the Creative Commons Attribution 4.0 License.
the Creative Commons Attribution 4.0 License.
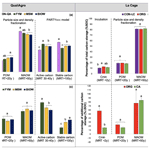
What is the stability of additional organic carbon stored thanks to alternative cropping systems and organic waste product application? A multi-method evaluation
Tchodjowiè P. I. Kpemoua
Pierre Barré
Sabine Houot
François Baudin
Cédric Plessis
Claire Chenu
The implementation of agroecological practices often leads to additional soil organic carbon storage, and we have sought to assess the biogeochemical stability of this additional carbon. To achieve this, we implemented a multi-method approach using particle size and density fractionation, Rock-Eval® (RE) thermal analyses and long-term incubation (484 d), which we applied to topsoil samples (0–30 cm) from temperate Luvisols that had been subjected in >20-year-long experiments in France to conservation agriculture (CA), organic agriculture (ORG) and conventional agriculture (CON-LC) in the La Cage experiment and to organic waste product (OWP) applications in the QualiAgro experiment, including biowaste compost (BIOW), residual municipal solid waste compost (MSW), farmyard manure (FYM) and conventional agriculture without organic inputs (CON-QA). The additional carbon resulting from agroecological practices is the difference between the carbon stock of the bulk soil and physical fractions or carbon pools in the soil affected by agroecological practices and that of the same soil affected by a conventional practice used as control. The incubations provided information on the additional carbon stability in the short term (i.e. mean residence time, MRT, of <2 years) and showed that the additional soil organic carbon mineralized faster than the carbon in the conventional control at La Cage but slower at QualiAgro. In OWP-treated plots at QualiAgro, 60 %–66 % of the additional carbon was stored as mineral-associated organic matter (MAOM-C) and 34 %–40 % as particulate organic matter (POM-C). In CA and ORG systems at La Cage, 77 %–84 % of the additional carbon was stored as MAOM-C, whereas 16 %–23 % was stored as POM-C. Management practices hence influenced the distribution of additional carbon in physical fractions. Utilizing the PARTYSOC model with Rock-Eval® thermal analysis parameters, we found that most, if not all, of the additional carbon belonged to the active carbon pool (MRT∼30–40 years). In summary, our comprehensive multi-method evaluation indicates that the additional soil organic carbon is less stable over decadal and pluri-decadal timescales compared to soil carbon under conventional control conditions. Our results show that particle size and density fractions can be heterogenous in their biogeochemical stability. On the other hand, although the additional carbon is mainly associated with MAOM, the high proportion of this carbon in the active pool suggests that it has a mean residence time which does not exceed ∼50 years. Furthermore, agroecological practices with equivalent additional carbon stocks (MSW, FYM and CA) exhibited a higher proportion of additional carbon in POM-C under MSW (40 %) and FYM (34 %) compared to CA (16 %), which suggests a high chemical recalcitrance of POM-C under OWP management relative to conservation agriculture. Additional soil organic carbon derived from organic waste, i.e. biomass that has partially decomposed and has been transformed through its processing prior to its incorporation in soil, would be more biogeochemically stable in soil than that derived directly from plant biomass. The apparent contradictions observed between methods can be explained by the fact that they address different kinetic pools of organic carbon. Care must be taken to specify which range of residence times is considered when using any method with the intent to evaluate the biogeochemical stability of soil organic matter, as well as when using the terms stable or labile. In conclusion, the contrasting biogeochemical stabilities observed in the different management options highlight the need to maintain agroecological practices to keep these carbon stocks at a high level over time, given that the additional carbon is stable on a pluri-decadal scale.
- Article
(4530 KB) - Full-text XML
-
Supplement
(637 KB) - BibTeX
- EndNote
Soil organic matter (SOM) plays a crucial role in the functioning of terrestrial ecosystems and can contribute to mitigating climate change. A minor change in soil organic carbon (SOC) content can make a significant difference in global climate because soil contains more carbon than vegetation and atmosphere combined (Lal, 2004). The 4p1000 initiative encourages the implementation of agricultural practices that increase and/or maintain soil carbon stocks (http://www.4p1000.org, last access: December 2022; Rumpel et al., 2020). At the field scale, changes in SOC stocks result from an imbalance between C inputs (crop residues; litter; root exudates; exogenous organic matter such as organic waste products, OWPs) and C outputs from the system due to crop residue export, SOC mineralization, leaching or erosion (Lal, 2018). Although some agricultural practices can reduce mineralization rates (e.g. reduced tillage; see review by Haddaway et al., 2017), it is generally accepted that the most effective way to increase SOC stocks is to increase carbon inputs (e.g. Virto et al., 2012; Autret et al., 2016; Fujisaki et al., 2018; Chenu et al., 2019). This can be achieved by increasing biomass production in the field and residue return (e.g. cover crops, Poeplau and Don, 2015; Autret et al., 2016) or by mobilizing external carbon resources, such as OWPs (Peltre et al., 2012; Paetsch et al., 2016).
The implementation of selected agroecological practices and systems such as conservation agriculture, agroforestry and OWP application allows for additional carbon storage in soils (Peltre et al., 2012; Autret et al., 2016; Paetsch et al., 2016; Pellerin et al., 2019; Bohoussou et al., 2022). The additional carbon storage linked to agricultural practice B (any agroecological practice) is the difference between the carbon stock in a soil under practice B and that of the same soil under a reference practice (Pellerin et al., 2019). This additional carbon storage is not necessarily only the result of recent carbon inputs, but can also include the legacy carbon. However, knowledge on the biogeochemical stability of this additional carbon is lacking, questioning the reversibility of this storage. The carbon sink effect will indeed be more effective if the additional carbon storage is realized in the form of persistent organic carbon (OC) and not in the form of labile OC. We propose that the biogeochemical stability of additional organic carbon is evaluated and compared following implementation of various agroecological practices.
Several methods have been reported in the literature to assess the organic carbon temporal stability in soils. These methods isolate kinetic pools or carbon fractions with contrasting mean residence times (MRTs) – e.g. particle size fractionation (Balesdent, 1996), density fractionation (Sollins et al., 2006), sequential extraction (Heckman et al., 2018), thermal analysis (Barré et al., 2016) and incubation (Schädel et al., 2020). Physical fractionation is probably the most used method so far to evaluate SOM stability. Physical fractionation methods isolate fractions based on size, density or a mixture of both (Chenu et al., 2015). In a study comparing several fractionation methods, Poeplau et al. (2018) found that particle size fractionation was well suited for isolating particulate organic matter (POM) fractions from mineral-associated organic matter (MAOM) with contrasting MRT. Fractionation of SOM into POM and MAOM components can reveal insights about the sources and stability of SOC (Kim et al., 2022). However, some studies have shown that SOC fractionation methods fail to accurately separate stable SOC from active SOC and, in particular, that the isolated MAOM fractions are mixtures of labile SOC (MRT of months to years) and stable centennial SOC (Balesdent, 1987; Jastrow et al., 1996; Sanderman et al., 2013; Torn et al., 2013; Balesdent, 1996; Hsieh, 1992; von Lützow et al., 2007; Sanderman and Grandy, 2020). This may be due to methodological challenges as well as the fact that there are multiple pathways for SOM formation and stabilization (Cotrufo et al., 2013; Sokol et al., 2019).
