the calculations presented within the present study. However, we are unable to include uncertainties in our calculations given that some of the previous studies themselves did not report them. For example, Sanderman et al. (2017) report that SOC loss is 116 Pg C, but these authors do not report a measure of uncertainty or error with this value. Thus, when calculating the contribution of soil-derived CO2, N2O, and CH4 to anthropogenic greenhouse gases, we are unable to provide error estimates for their total contribution. Regardless, it is important to note that the value of 116 Pg C reported by Sanderman et al. (2017) for agricultural land use change, for example, is similar to previously reported values, such as the 115–154 Pg C reported by Lal (2018), the 85 Pg C reported by Padarian et al. (2022) for cropping alone (i.e., excluding pasture and grasslands), and the 80–100 Pg C reported by Lal (1999) for the conversion of natural systems to managed systems. While our inability to include measures of uncertainty is a substantial limitation of our study, this reflects the observation that additional work is urgently required to allow for more rigorous assessments given the importance of soil-based emissions of greenhouse gases.
As a second limitation, we acknowledge the different time periods examined by the studies we have utilized for CO2 (>1000 years), N2O (>100 years), and CH4 (ca. 40 years). This limitation arises from differences between the three studies upon which we have based the present assessment. However, we do not consider this to be an important limitation. For N2O, we include data from the 1860s onwards, with anthropogenic emissions prior to this time being negligible (Tian et al., 2019); on the other hand, for CH4, although the data are available only for the last ca. 40 years, given that the atmospheric life of CH4 is 11.8 years, emissions of CH4 prior to this time would not make substantial contributions to current CH4 concentrations in the atmosphere. Thus, despite the time frames being markedly different between these previous studies, we contend that this does not influence the outcome markedly.
Third, it is important to note differences within the scope of the three studies upon which the present one is based. For example, the study of Sanderman et al. (2017) only examined agriculture and hence did not consider the effect of other forms of land use change, such as urban development, on the loss of SOC. Regardless, given that urban areas consist of ca. 0.7 % of global land surface (Zhao et al., 2022) while cropping accounts for 12 % of the ice-free land and permanent grassland and pasture account for 25 % (FAO, 2021), the magnitude of this error is likely to be small.
Fourth, we make the assumption here that all SOC that is lost from soil has been emitted to the atmosphere as CO2 through mineralization. We expect that the error from this assumption is only small given that, during the early stages (up to ca. 20 years) following land use conversion, when the loss of SOC is the greatest, 80 % of the SOC is lost due to mineralization, while 20 % is lost to erosion (Lal, 2001). Furthermore, even for SOC, which is eroded rather than directly mineralized, the majority of this eroded SOC is simply redistributed to other soil within the landscape – of the 5.7 Pg C eroded by water annually, 3.9 Pg C is simply redistributed over the landscape, 0.57 Pg C is buried in lakes and reservoirs, and 1.14 Pg C is mineralized (Lal, 1995). Thus, while not all SOC that is lost from soil is mineralized to the atmosphere as CO2, the error is likely to be small.
Despite the clear limitations noted above, we collate these data as a starting point to highlight and discuss the critical importance of anthropogenic management of soil in relation to greenhouse gas emissions. In this regard, it is imperative that future studies refine these estimates with more comprehensive data. Regardless, despite these uncertainties, the relative importance (contribution) of each greenhouse gas over different timescales is unlikely to differ greatly from that presented here, even once better data become available.
In the present study, we have focused on quantifying the overall contribution of soil to global anthropogenic greenhouse gas emissions and global climate change, comparing CO2, N2O, and CH4. By comparing the historical and current global emissions between these three gases, it has been possible to identify important trends over time, with this being critical for focusing future efforts. It is important to note, however, that there is also spatial variability in these emissions across the planet. For example, it is known that some soils (particularly those of the boreal and temperate forests of the Northern Hemisphere) are making an increased contribution to the terrestrial (vegetation plus soil) C sink (Canadell et al., 2021; Sanderman et al., 2017). Thus, developing detailed plans to mitigate C emissions (or other greenhouse gases) must first consider such variability.
Finally, it must be noted that, in the present study, we have focused on the release of greenhouse gases due to anthropogenic management of soil, and we have not considered the role of soil in removing CO2 from the atmosphere and by means of SOC. Indeed, there are an increasing number of studies examining the role of soil as a “negative emission technology” (NET) for the capture of CO2 from the atmosphere (Smith, 2012; Paustian et al., 2016; Minasny et al., 2017; Lal et al., 2021; van Vuuren et al., 2018). However, for soil to be effective as an NET, we must first reduce the substantial emissions of greenhouse gases from soil.