Thermal analysis techniques, long-used in petroleum exploration and clay mineralogy, offer a promising alternative to or complement physical and chemical fractionation methods and are being increasingly applied to studies of SOC stability (Peltre et al., 2013; Plante et al., 2009). Indeed, several parameters obtained using thermal analysis are strongly related to SOM biogeochemical stability (Barré et al., 2016; Poeplau et al., 2019). However, these parameters do not allow us to separate the kinetic carbon pools (Schiedung et al., 2017). And so, recently, Cécillon et al. (2018, 2021) developed a machine learning model, called PARTYSOC, which shows that Rock-Eval® (RE) parameters can be used to predict the fraction of SOC that is stable at a centennial timescale. Kanari et al. (2022) evidenced that SOC fractions calculated using PARTYSOC matched the stable (MRT>100 years) and active (MRT∼30–40 years) OC pools of the AMG (Andriulo, Mary and Guérif) model, a model widely known to simulate SOC stock evolution in French and European croplands (Clivot et al., 2019; Bruni et al., 2022). As a result, one can consider that a Rock-Eval® analysis associated with the PARTYSOC model allows for the quantification of carbon fractions that are stable at a centennial timescale and active sensu the AMG model.
The incubation method is, however, the only direct test for the biological stability of SOC that results from chemical resistance to decomposition and/or organomineral associations and/or inaccessibility of organic substrates to microbial decomposition. Long-term incubations (months to years in length) may diverge from the conditions that prevail in the soil profile but provide insights into the potential decomposability of slower-cycling SOC (e.g. Schädel et al., 2014). In early laboratory incubations, fast-cycling C respiration dominates total respired SOC but rapidly declines, while slow-cycling SOC accounts for most of the respired SOC after the fast SOC pool is depleted.
These different methods do not separate similar carbon kinetic pools. Indeed, the incubation method isolates carbon, with MRT ranging from days to years (Schädel et al., 2014) while others isolate carbon with longer MRT (decades to centuries) (Cécillon et al., 2018, 2021; Balesdent, 1996). A multi-method approach will thus further improve our knowledge of the biogeochemical stability of SOC in the short (<2 years), medium (2–50 years) or long term (>50 years). The objective of this study was to evaluate the biogeochemical stability of additional SOC stored after the implementation of carbon-storing agroecological practices using a multi-method approach. To do so, we characterized SOM using particle size and density fractionation, Rock-Eval® (RE) thermal analysis and incubation in soil from plots managed using various agroecological practices, such as the addition of OWPs (composts and farmyard manure) and alternative cropping systems including no tillage, permanent cover crop and the introduction of legumes to the rotation. The application of OWPs is likely to provide organic matter (OM) that has been pre-stabilized by the storage (manure) or composting process and is hence less decomposable than the fresh matter provided by plant biomass in alternative cropping systems. Then, we hypothesized that (i) the biogeochemical stability of additional SOC depends on the management practices implemented; (ii) the additional SOC originating from OWPs would be more stable than that directly originating from plant biomass; and (iii) overall, however, the additional SOC would be less stable than the SOC stored in the conventional controls.
2.1 Field site and soil sampling
This study focuses on two French long-term experiments (LTEs) developed on Luvisols in the same region, where agroecological practices including conservation agriculture, organic agriculture and OWP application (composts and manure) were implemented.
La Cage experiment. This experiment is conducted in Versailles (48°48′ N, 2°08′ E; 120 m in altitude). During the 21 years of experimentation, the mean annual temperature and precipitation were 11.6 °C and 633 respectively. The soil is a well-drained deep Luvisol (IUSS Working Group WRB, 2015). The field experiment is arranged in a randomized complete block design and divided into two blocks, themselves divided into four plots for each cropping system. Each plot is divided into two subplots of 0.56 ha so that two different crops of the crop rotation are present each year, with wheat being grown every year in one of the two subplots (Autret et al., 2020). A detailed presentation of crop rotations, soil management and fertilization were given by Autret et al. (2016). The 4-year crop rotation mainly consisted of rapeseed (Brassica napus L.), winter wheat (Triticum aestivum L.), spring pea (Pisum sativum L.) and winter wheat.
-
Conventional agriculture (CON-LC) is characterized by a soil and crop management representative of the Paris Basin cereal production, with annual soil ploughing, absence of organic amendment, mineral N fertilization (average rate of 143 ) and systematic use of pesticides.
-
Conservation agriculture (CA) includes a permanent soil cover, initially fescue (Festuca rubra) and, since 2008, alfalfa, grown under all the main crops except pea. In the rotation, rapeseed is replaced by maize (Zea mays L.) in CA and direct seeding is performed.
-
Organic agriculture (ORG) is characterized by alfalfa–alfalfa–wheat–wheat rotation, with annual soil ploughing and no synthetic fertilizers nor pesticides.
The QualiAgro experiment. The site of this experiment is located in Feucherolles, 20 km west of Versailles (48°52′ N, 1°57′ E; 150 m in altitude). During the 21 years of experimentation, the mean annual temperature and precipitation were 11.0 °C and 614 respectively. The soil is a Luvisol (IUSS Working Group WRB, 2015). The crop rotation mainly consisted of wheat and maize (Peltre et al., 2012). It is a field experiment conducted in collaboration with the National Research Institute for Agriculture, Food and the Environment (INRAE) and Veolia Environment Research and Innovation (VERI) since 1998, during which composts of OWPs are applied every 2 years for a dose equivalent to ∼4 t C ha−1 from 1998 to 2013 and ∼2 t C ha−1 from 2015 to 2020. The unit plots are 10 m×45 m in size. Each treatment has four replicates and OWPs are applied every 2 years on wheat stubble. Soils are ploughed every year on this experimental site. Since 2015, wheat and maize residues are buried in the soil. Four treatments are considered in this study.
-
Conventional agriculture (CON-QA) is characterized by a soil and crop management representative of the Paris Basin cereal, the absence of organic amendments, a mineral N fertilization (average rate of 167 ).
-
Biowaste compost (BIOW) is the compost of the fermentable fraction of selectively collected household waste, mixed with green waste.
-
Municipal solid waste compost (MSW) is the compost of the residual fraction of household waste after selective collection of packaging.
-
Farmyard manure (FYM) represents a reference amendment.
At both sites, four replicate plots were available per treatment. From each plot, three sub-samples were taken from the topsoil at 30 ± 1 cm (in September 2019 at QualiAgro and in November 2020 at La Cage), thoroughly mixed and combined into one sample. The samples were sieved to 4 mm and homogenized, the plant material was removed and the soil was oven-dried at 35 °C for 72 h before particle size and density fractionation and RE thermal analysis.
2.2 Calculation of SOC stocks and additional carbon stocks
SOC stocks were calculated at equivalent soil mass in both long-term experiments. Thus, at QualiAgro, the SOC stock was calculated by multiplying the SOC content by bulk density (data provided by QualiAgro) and was normalized to a depth of 10 cm (factor 10−3) (reference soil mass of 3963 kg ha−1). Bulk densities between 1998 and 2019 increased significantly in all plots. We calculated the additional soil thickness required to achieve this equivalent soil mass in treatments with lighter tilled layers as described by Ellert and Bettany (1995):
where Tadd is the additional thickness of the subsoil layer (in cm) needed to reach the equivalent soil mass and Msoil equiv is the equivalent soil mass of the denser horizon (in kg ha−1). In our study, the dense 0–29 cm layer was the reference treatment in 2019, with a bulk density of 1.37 g cm−3 giving an equivalent soil mass (Msoil equiv) of 3963 kg ha−1. Msoil topsoil is the soil mass in the surface (tilled) layer and ρbsubsoil is the bulk density of the underlying 29–35 cm layer (in g cm−3). Carbon stocks per hectare in equivalent soil masses (stock Cequiv) were calculated by adding the carbon stock in the surface layers (stock Ctopsoil) and in the additional underlying layers (stock CTadd) with the following formula:
In the La Cage experiment, the soil sampling strategy was designed to calculate SOC stocks on an equivalent soil mass (ESM) based on Ellert and Bettany (1995) over a depth at least equal to the deepest tillage event. The ploughing depth was around 30 cm before 1998 and shallower afterwards, at about 25 cm (Autret et al., 2016). The sample was taken at the depth equivalent to a soil mass of 4300 kg ha−1. The carbon stocks were calculated by multiplying the SOC content with this equivalent soil mass.
We then calculated the additional carbon storage (ΔSOC stock) considering each time the conventional control at La Cage (CON-LC) and the conventional control at QualiAgro (CON-QA). The following formula was used:
with stock CPractice being the carbon stock of the agroecological practice and stock Cconventional the carbon stock of the conventional control. The standard deviation used for the additional carbon stock was calculated based on the equation described by Kuzyakov and Bol (2004) as follows:
2.3 Particle size and density fractionation
The method uses a preliminary disaggregation aiming at the best compromise between maximum destruction of micro-aggregates of a size <50 µm and respect of the integrity of organic debris (Balesdent et al., 1991) and combines fractionation by particle size to separate POM from OM associated with clays and silt minerals with water flotation to separate POM from sands. For this purpose, approximately 50 g of soil was suspended in 180 mL of 0.5 % sodium hexametaphosphate (SHMP) saline solution in a 250 mL polyethylene bottle; 10 glass beads were added and the whole set was brought to agitation by inversion (REAX 2-type inversion mixers) for 16 h at a speed of approximately 50 rpm to destroy the aggregates. The SHMP solution and the glass beads allow for the complete dispersion of soil aggregates that are >50 µm diameter in these soils (Balesdent et al., 1991). After agitation, the suspension was first sieved on a 200 µm sieve from which the refusal, i.e. the coarse fraction, was recovered in a 250 mL glass beaker. We separated the coarse POM (cPOM) from the coarse sands (cSand) by flotation in water. The suspension <200 µm was submitted to sieving a second time at 50 µm and the same operations were performed to separate the fine POM (fPOM) from the fine sands (fSand) using water flotation. The suspension <50 µm is submitted to ultrasounds by imposing onto it an energy of 300 J mL−1 necessary to disperse the micro-aggregates (Balesdent et al., 1998). After this step, we sieved the suspension <50 to 20 µm to recover the coarse silts of a size between 20 and 50 µm (cSilt) remaining on the sieve. The suspensions containing particles <20 µm were pooled in a 2 L beaker. The separation of the fine silts between 2 and 20 µm (fSilt) from the clays was performed by centrifuging the <20 µm filtrate at 64 g (circa 500 rpm) for 10 min. The supernatant containing the clays was collected in a 5 L beaker. The same process was repeated four to five times by resuspending the pellet for an optimal recovery of fine silts by decantation. The supernatant collected in the 5 L beaker constitutes the Clay fraction (<2 µm) and the pellet after repeated centrifugation constitutes the fine silt fraction. To reduce the volume of the clay suspension to be freeze-dried, we added CaCl2 to flocculate the clay particles, and by centrifugation for 20 min at 16 000 g (circa 8000 rpm), we recovered the pellet which constitutes the Clay fraction (Fig. 1). An aliquot of the supernatant was taken to determine the dissolved organic carbon (DOC). The particle size and density fractionation resulted in recovery rates of 95 % ± 2 % of the initial sample mass and 98 % ± 6 % of carbon.
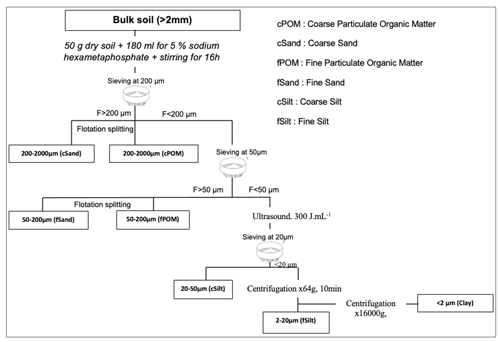
Figure 1Particle size and density fractionation protocol (adapted from Balesdent et al., 1998). The POM fraction is the sum of the cPOM, fPOM, cSand and fSand fractions, while the MAOM fraction is the sum of the cSilt, fSilt and Clay fractions.
2.3.1 Fractions preparation and elemental analysis (C, N)
The fractions obtained during this fractionation were dried or freeze-dried. The organic (cPOM and fPOM) and mineral (cSand, fSand and cSilt) fractions were oven-dried at 50 °C for 3 d, while the fine silt (fSilt) and clay (Clay) fractions were freeze dried. Each fraction was weighed and C and N were determined using dry combustion (elemental analyser, elemental vario ISOTOPE).