Our increasing focus on soil's ability to provide biomass (especially food) for human use through intensive agriculture has caused soil to release profound amounts of greenhouse gases, with this threatening planetary survivability. We show that anthropogenic emissions of greenhouse gases from soil account for 15 % of the entire global increase in warming (radiative forcing) caused by well-mixed greenhouse gases. Although CO2 is the most important greenhouse gas emitted from soil (74 % of the total warming), much of this CO2 has been emitted historically, with current rates of release being considerably lower. Thus, for CO2, efforts should be directed towards preventing the rate of release from increasing again by preventing ongoing land use change and restoring a portion of the historically lost soil organic carbon through soil best management practices while also preventing global warming that will result in release from permafrost. However, preventing further land use change will require ongoing intensification of production through increased N fertilizer application, with strategies being required to markedly improve N fertilizer efficiency and to limit the already rapidly accelerating emissions of N2O – a potent greenhouse gas. We also need to decrease CH4 emissions from rice paddies, which, although it may only have a comparatively modest impact, has the advantage that reduced emissions result in a benefit in the short term. Finally, although the present study highlights how human use of soil is resulting in substantial releases of greenhouse gases and contributing to global warming, it is also important to note that soil also acts as a sink for greenhouse gases (whether emitted from soil or from other anthropogenic sources), with this also being a critical role of soil. Recognizing the central importance of soil in contributing to climate change is essential if we are to maintain planetary hospitability.
The data and analyses that support these findings will be made available in response to a reasonable request.
Conceptualization: PMK, RCD, BAM, and PW. Methodology: PMK, RCD, PS, and NWM. Formal analysis: PMK, RCD, BAM, PW, ZW, and FJTvdB. Writing: PMK, RCD, BAM, PS, PW, ZW, FJTvdB, and NWM.
The contact author has declared that none of the authors has any competing interests.
Publisher's note: Copernicus Publications remains neutral with regard to jurisdictional claims made in the text, published maps, institutional affiliations, or any other geographical representation in this paper. While Copernicus Publications makes every effort to include appropriate place names, the final responsibility lies with the authors.
The authors acknowledge the assistance of Yriah Rusden.
This paper was edited by Ngonidzashe Chirinda and reviewed by two anonymous referees.
Amundson, R.: Soil biogeochemistry and the global agricultural footprint, Soil Security, 6, 100022, https://doi.org/10.1016/j.soisec.2021.100022, 2022.
Bailey, V. L., Pries, C. H., and Lajtha, K.: What do we know about soil carbon destabilization?, Environ. Res. Lett., 14, 083004, https://doi.org/10.1088/1748-9326/ab2c11, 2019.
Boardman, J., Poesen, J., and Evans, R.: Socio-economic factors in soil erosion and conservation, Environ. Sci. Policy, 6, 1–6, https://doi.org/10.1016/S1462-9011(02)00120-X, 2003.
Canadell, J. G., Monteiro, P. M. S., Costa, M. H., Cotrim da Cunha, L., Cox, P. M., Eliseev, A. V., Henson, S., Ishii, M., Jaccard, S., Koven, C., Lohila, A., Patra, P. K., Piao, S., Rogelj, J., Syampungani, S., Zaehle, S., and Zickfeld, K.: Global carbon and other biogeochemical cycles and feedbacks, in: Climate Change 2021: The Physical Science Basis. Contribution of Working Group I to the Sixth Assessment Report of the Intergovernmental Panel on Climate Change, edited by: Masson-Delmotte, V., Zhai, P., Pirani, A., Connors, S. L., Péan, C., Berger, S., Caud, N., Chen, Y., Goldfarb, L., Gomis, M. I., Huang, M., Leitzell, K., Lonnoy, E., Matthews, J. B. R., Maycock, T. K., Waterfield, T., Yelekçi, O., Yu, R., and Zhou, B., Cambridge University Press, https://doi.org/10.1017/9781009157896.007, 2021.
Crow, S. E. and Sierra, C. A.: The climate benefit of sequestration in soils for warming mitigation, Biogeochemistry, 161, 71–84, https://doi.org/10.1007/s10533-022-00981-1, 2022.