2.4 Rock-Eval® (RE) thermal analysis
We analysed 28 samples of bulk soil using a RE6 Turbo apparatus (Vinci Technologies). A small amount of soil (about 60 mg) was required for the analysis, which was performed in two consecutive steps during which carbon-containing effluents were directly detected. First, the sample underwent pyrolysis in an inert atmosphere (N2) followed by oxidation in the presence of O2 (ambient air). The heating routine applied during pyrolysis was that proposed by Disnar et al. (2003) and Baudin et al. (2015), including a 3 min isotherm at 200 °C, which was followed by a 30 °C min−1 heating ramp to 650 °C. Oxidation began with a 1 min isotherm at 300 °C followed by a 20 °C min−1 heating ramp to 850 °C and a final 5 min isotherm at 850 °C (oxidation routine presented in Baudin et al., 2015, as the “bulk rock/basic” method). Simultaneous detection of effluents during both analytical steps generated a total of five thermograms per sample, describing the evolution of hydrocarbons during pyrolysis (HC_PYR) and CO and CO2 during both pyrolysis and oxidation steps (CO_PYR, CO2_PYR, CO_OX and CO2_OX).
2.4.1 Rock-Eval® parameters
The classical Rock-Eval® parameters were acquired using the Rocksix software (Vinci Technologies) with a good reproducibility (Pacini et al., 2023). They include six automatically generated “peaks” defined as specific areas of the three pyrolysis thermograms (S1, S2, S3, S3′, S3CO and S3′CO; Lafargue et al., 2018), the amount of pyrolized carbon (PC corresponding to the sum of organic C released as HC, CO and CO2 during pyrolysis), total organic carbon (TOC corresponding to the amount of organic C released during analysis), inorganic carbon (MinC, corresponding to the amount of C released from carbonate cracking), hydrogen index (HI corresponding to the ratio of hydrocarbons released to TOC) and oxygen index (OIRE6 corresponding to the ratio of organic oxygen released to TOC). In addition, other parameters used as predictors by the PARTYSOCv2.0EU model were calculated based on thermograms obtained using R scripts available on Zenodo (https://zenodo.org/record/4446138#.YDe84Xlw2SQ, last access: December 2021) (Cécillon et al., 2021; Kanari et al., 2021). These include PseudoS1 (the sum of carbon released during the first 200 s of isothermal 200 °C pyrolysis as HC, CO, and CO2); the S2PC ratio (the ratio of the amount of hydrocarbons released excluding the first 200 s of pyrolysis to the pyrolyzed carbon); the PCTOC ratio; the HIOIRE6 ratio; and 10 temperature parameters (e.g. T30, T50, T70 and T90) that describe the evolutionary steps, i.e. at what temperature 30 %, 50 %, 70 % and 90 % of a given gas was released. A detailed description of the definition, units, and equations used to calculate all parameters can be found in the study of Kanari et al. (2021). The HI and OIRE6 are commonly reported indices that represent proxies for the SOM and ratios respectively.
2.4.2 PARTYSOC model based on Rock-Eval® (RE)
In this study, we used the random forest model based on RE results of PARTYSOCv2.0EU (https://zenodo.org/record/4446138#.YDe84Xlw2SQ) proposed by Cécillon et al. (2021). This model was calibrated on data from six long-term agricultural experiments, including a bare fallow treatment in northwestern Europe and can predict the proportion of persistent SOC at a centennial timescale in topsoil samples (0–30 cm). The model requires a set of 18 RE parameters (e.g. Kanari et al., 2021) characteristic of a sample and provides a prediction of the proportion of stable SOC for soils from the La Cage and the QualiAgro long-term experiments. The 18 RE parameters retained were the RE temperature parameters T70HC_PYR, T90HC_PYR, T30CO2_PYR, T50CO2_PYR, T70CO2_PYR, T90CO2_PYR, T70CO_OX, T50CO2_OX, T70CO2_OX and T90CO2_OX and the RE parameters PseudoS1, S2, S2 / PC, HI, HI / OIRE6, PC, PC / TOCRE6 and TOCRE6 (Cécillon et al., 2021).
2.5 Long-term incubation
Polyvinyl chloride (PVC) cylinders that are 5.7 cm in diameter and 4 cm in height with 2 mm perforations were used to build soil microcosms. A 50 µm mesh fabric at the bottom of the cylinder supported the soil while promoting gas exchange. Each cylinder was weighed empty and then with fresh soil equivalent to 100 g of dry soil. The soil samples were then brought to a bulk density of 1.3 g cm−3 with a hand press and mold. Knowing the initial water content, the samples were gradually brought to the water content corresponding to a soil matrix potential of pF 2.5 by adding water with a Pasteur pipette. Then, the microcosms were mounted in 0.5 L jars. The soil cylinders were placed on PVC racks and 15 mL of water was added to the bottom of the jars to stabilize the humidity. The jars were sealed and the whole set was placed in the incubator at 20 °C for 1 week before incubation. Four replicates per agricultural practice were prepared.
After the pre-incubation period, we readjusted the water content of the soil cylinders to a pF of 2.5 when necessary. A total of 28 soil cylinders were incubated for 484 d under the same temperature (20 °C) and moisture (pF of 2.5) conditions.
2.6 Mineralization measurement
Soil organic carbon (SOC) mineralization in samples from both long-term experiments (LTEs) was measured nondestructively using a gas microchromatograph (μGC 490; Agilent Technologies, USA). Measurements were performed at days 1, 3, 7, 14, 28 and 35, then one measurement was performed every 2 weeks until the sixth month, and, finally, one measurement was performed every month until the end of incubation. The CO2 emitted is measured in parts per million (ppm). It is then converted to µg C–CO2 g−1 of dry soil using the following formula (Védère et al., 2020):
where CO2 (ppm) is the amount of CO2 emitted measured by gas-phase microchromatography, Mc the molar mass of carbon (in g mol−1), Vb the volume of the jar (in L), VM the molar volume of the gas (in L mol−1) and Msoil the mass of the incubated dry soil (in g). The absolute amount of carbon mineralized was expressed per unit of SOC to obtain the specific SOC mineralization in , i.e. %SOC mineralized (Kpemoua et al., 2023). To calculate the amount of additional carbon mineralized over the 484 d, we first calculated the difference in absolute carbon mineralization between the agroecological practice and the conventional control. We assume that the extra absolute carbon mineralized in the agroecological practice relative to the conventional control comes from the additional carbon. Given the amount of additional carbon (ΔSOC), we then expressed this extra absolute carbon mineralization in terms of additional carbon (%ΔSOC).
2.7 Statistical analysis
All data were tested for normality and homogeneity of variance. A log transformation was applied to the data for the cSand and fSand fractions as the transformation improved the normality and variance substantially. A one-way ANOVA was used to detect significant differences at the 5 % threshold in bulk soil carbon stocks, fractions, carbon pools and amount of carbon mineralized (Cmin). Once a significant difference was detected, Tukey's multiple comparison test was used to compare carbon stocks, additional carbon stocks, percentage of total carbon storage and percentage of additional carbon storage in either bulk soil, fractions or carbon pools according to agricultural practices. All statistical analyses were completed in R (version 4.0.2).
3.1 SOC stocks
The application of organic waste products (OWPs), increased soil organic carbon (SOC) content in soils by 64 % in biowaste compost treatment (BIOW), 40 % in farmyard manure treatment (FYM) and 39 % in residual solid waste compost treatment (MSW) compared to the conventional control (CON-QA); at the same time, at La Cage, the implementation of organic agriculture (ORG) and conservation agriculture (CA) increased SOC contents by 6 % and 35 % respectively relative to conventional control (CON-LC) (Table 1). The OWP application significantly increased carbon stocks at QualiAgro. SOC stocks were in the following order: BIOW > FYM ≥ MSW > CON-QA (Table 1). At La Cage, SOC stocks were in the following order: CA > ORG ≥ CON-LC (Table 1).
Table 1Soil organic carbon (SOC), soil organic nitrogen (SON) and measured in topsoil. Values in brackets are standard deviations. CON-QA: conventional agriculture without organic inputs, MSW: municipal solid waste compost, FYM: farmyard manure, BIOW: biowaste compost, CON-LC: conventional agriculture, ORG: organic agriculture and CA: conservation agriculture.
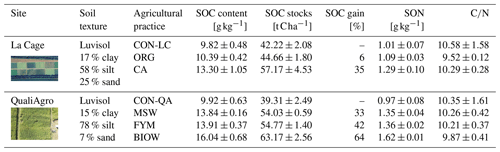
3.2 SOC distribution in fractions
The mass proportion, carbon content and percentage of carbon distribution of the physical fractions after particle size and density fractionation are presented in Tables S1 and S2 in the Supplement. The distribution of SOC stocks over the fractions obtained, expressed in t C ha−1, is given in Fig. 2a and b. Carbon distribution in conventional controls (CON-LC and CON-QA) showed that 19 %–22 % of carbon was found in POM fractions, while 78 %–81 % was found in MAOM fractions (Fig. 4a and b). Overall, most of the organic carbon was located in the Clay fraction (64 %–72 % SOC; see Tables S1 and S2) regardless of the site and agricultural practice implemented. The carbon distribution in QualiAgro indicated a significant increase in SOC stocks in the cSand, fPOM, cSilt and Clay fractions after OWP application (p<0.05), while no significant difference was observed in the cPOM, fSand and fSilt fractions (p>0.05). In La Cage, the implementation of conservation agriculture significantly increased SOC stocks as fPOM and Clay fractions compared to organic and conventional agriculture, which remained statistically equal.
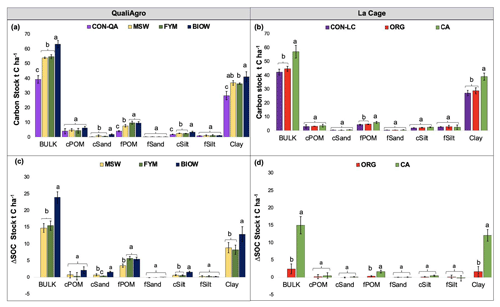
Figure 2Soil organic carbon stock and additional carbon (ΔSOC) stock of bulk soils and physical fractions (n=4) at QualiAgro and La Cage experiments. The error bars represent the standard deviations. Grouped bars with different letters are significantly different between agricultural practices (Tukey HSD; p<0.05). CON-QA: conventional agriculture without organic inputs, BIOW: biowaste compost, MSW: municipal solid waste compost, FYM: farmyard manure, CON-LC: conventional agriculture, CA: conservation agriculture and ORG: organic agriculture. The POM fraction is the sum of the cPOM, fPOM, cSand and fSand fractions, while the MAOM fraction is the sum of the cSilt, fSilt and Clay fractions.
We calculated the distribution of additional carbon (ΔSOC) in the fractions as the difference between the carbon stock of the bulk soil or physical fractions under agroecological practices and the carbon stock of the bulk soil or physical fractions under conventional control (CON-QA or CON-LC respectively for the QualiAgro and the La Cage experiments). The additional carbon stock at QualiAgro was 23.86 ± 1.79 t C ha−1 in BIOW compared to 15.46 ± 1.43 t C ha−1 in FYM and 14.72 ± 1.28 t C ha−1 in MSW (Fig. 2c). At La Cage, the additional SOC stock was 14.95 ± 2.49 t C ha−1 in CA compared to 2.44 ± 1.38 t C ha−1 in ORG (Fig. 2d). In terms of percentage, we observed that the coarse mineral fractions (cSand and fSand) have a negligible proportion of additional carbon at La Cage, representing 1 % in CA and 0 % in ORG, while this proportion was raised to 2 % in FYM, 5 % in MSW and 7 % in BIOW at QualiAgro. This non-negligible proportion of carbon in the sand at QualiAgro suggests that probably not all particulate organic matter (POM-C) has been isolated from the sand. Hence, in the following, to define the POM-C fraction class, we combine the fractions of cPOM, fPOM, cSand and fSand. Thus, we observed in the QualiAgro experiment that 60 %–66 % of the additional carbon was localized in mineral-associated organic matter fractions (MAOM-C), which included the cSilt, fSilt and Clay fractions, versus 34 %–40 % in POM-C, whereas in the La Cage experiment, 77 %–84 % of the additional SOC stock was located in the MAOM-C versus 16 %–23 % in the POM-C. Furthermore, among practices with equivalent additional carbon stocks (MSW, FYM and CA), OWP application resulted in a higher proportion of additional carbon in POM-C (34 % for MSW; 40 % for FYM) compared to CA (16 %).
3.3 Estimating stable and active SOC pools with the PARTYSOC model
The PARTYSOC machine learning model was used to estimate the proportion of stable SOC under the different managements. The distribution of organic carbon stocks in the active and stable pools is shown in Fig. 3. In conventional controls, 38 %–43 % of the soil carbon is found in the active pool, whereas 57 %–62 % is found in the stable pool. The organic waste product (OWP) application significantly increased the size of the active pool relative to the conventional control (Fig. 3a; ANOVA; p<0.05). It was 31.87 ± 2.23 t C ha−1 in BIOW compared to 29.62 ± 1.97 t C ha−1 in FYM and 26.97 ± 1.07 t C ha−1 in MSW and 16.76 ± 1.69 t C ha−1 in CON-QA. The OWP application significantly increased the size of the stable SOC pool in the BIOW (31.29 ± 0.91 t C ha−1) and MSW (25.15 ± 1.36 t C ha−1) treatments compared to the FYM (25.15 ± 1.44 t C ha−1) and CON-QA (22.55 ± 1.31 t C ha−1), which were statistically similar. Contrastingly, in the La Cage experiment, 20 years of contrasted management had no significant effect on the size of the stable SOC pool (28.02 ± 2.95, 26.31 ± 0.93 and 26.08 ± 1.89 t C ha−1 for CA, ORG and CON-LC respectively). However, CA significantly increased the size of the active pool (29.15 ± 5.79 t C ha−1) compared to ORG and CON-LC, in which it was similar (18.35 ± 3.47 and 16.14 ± 0.97 t C ha−1 respectively) (Fig. 3b).