Erisman, J. W., Sutton, M. A., Galloway, J., Klimont, Z., and Winiwarter, W.: How a century of ammonia synthesis changed the world, Nat. Geosci., 1, 636–639, 2008.
FAO: FAO Statistical Databases, Food and Agriculture Organization of the United Nations (FAO), http://www.fao.org/faostat/ (last access: 21 November 2021), 2021.
Fargione, J., Hill, J., Tilman, D., Polasky, S., and Hawthorne, P.: Land clearing and the biofuel carbon debt, Science, 319, 1235–1238, https://doi.org/10.1126/science.1152747, 2008.
Forster, P., Storelvmo, T., Armour, K., Collins, W., Dufresne, J. L., Frame, D., Lunt, D. J., Mauritsen, T., Palmer, M. D., Watanabe, M., Wild, M., and Zhang, H.: The Earth's energy budget, climate feedbacks, and climate sensitivity, in: Climate Change 2021: The Physical Science Basis. Contribution of Working Group I to the Sixth Assessment Report of the Intergovernmental Panel on Climate Change, edited by: Masson-Delmotte, V., Zhai, P., Pirani, A., Connors, S. L., Péan, C., Berger, S., Caud, N., Chen, Y., Goldfarb, L., Gomis, M. I., Huang, M., Leitzell, K., Lonnoy, E., Matthews, J. B. R., Maycock, T. K., Waterfield, T., Yelekçi, O., Yu, R., and Zhou, B., Cambridge University Press, https://doi.org/10.1017/9781009157896.009, 2021.
Friedlingstein, P., O'Sullivan, M., Jones, M. W., Andrew, R. M., Bakker, D. C. E., Hauck, J., Landschützer, P., Le Quéré, C., Luijkx, I. T., Peters, G. P., Peters, W., Pongratz, J., Schwingshackl, C., Sitch, S., Canadell, J. G., Ciais, P., Jackson, R. B., Alin, S. R., Anthoni, P., Barbero, L., Bates, N. R., Becker, M., Bellouin, N., Decharme, B., Bopp, L., Brasika, I. B. M., Cadule, P., Chamberlain, M. A., Chandra, N., Chau, T.-T.-T., Chevallier, F., Chini, L. P., Cronin, M., Dou, X., Enyo, K., Evans, W., Falk, S., Feely, R. A., Feng, L., Ford, D. J., Gasser, T., Ghattas, J., Gkritzalis, T., Grassi, G., Gregor, L., Gruber, N., Gürses, Ö., Harris, I., Hefner, M., Heinke, J., Houghton, R. A., Hurtt, G. C., Iida, Y., Ilyina, T., Jacobson, A. R., Jain, A., Jarníková, T., Jersild, A., Jiang, F., Jin, Z., Joos, F., Kato, E., Keeling, R. F., Kennedy, D., Klein Goldewijk, K., Knauer, J., Korsbakken, J. I., Körtzinger, A., Lan, X., Lefèvre, N., Li, H., Liu, J., Liu, Z., Ma, L., Marland, G., Mayot, N., McGuire, P. C., McKinley, G. A., Meyer, G., Morgan, E. J., Munro, D. R., Nakaoka, S.-I., Niwa, Y., O'Brien, K. M., Olsen, A., Omar, A. M., Ono, T., Paulsen, M., Pierrot, D., Pocock, K., Poulter, B., Powis, C. M., Rehder, G., Resplandy, L., Robertson, E., Rödenbeck, C., Rosan, T. M., Schwinger, J., Séférian, R., Smallman, T. L., Smith, S. M., Sospedra-Alfonso, R., Sun, Q., Sutton, A. J., Sweeney, C., Takao, S., Tans, P. P., Tian, H., Tilbrook, B., Tsujino, H., Tubiello, F., van der Werf, G. R., van Ooijen, E., Wanninkhof, R., Watanabe, M., Wimart-Rousseau, C., Yang, D., Yang, X., Yuan, W., Yue, X., Zaehle, S., Zeng, J., and Zheng, B.: Global Carbon Budget 2023, Earth Syst. Sci. Data, 15, 5301–5369, https://doi.org/10.5194/essd-15-5301-2023, 2023.