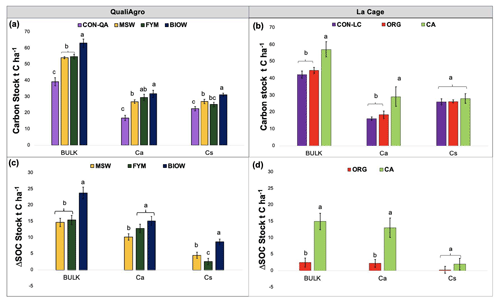
Figure 3Soil organic carbon stock and additional carbon (ΔSOC) stock of bulk soils, active carbon (Ca) and stable carbon (Cs) (n=4) at QualiAgro and La Cage experiments. The error bars represent the standard deviations. Grouped bars with different letters are significantly different between agricultural practices (Tukey HSD; p<0.05). CON-QA: conventional agriculture without organic inputs, BIOW: biowaste compost, MSW: municipal solid waste compost, FYM: farmyard manure, CON-LC: conventional agriculture, CA: conservation agriculture and ORG: organic agriculture.
The results of additional carbon (i.e. the difference between the active or stable carbon pool of agroecological practices and the active or stable carbon pool of conventional control) distribution in the active and stable carbon pools are shown in Fig. 3c and d. In the QualiAgro experiment, BIOW (15.85 ± 1.48 t C ha−1) and FYM (13.36 ± 1.16 t C ha−1) had a similar active carbon pool size which was higher than in the MSW (12.34 ± 0.75 t C ha−1). This active pool represented 63 %–83 % of the additional carbon storage (Fig. 4c). Additional stable carbon pools were ordered as follows: BIOW (8.74 ± 0.79 t C ha−1) > MSW (4.51 ± 0.94 t C ha−1) > FYM (2.60 ± 0.97 t C ha−1) and represented between 17 % (FYM) and 37 % (BIOW) of the additional carbon. At La Cage, 87 % (CA) to 91 % (ORG) of the additional carbon was in the active pool versus 9 % (ORG) to 13 % (CA) that was in the stable pool (Fig. 4d).
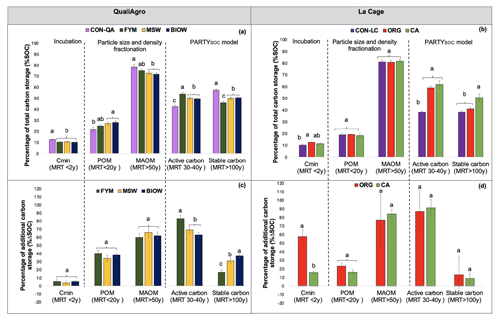
Figure 4Distribution of total carbon and additional carbon in carbon kinetic pools (Cmin, carbon mineralized); active; and stable carbon) or fractions (POM and MAOM) under agricultural practices. The error bars represent the standard errors. Grouped bars with different letters are significantly different between agricultural practices (Tukey HSD; p<0.05).
3.4 Carbon mineralization kinetics
At the end of soil incubation (day 484), the cumulative amounts of mineralized carbon expressed as percent soil organic carbon (% SOC) in the La Cage experiment differed significantly between the three cropping systems, i.e. 12.60 % ± 0.29 % SOC was in ORG compared to 11.52 % ± 1.19 % SOC in CA and 10.21 % ± 1.36 % SOC in CON-LC (Fig. 4b). In the QualiAgro experiment, the specific carbon mineralization kinetics were significantly higher on the conventional control (CON-QA) without organic inputs compared to the soils receiving OWPs, where the mineralization carbon of the MSW and FYM plots were statistically identical but higher than the BIOW plot (Fig. 4a).
Overall, these two experiments show opposite trends: on the one hand, higher carbon mineralization under agroecological practices (ORG and CA) in the La Cage experiment relative to the conventional control (CON-LC), and on the other hand, lower carbon mineralization under agroecological practices (MSW, FYM and BIOW) in the QualiAgro experiment relative to the conventional control (CON-QA). Moreover, the percentage of additional carbon mineralized (%ΔSOC) at La Cage in CA (15 % of ΔSOC) and ORG (57 % of ΔSOC) was higher than at QualiAgro (4 %–5 % of ΔSOC) (Fig. 4c and d). It must be noted, however, that the additional carbon stock was very small in the ORG treatment, which numerically explains the high %ΔSOC calculated value.
4.1 Both POM-C and MAOM-C are sensitive to management
The observed distribution of SOC in the fractions, i.e. most of the SOC (70 %–80 %) being located in the fine fraction (< 50 µm), regardless of the agricultural practice implemented (Fig. 4a and b), is in agreement with the literature (Christensen, 1985, 1987; Balesdent, 1996; Baldock and Skjemstad, 2000; Jolivet et al., 2003; Carter et al., 2003; Beare et al., 2014; Curtin et al., 2016; Poeplau et al., 2018).
Many studies have indicated that the POM-C fraction is more sensitive to land use and management changes than the MAOM-C fraction (Cambardella and Elliott, 1992; Elliott et al., 1994; Bayer et al., 2001; Salvo et al., 2010). However, our study showed that both POM-C and MAOM-C fractions were highly sensitive to the implementation of agroecological practices for during a period of 20 years. The application of OWPs resulted in additional soil organic carbon as both POM-C (34 %–40 % of ΔSOC) and MAOM-C (60 %–66 % of ΔSOC), while conservation and organic agriculture resulted in additional organic carbon mainly as MAOM-C (77 %–84 % of ΔSOC) and less so as POM-C (16 %–23 % of ΔSOC). A significant proportion of the additional carbon is associated with soil minerals, particularly in the Clay fraction (see Tables S1 and S2).