Gulev, S. K., Thorne, P. W., Ahn, J., Dentener, F. J., Domingues, C. M., Gerland, S., Gong, D., Kaufman, D. S., Nnamchi, H. C., Quaas, J., Rivera, J. A., Sathyendranath, S., Smith, S. L., Trewin, B., von Shuckmann, K., and Vose, R. S.: Changing state of the climate system, in: Climate Change 2021: The Physical Science Basis. Contribution of Working Group I to the Sixth Assessment Report of the Intergovernmental Panel on Climate Change, edited by: Masson-Delmotte, V., Zhai, P., Pirani, A., Connors, S. L., Péan, C., Berger, S., Caud, N., Chen, Y., Goldfarb, L., Gomis, M. I., Huang, M., Leitzell, K., Lonnoy, E., Matthews, J. B. R., Maycock, T. K., Waterfield, T., Yelekçi, O., Yu, R., and Zhou, B., Cambridge University Press, https://doi.org/10.1017/9781009157896.004, 2021.
Guo, L. B. and Gifford, R. M.: Soil carbon stocks and land use change: a meta analysis, Glob. Change Biol., 8, 345–360, https://doi.org/10.1046/j.1354-1013.2002.00486.x, 2002.
He, J., Naik, V., Horowitz, L. W., Dlugokencky, E., and Thoning, K.: Investigation of the global methane budget over 1980–2017 using GFDL-AM4.1, Atmos. Chem. Phys., 20, 805–827, https://doi.org/10.5194/acp-20-805-2020, 2020.
Hussain, S., Peng, S., Fahad, S., Khaliq, A., Huang, J., Cui, K., and Nie, L.: Rice management interventions to mitigate greenhouse gas emissions: a review, Environ. Sci. Pollut. R., 22, 3342–3360, https://doi.org/10.1007/s11356-014-3760-4, 2015.
IPCC: The carbon cycle and atmospheric carbon dioxide, in: TAR Climate Change 2001: The Scientific Basis, Intergovernmental Panel on Climate Change, Cambridge University Press, Cambridge, United Kingdom and New York, NY, USA, 881 pp., ISBN 0521 01495 6, 2001.
IPCC: IPCC Special Report on the Ocean and Cryosphere in a Changing Climate, Intergovernmental Panel on Climate Change, https://doi.org/10.1017/9781009157964, 2019.
IPCC: Summary for policymakers, in: Climate Change 2021: The Physical Science Basis. Contribution of Working Group I to the Sixth Assessment Report of the Intergovernmental Panel on Climate Change, edited by: Masson-Delmotte, V., Zhai, P., Pirani, A., Connors, S. L., Péan, C., Berger, S., Caud, N., Chen, Y., Goldfarb, L., Gomis, M. I., Huang, M., Leitzell, K., Lonnoy, E., Matthews, J. B. R., Maycock, T. K., Waterfield, T., Yelekçi, O., Yu, R., and Zhou, B., Cambridge University Press, https://doi.org/10.1017/9781009157896.001, 2021.
Ishangulyyev, R., Kim, S., and Lee, S. H.: Understanding food loss and waste—Why are we losing and wasting food?, Foods, 8, 297, https://doi.org/10.3390/foods8080297, 2019.
Islam, S. F.-u., van Groenigen, J. W., Jensen, L. S., Sander, B. O., and de Neergaard, A.: The effective mitigation of greenhouse gas emissions from rice paddies without compromising yield by early-season drainage, Sci. Total Environ., 612, 1329–1339, https://doi.org/10.1016/j.scitotenv.2017.09.022, 2018.
Jia, G., Shevliakova, E., Artaxo, P., De Noblet-Ducoudré, N., Houghton, R., House, J., Kitajima, K., Lennard, C., Popp, A., Sirin, A., Sukumar, R., and Verchot, L.: Land–climate interactions, in: Climate Change and Land: an IPCC special report on climate change, desertification, land degradation, sustainable land management, food security, and greenhouse gas fluxes in terrestrial ecosystems, edited by: Shukla, P. R., Skea, J., Calvo Buendia, E., Masson-Delmotte, V., Pörtner, H.-O., Roberts, D. C., Zhai, P., Slade, R., Connors, S., van Diemen, R., Ferrat, M., Haughey, E., Luz, S., Neogi, S., Pathak, M., Petzold, J., Portugal Pereira, J., Vyas, P., Huntley, E., Kissick, K., Belkacemi, M., and Malley, J., Intergovernmental Panel on Climate Change, https://doi.org/10.1017/9781009157988, 2019.