Studies comparing no-till compared to conventional tillage management in the surface layer showed an increase in the POM-C fraction with no tillage and no difference in MAOM-C (Wander et al., 1998; Hussain et al., 1999; Carbonell-Bojollo et al., 2015; Samson et al., 2020). A recent meta analysis by Prairie et al. (2023) indicated that no tillage increased both POM-C and MAOM-C fractions in soils when this practice was maintained for up to 6 years. However, the increase in the MAOM-C fraction was less important than that of the POM-C fraction. We therefore ascribe the observed increase in MAOM-C at La Cage to no tillage and the introduction of cover crops and the diversification of species (e.g. legumes) in the rotation. Interestingly, an earlier analysis of SOC distribution at La Cage after 5 years of differentiation showed a significant increase in POM-C in the conservation agriculture system, while there was no change in POM-C in the organic system and no significant change in MAOM-C (Balabane et al., 2005), suggesting either that it took more than 5 years for the additional POM-C to be broken down and biodegraded as MAOM-C or that the introduction of alfalfa as the cover crop instead of fescue since 2008 (i.e. 12 years later) resulted in more direct rhizodeposits inputs to MAOM-C. Indeed, according to Autret et al. (2016), the estimated inputs from fescue were lower (0.88 ) than those coming from alfalfa as a cover crop (1.12 ), with about half of these amounts deriving from root material. The cover crops and legume rotation in conservation agriculture and the legume rotation in organic agriculture would likely have affected carbon input via the root system as dead roots (POM) and rhizodeposits (MAOM). This would explain the high proportion of carbon associated with MAOM-C. Typically, the cover crops characterized by low litter quality (e.g. grass) resulted in a higher accumulation of POM that was abundant in plant carbon, while cover crops with high litter quality (e.g. legumes) contributed to a higher accumulation of SOC (Cotrufo et al., 2013), and of microbial necromass carbon (Zhang et al., 2022) in MAOM-C. Thus, the high proportion of carbon in the MAOM-C at La Cage (77 %–84 % of ΔSOC) compared to QualiAgro (60 %–66 % of ΔSOC) could be explained by the type and quality of the carbon input. Because the cover crops increase the time period during which plant roots interact with the soil environment (Tiemann et al., 2015), they deliver an additional source of root litter and exudates, providing greater diversity in belowground inputs (Austin et al., 2017). This promotes the microbial growth and turnover in rhizosphere hotspots, processes that can enhance the formation of MAOM (Kallenbach et al., 2016).
The recent meta analysis conducted by Zhang et al. (2022) indicated that the application of OWPs significantly increases both MAOM and POM fractions in the soil relative to the control treatment, which is consistent with our results. Peltre (2010) observed that the short-term application (four times) of the OWPs at QualiAgro increased the additional carbon only in the POM-C fraction, the MAOM-C fraction <50 µm remains unchanged. Paetsch et al. (2016) later found that seven successive applications of the OWPs led to additional carbon in occluded small POM (<20 µm) and in the fine silt–clay fraction (<6.3 µm). After 11 applications of OWPs, we observed an increase in fine POM (50–200 µm), coarse silt (20–50 µm) and MAOM-C (<50 µm). This series of results indicates that the application of the OWPs increases the POM-C fraction in the short term and that in the longer term (>10 years), the organic carbon in the POM-C is transferred to MAOM-C through biological activity in the soil. The transfer of additional carbon from POM to MAOM is, however, slower at QualiAgro compared to La Cage. Cotrufo et al. (2015) show that POM and MAOM likely form under different biochemical and physical pathways. While there is certainly some transfer from POM to MAOM, much of MAOM is formed from dissolved organic matter (DOM) early during decomposition. There might just be a great abundance of DOM and labile inputs to La Cage to explain greater MAOM formation.
4.2 POM heterogeneity can hamper SOC stability assessments
The POM in this study consists of crop residues and/or added manure or composts and microbial residues. The agroecological practices with equivalent additional carbon stocks (MSW, FYM and CA) showed, after 20 years, a higher proportion of additional carbon in POM-C under MSW (40 % of ΔSOC) and FYM (34 % of ΔSOC) conditions compared to CA (16 % of ΔSOC). These results show that it is likely that different management (e.g. OWP application, no tillage, cover crops and legume) alter the way gross organic carbon inputs were distributed among the different organic carbon fractions. These results can be explained by the fact that the decomposition rate of organic amendments and the SOC formed and remaining in the long term vary according to the intrinsic quality of the amendment (Lashermes et al., 2009). For example, Paustian et al. (1992) observed that the high lignin content of FYM, which was more recalcitrant to decomposition, resulted in greater accumulation of C than lower-lignin amendments, such as straw. Previous studies demonstrated that the OWPs generally are partially stabilized by the composting and storage processes (Benbi and Khosa, 2014), unlike plant biomass, which is fresh OM.
The incubations revealed that in the La Cage experiment, a higher percentage of the additional carbon was mineralized in conservation agriculture (15 % of ΔSOC) over 484 d than additional carbon at QualiAgro (4 %–5 % of ΔSOC) (Fig. 4c and d). The low additional carbon mineralization at QualiAgro raises questions about the degradability of POM derived from OWPs, which were in higher proportion (34 %–40 % of ΔSOC) than in the La Cage experiment (16 %–23 % of ΔSOC). It is therefore likely that the OWP-derived POM was more recalcitrant with higher mean residence times compared to plant-derived POM. The mean residence time of <20 years given to POM in the study by Balesdent (1996) may not be applicable to systems where pre-treated exogenous OM is applied as the POM separated in the Balesdent (1996) study results from situations where the organic input was crop residues. Thus, we assume a greater chemical recalcitrance of POM-C in plots receiving OWPs, thereby reducing decomposer activity and carbon transfer to the fine soil fraction (<50 µm).
4.3 Different methods provide a contrasted evaluation of biogeochemical stability
We used different methods to assess the biogeochemical stability of the additional carbon stored in soil thanks to specific management options. The incubation method isolates carbon with the mean residence time (MRT) ranging from days to years (MRT<2 years in this case), while particle size and density fractionation isolates carbon fractions, with MRT ranging from years to decades (POM with MRT<20 years and MAOM with MRT>50 years), and the PARTYSOC model based on RE thermal analysis that isolates carbon pools, with the MRT ranging from decades to centuries (active pool with MRT ∼30–40 years and stable pool with MRT>100 years).
In the QualiAgro experiment, the incubations results indicate a greater stability of additional carbon compared to bulk SOC in the conventional control (i.e. lower specific carbon mineralization for soils receiving OWPs relative to CON-QA). However, the results of particle size and density fractionation and PARTYSOC based to RE thermal analysis indicate that the additional carbon stored by OWP application is, on average, less stable than the soil carbon in the conventional control (CON-QA). This is because in these plots, the additional carbon has a higher proportion of POM (MRT<20 years) and active carbon (MRT∼30–40 years) than the conventional control (Fig. 4a and c). As the incubations target carbon with MRT of the order of incubation length (i.e. MRT<2 years in this study), we posit that this difference is due to the fact that the different methods do not target the carbon pools with the same MRT. Put together, these results suggest that on the scale of a few decades, soil additional carbon in the QualiAgro experiment is less stable than soil carbon in conventional control but that in the shorter term (i.e. MRT<2 years), the additional carbon is quite resistant.