Jiang, Y., Qian, H., Huang, S., Zhang, X., Wang, L., Zhang, L., Shen, M., Xiao, X., Chen, F., Zhang, H., Lu, C., Li, C., Zhang, J., Deng, A., van Groenigen, K. J., and Zhang, W.: Acclimation of methane emissions from rice paddy fields to straw addition, Science Advances, 5, eaau9038, https://doi.org/10.1126/sciadv.aau9038, 2019.
Klein Goldewijk, K., Beusen, A., Doelman, J., and Stehfest, E.: Anthropogenic land use estimates for the Holocene – HYDE 3.2, Earth Syst. Sci. Data, 9, 927–953, https://doi.org/10.5194/essd-9-927-2017, 2017.
Koch, A., McBratney, A., Adams, M., Field, D., Hill, R., Crawford, J., Minasny, B., Lal, R., Abbott, L., O'Donnell, A., Angers, D., Baldock, J., Barbier, E., Binkley, D., Parton, W., Wall, D. H., Bird, M., Bouma, J., Chenu, C., Flora, C. B., Goulding, K., Grunwald, S., Hempel, J., Jastrow, J., Lehmann, J., Lorenz, K., Morgan, C. L., Rice, C. W., Whitehead, D., Young, I., and Zimmermann, M.: Soil security: Solving the global soil crisis, Glob. Policy, 4, 434–441, https://doi.org/10.1111/1758-5899.12096, 2013.
Kopittke, P. M., Dalal, R. C., Finn, D., and Menzies, N. W.: Global changes in soil stocks of carbon, nitrogen, phosphorus, and sulphur as influenced by long-term agricultural production, Glob. Change Biol., 23, 2509–2519, 2017.
Kopittke, P. M., Menzies, N. W., Wang, P., McKenna, B. A., and Lombi, E.: Soil and the intensification of agriculture for global food security, Environ. Int., 132, 105078, https://doi.org/10.1016/j.envint.2019.105078, 2019.
Lal, R.: Global soil erosion by water and carbon dynamics, in: Soils and Global Change, edited by: Lal, R., Kimble, J. M., Levine, E., and Stewart, B. A., CRC/Lewis, Boca Raton, Florida, 131–141, ISBN 9781566701181, 1995.
Lal, R.: Soil management and restoration for C sequestration to mitigate the accelerated greenhouse effect, Progress in Environmental Science, 1, 307–326, 1999.
Lal, R.: World cropland soils as a source or sink for atmospheric carbon, Adv. Agron., 71, 145–191, 2001.
Lal, R.: Digging deeper: A holistic perspective of factors affecting soil organic carbon sequestration in agroecosystems, Glob. Change Biol., 24, 3285–3301, https://doi.org/10.1111/gcb.14054, 2018.
Lal, R., Monger, C., Nave, L., and Smith, P.: The role of soil in regulation of climate, Philos. T. R. Soc. B, 376, 20210084, https://doi.org/10.1098/rstb.2021.0084, 2021.
Langeveld, J. W. A., Dixon, J., van Keulen, H., and Quist-Wessel, P. M. F.: Analyzing the effect of biofuel expansion on land use in major producing countries: evidence of increased multiple cropping, Biofuel. Bioprod. Bior., 8, 49–58, https://doi.org/10.1002/bbb.1432, 2013.
Lehmann, J. and Kleber, M.: The contentious nature of soil organic matter, Nature, 528, 60–68, https://doi.org/10.1038/nature16069, 2015.
Minasny, B., Malone, B. P., McBratney, A. B., Angers, D. A., Arrouays, D., Chambers, A., Chaplot, V., Chen, Z.-S., Cheng, K., Das, B. S., Field, D. J., Gimona, A., Hedley, C. B., Hong, S. Y., Mandal, B., Marchant, B. P., Martin, M., McConkey, B. G., Mulder, V. L., O'Rourke, S., Richer-de-Forges, A. C., Odeh, I., Padarian, J., Paustian, K., Pan, G., Poggio, L., Savin, I., Stolbovoy, V., Stockmann, U., Sulaeman, Y., Tsui, C.-C., Vågen, T.-G., van Wesemael, B., and Winowiecki, L.: Soil carbon 4 per mille, Geoderma, 292, 59–86, https://doi.org/10.1016/j.geoderma.2017.01.002, 2017.
Murty, D., Kirschbaum, M. U. F., McMurtrie, R. E., and McGilvray, H.: Does conversion of forest to agricultural land change soil carbon and nitrogen? A review of the literature, Glob. Change Biol., 8, 105–123, https://doi.org/10.1046/j.1354-1013.2001.00459.x, 2002.
Nikolaisen, M., Cornulier, T., Hillier, J., Smith, P., Albanito, F., and Nayak, D.: Methane emissions from rice paddies globally: A quantitative statistical review of controlling variables and modelling of emission factors, J. Clean. Prod., 409, 137245, https://doi.org/10.1016/j.jclepro.2023.137245, 2023.
Nkonya, E., von Braun, J., Mirzabaev, A., Le, Q. B., Kwon, H.-Y., and Kirui, O.: Concepts and methods of global assessment of the economics of land degradation and improvement, in: Economics of Land Degradation and Improvement – A Global Assessment for Sustainable Development, edited by: Nkonya, E., Mirzabaev, A., and von Braun, J., Springer International Publishing, Cham, https://doi.org/10.1007/978-3-319-19168-3_2, 15–32, 2016.
Oertel, C., Matschullat, J., Zurba, K., Zimmermann, F., and Erasmi, S.: Greenhouse gas emissions from soils—A review, Geochemistry, 76, 327–352, https://doi.org/10.1016/j.chemer.2016.04.002, 2016.
Padarian, J., Minasny, B., McBratney, A., and Smith, P.: Soil carbon sequestration potential in global croplands, PeerJ, 10, e13740, https://doi.org/10.7717/peerj.13740, 2022.
Paustian, K., Lehmann, J., Ogle, S., Reay, D., Robertson, G. P., and Smith, P.: Climate-smart soils, Nature, 532, 49, https://doi.org/10.1038/nature17174, 2016.
Pretty, J. and Bharucha, Z. P.: Sustainable intensification in agricultural systems, Ann. Bot., 114, 1571–1596, https://doi.org/10.1093/aob/mcu205, 2014.
Pretty, J., Benton, T. G., Bharucha, Z. P., Dicks, L. V., Flora, C. B., Godfray, H. C. J., Goulson, D., Hartley, S., Lampkin, N., Morris, C., Pierzynski, G., Prasad, P. V. V., Reganold, J., Rockström, J., Smith, P., Thorne, P., and Wratten, S.: Global assessment of agricultural system redesign for sustainable intensification, Nat. Sustain., 1, 441–446, https://doi.org/10.1038/s41893-018-0114-0, 2018.
Rittel, H. W. J. and Webber, M. M.: Dilemmas in a general theory of planning, Policy Sci., 4, 155–169, https://doi.org/10.1007/BF01405730, 1973.
Robertson, G. P., Paul, E. A., and Harwood, R. R.: Greenhouse gases in intensive agriculture: Contributions of individual gases to the radiative forcing of the atmosphere, Science, 289, 1922–1925, https://doi.org/10.1126/science.289.5486.1922, 2000.
Sanderman, J., Hengl, T., and Fiske, G. J.: Soil carbon debt of 12,000 years of human land use, P. Natl. Acad. Sci. USA, 114, 9575–9580, https://doi.org/10.1073/pnas.1706103114, 2017.
Saunois, M., Stavert, A. R., Poulter, B., Bousquet, P., Canadell, J. G., Jackson, R. B., Raymond, P. A., Dlugokencky, E. J., Houweling, S., Patra, P. K., Ciais, P., Arora, V. K., Bastviken, D., Bergamaschi, P., Blake, D. R., Brailsford, G., Bruhwiler, L., Carlson, K. M., Carrol, M., Castaldi, S., Chandra, N., Crevoisier, C., Crill, P. M., Covey, K., Curry, C. L., Etiope, G., Frankenberg, C., Gedney, N., Hegglin, M. I., Höglund-Isaksson, L., Hugelius, G., Ishizawa, M., Ito, A., Janssens-Maenhout, G., Jensen, K. M., Joos, F., Kleinen, T., Krummel, P. B., Langenfelds, R. L., Laruelle, G. G., Liu, L., Machida, T., Maksyutov, S., McDonald, K. C., McNorton, J., Miller, P. A., Melton, J. R., Morino, I., Müller, J., Murguia-Flores, F., Naik, V., Niwa, Y., Noce, S., O'Doherty, S., Parker, R. J., Peng, C., Peng, S., Peters, G. P., Prigent, C., Prinn, R., Ramonet, M., Regnier, P., Riley, W. J., Rosentreter, J. A., Segers, A., Simpson, I. J., Shi, H., Smith, S. J., Steele, L. P., Thornton, B. F., Tian, H., Tohjima, Y., Tubiello, F. N., Tsuruta, A., Viovy, N., Voulgarakis, A., Weber, T. S., van Weele, M., van der Werf, G. R., Weiss, R. F., Worthy, D., Wunch, D., Yin, Y., Yoshida, Y., Zhang, W., Zhang, Z., Zhao, Y., Zheng, B., Zhu, Q., Zhu, Q., and Zhuang, Q.: The Global Methane Budget 2000–2017, Earth Syst. Sci. Data, 12, 1561–1623, https://doi.org/10.5194/essd-12-1561-2020, 2020.
Schuur, E. A. G., Abbott, B. W., Bowden, W. B., Brovkin, V., Camill, P., Canadell, J. G., Chanton, J. P., Chapin, F. S., Christensen, T. R., Ciais, P., Crosby, B. T., Czimczik, C. I., Grosse, G., Harden, J., Hayes, D. J., Hugelius, G., Jastrow, J. D., Jones, J. B., Kleinen, T., Koven, C. D., Krinner, G., Kuhry, P., Lawrence, D. M., McGuire, A. D., Natali, S. M., O'Donnell, J. A., Ping, C. L., Riley, W. J., Rinke, A., Romanovsky, V. E., Sannel, A. B. K., Schädel, C., Schaefer, K., Sky, J., Subin, Z. M., Tarnocai, C., Turetsky, M. R., Waldrop, M. P., Walter Anthony, K. M., Wickland, K. P., Wilson, C. J., and Zimov, S. A.: Expert assessment of vulnerability of permafrost carbon to climate change, Climatic Change, 119, 359–374, https://doi.org/10.1007/s10584-013-0730-7, 2013.
Schuur, E. A. G., McGuire, A. D., Schädel, C., Grosse, G., Harden, J. W., Hayes, D. J., Hugelius, G., Koven, C. D., Kuhry, P., Lawrence, D. M., Natali, S. M., Olefeldt, D., Romanovsky, V. E., Schaefer, K., Turetsky, M. R., Treat, C. C., and Vonk, J. E.: Climate change and the permafrost carbon feedback, Nature, 520, 171–179, https://doi.org/10.1038/nature14338, 2015.
Smith, P.: Soils and climate change, Curr. Opin. Env. Sust., 4, 539–544, https://doi.org/10.1016/j.cosust.2012.06.005, 2012.
Smith, P., Martino, D., Cai, Z., Gwary, D., Janzen, H., Kumar, P., McCarl, B., Ogle, S., O'Mara, F., Rice, C., Scholes, B., Sirotenko, O., Howden, M., McAllister, T., Pan, G., Romanenkov, V., Schneider, U., Towprayoon, S., Wattenbach, M., and Smith, J.: Greenhouse gas mitigation in agriculture, Philos. T. R. Soc. B, 363, 789–813, https://doi.org/10.1098/rstb.2007.2184, 2008.
Smith, P., Calvin, K., Nkem, J., Campbell, D., Cherubini, F., Grassi, G., Korotkov, V., Le Hoang, A., Lwasa, S., McElwee, P., Nkonya, E., Saigusa, N., Soussana, J.-F., Taboada, M. A., Manning, F. C., Nampanzira, D., Arias-Navarro, C., Vizzarri, M., House, J., Roe, S., Cowie, A., Rounsevell, M., and Arneth, A.: Which practices co-deliver food security, climate change mitigation and adaptation, and combat land degradation and desertification?, Glob. Change Biol., 26, 1532–1575, https://doi.org/10.1111/gcb.14878, 2020.
Smith, P., Reay, D., and Smith, J.: Agricultural methane emissions and the potential formitigation, Philos. T. R. Soc. A, 379, 20200451, https://doi.org/10.1098/rsta.2020.0451, 2021.
Snyder, C. S., Davidson, E. A., Smith, P., and Venterea, R. T.: Agriculture: sustainable crop and animal production to help mitigate nitrous oxide emissions, Curr. Opin. Env. Sust., 9–10, 46–54, https://doi.org/10.1016/j.cosust.2014.07.005, 2014.
Souza, R., Yin, J., and Calabrese, S.: Optimal drainage timing for mitigating methane emissions from rice paddy fields, Geoderma, 394, 114986, https://doi.org/10.1016/j.geoderma.2021.114986, 2021.
Steffen, W., Richardson, K., Rockström, J., Cornell, S. E., Fetzer, I., Bennett, E. M., Biggs, R., Carpenter, S. R., de Vries, W., de Wit, C. A., Folke, C., Gerten, D., Heinke, J., Mace, G. M., Persson, L. M., Ramanathan, V., Reyers, B., and Sörlin, S.: Planetary boundaries: Guiding human development on a changing planet, Science, 347, 1259855, https://doi.org/10.1126/science.1259855, 2015.
Stewart, W. M., Dibb, D. W., Johnston, A. E., and Smyth, T. J.: The contribution of commercial fertilizer nutrients to food production, Agron. J., 97, 1–6, https://doi.org/10.2134/agronj2005.0001, 2005.
Sulman, B. N., Shevliakova, E., Brzostek, E. R., Kivlin, S. N., Malyshev, S., Menge, D. N. L., and Zhang, X.: Diverse mycorrhizal associations enhance terrestrial C storage in a global model, Global Biogeochem. Cy., 33, 501–523, https://doi.org/10.1029/2018GB005973, 2019.
Syakila, A. and Kroeze, C.: The global nitrous oxide budget revisited, Greenhouse Gas Measurement and Management, 1, 17–26, https://doi.org/10.3763/ghgmm.2010.0007, 2011.
Tian, H., Yang, J., Xu, R., Lu, C., Canadell, J. G., Davidson, E. A., Jackson, R. B., Arneth, A., Chang, J., Ciais, P., Gerber, S., Ito, A., Joos, F., Lienert, S., Messina, P., Olin, S., Pan, S., Peng, C., Saikawa, E., Thompson, R. L., Vuichard, N., Winiwarter, W., Zaehle, S., and Zhang, B.: Global soil nitrous oxide emissions since the preindustrial era estimated by an ensemble of terrestrial biosphere models: Magnitude, attribution, and uncertainty, Glob. Change Biol., 25, 640–659, https://doi.org/10.1111/gcb.14514, 2019.
Tian, H., Xu, R., Canadell, J. G., Thompson, R. L., Winiwarter, W., Suntharalingam, P., Davidson, E. A., Ciais, P., Jackson, R. B., Janssens-Maenhout, G., Prather, M. J., Regnier, P., Pan, N., Pan, S., Peters, G. P., Shi, H., Tubiello, F. N., Zaehle, S., Zhou, F., Arneth, A., Battaglia, G., Berthet, S., Bopp, L., Bouwman, A. F., Buitenhuis, E. T., Chang, J., Chipperfield, M. P., Dangal, S. R. S., Dlugokencky, E., Elkins, J. W., Eyre, B. D., Fu, B., Hall, B., Ito, A., Joos, F., Krummel, P. B., Landolfi, A., Laruelle, G. G., Lauerwald, R., Li, W., Lienert, S., Maavara, T., MacLeod, M., Millet, D. B., Olin, S., Patra, P. K., Prinn, R. G., Raymond, P. A., Ruiz, D. J., van der Werf, G. R., Vuichard, N., Wang, J., Weiss, R. F., Wells, K. C., Wilson, C., Yang, J., and Yao, Y.: A comprehensive quantification of global nitrous oxide sources and sinks, Nature, 586, 248–256, https://doi.org/10.1038/s41586-020-2780-0, 2020.
van Vuuren, D. P., Stehfest, E., Gernaat, D. E. H. J., van den Berg, M., Bijl, D. L., de Boer, H. S., Daioglou, V., Doelman, J. C., Edelenbosch, O. Y., Harmsen, M., Hof, A. F., and van Sluisveld, M. A. E.: Alternative pathways to the 1.5 °C target reduce the need for negative emission technologies, Nat. Clim. Change, 8, 391–397, https://doi.org/10.1038/s41558-018-0119-8, 2018.
Zhao, M., Cheng, C., Zhou, Y., Li, X., Shen, S., and Song, C.: A global dataset of annual urban extents (1992–2020) from harmonized nighttime lights, Earth Syst. Sci. Data, 14, 517–534, https://doi.org/10.5194/essd-14-517-2022, 2022.