In the La Cage experiment, the results of the incubations and the PARTYSOC model based on RE thermal analysis are consistent and indicate that the additional carbon stored by conservation agriculture and organic agriculture is less stable than the soil carbon in the conventional control (CON-LC), whereas the particle size and density fractionation indicates a more stable additional carbon, i.e. a higher proportion of MAOM (MRT>50 years) than the conventional control (Fig. 4). However, the study by von Lützow et al. (2006) showed that MAOM does not have a unique mean residence time. For example, land use change (native and cropped lands) studies have indicated a decrease in carbon content in MAOM-C over time (Balesdent et al., 1998; Yeasmin et al., 2019). Lutfalla et al. (2019), using samples from 42 plots in Versailles, observed a decrease in carbon content in the Clay fraction (<2 µm) after 52 years of bare fallow conditions, thus questioning the long-term persistence of carbon associated with clays and MAOM-C. Our results provide evidence that at least part of the carbon contained in MAOM may not persist in soils over the long term, as shown by others previously (e.g. Balesdent, 1987; Keiluweit et al., 2015; Lutfalla et al., 2019; Chassé et al., 2021). We therefore hypothesize that the additional carbon stored in the form of MAOM has a lower MRT than the MAOM in the conventional control.
Based on these results, our hypothesis that the biogeochemical stability of additional carbon is less stable than the carbon in the conventional control is not always verified. However, considering that MAOM is kinetically heterogenous, the results of these methods can be reconciled. Therefore, the additional carbon is overall less stable at a decadal or pluri-decadal timescale than the carbon stored in the conventional control in both long-term experiments. Furthermore, taking all these elements and the complementary nature of the methods into consideration, it emerges that the additional carbon stored thanks to OWP application is more stable in the short (MRT<2 years) and long term (MRT>100 years) than the additional carbon enabled by alternative cropping systems but less so on the decadal and pluri-decadal timescale. The large timescale of SOM persistence shows that qualifying SOC simply as stable or labile is not sufficient. It is essential to always associate a temporality with the biogeochemical stability that is described in order to better assess the persistence of carbon in soils.
This study provided detailed information on the biogeochemical stability of additional carbon via a multi-method evaluation. Soils from the same experimental sites but under widely contrasting management have resulted in contrasting carbon contents and stocks after approximately 20 years of management. The results of particle size and density fractionation and the PARTYSOC model suggest that the additional carbon contained in MAOM may not persist in soils in the long term (>50 years). Incubation, on the other hand, provided information on the short-term stability of additional carbon (i.e. MRT<2 years). Overall, the multi-method evaluation showed that additional carbon was less stable on the decadal and pluri-decadal timescales than carbon under conventional controls. However, incubations and the PARTYSOC model based on RE thermal analysis revealed that additional SOC in the QualiAgro experiment was more stable in the short term (MRT<2 years) and long term (MRT>100 years) than that in the La Cage experiment. Additional SOC deriving from organic wastes, i.e. biomass that has been partially decomposed and transformed through its processing (digestion by cattle, storage and composting) prior to its incorporation in soil, would have a different biogeochemical stability than that deriving directly from plant biomass. Widely used methods (incubation and particle size fractionation) and increasingly used methods (RE) provide seemingly inconsistent assessments of the biogeochemical stability of SOC. These apparent contradictions can be explained by the fact that they address different kinetic pools of organic carbon. Care must be taken to specify which range of residence times is considered when using any method intending to evaluate the biogeochemical stability of SOM as well as when using the terms stable or labile. As we found that the additional SOC stored thanks to the implementation of different management options had contrasting biogeochemical stabilities, there is a need to evaluate the biogeochemical stability of the additional SOC stored via other management options (e.g. agroforestry, lengthening temporary leys and no tillage).
Data are available from the authors upon reasonable request.
The supplement related to this article is available online at: https://doi.org/10.5194/soil-10-533-2024-supplement.
TPIK, CC, PB and SH designed the study. TPIK performed soil fractionation and long-term incubations. FB and CP performed the RE6 thermal analyses and elementary analyses respectively. PB tested the R code for the PARTYSOC machine learning model. TPIK wrote the R codes and performed all statistical analyses. TPIK, CC, PB, SH and FB contributed to the interpretation of the results. CC acquired the funding. TPIK prepared the paper with contributions from all co-authors.
The contact author has declared that none of the authors has any competing interests.
Publisher's note: Copernicus Publications remains neutral with regard to jurisdictional claims made in the text, published maps, institutional affiliations, or any other geographical representation in this paper. While Copernicus Publications makes every effort to include appropriate place names, the final responsibility lies with the authors.
The QualiAgro site is conducted as a collaboration between INRAE and VERI. The authors thank the personnel from the Unité expérimentale Versailles Grignon INRAE and Michel Bertrand for the maintenance of and access to the La Cage long-term experiment and Fabien Ferchaud for the collaboration on La Cage SOC stock assessment. The authors also thank Valérie Pouteau for helping with incubation and fractionation and Florence Savignac for helping with Rock-Eval® thermal analyses.
This research has been supported by the French National Research Agency (StoreSoilC project; grant no. ANR-17-CE32-0005). Claire Chenu was also supported by the CLand programme (grant no. ANR-16-CONV-0003). Tchodjowiè P. I. Kpemoua was supported by ADEME. The QualiAgro field experiment forms part of the SOERE-PRO (network of long-term experiments dedicated to the study of impacts of organic waste product recycling), integrated as a service of Investments in the Future, AnaEE-France infrastructure, overseen by the French National Research Agency (grant no. ANR-11-INBS-0001).
This paper was edited by Ashish Malik and reviewed by two anonymous referees.
Austin, E. E., Wickings, K., McDaniel, M. D., Robertson, G. P., and Grandy, A. S.: Cover crop root contributions to soil carbon in a no-till corn bioenergy cropping system, GCB Bioenergy, 9, 1252–1263, https://doi.org/10.1111/gcbb.12428, 2017.
Autret, B., Mary, B., Chenu, C., Balabane, M., Girardin, C., Bertrand, M., Grandeau, G., and Beaudoin, N.: Alternative arable cropping systems: A key to increase soil organic carbon storage? Results from a 16 year field experiment, Agr. Ecosyst. Environ., 232, 150–164, https://doi.org/10.1016/j.agee.2016.07.008, 2016.
Autret, B., Guillier, H., Pouteau, V., Mary, B., and Chenu, C.: Similar specific mineralization rates of organic carbon and nitrogen in incubated soils under contrasted arable cropping systems, Soil Till. Res., 204, 104712, https://doi.org/10.1016/j.still.2020.104712, 2020.
Balabane, M., Bureau, F., Decaens, T., Akpa, M., Hedde, M., Laval, K., Puget, P., Pawlak, B., Barray, S., Cluzeau, D., Labreuche, J., Bodet, J. M., Le Bissonnais, Y., Saulas, P., Bertrand, M., Guichard, L., Picard, D., Houot, S., Arrouays, D., Brygoo, Y., and Chenu, C.: Restauration de fonctions et propriétés des sols de grande culture intensive. Effets de systèmes de culture alternatifs sur les matières organiques et la structure des sols limoneux et approche du rôle fonctionnel de la diversité biologique des sols (Dmostra), Rapport final de contrat MEDD 01105, Gestion durable des Sols, INRA, 119, 2005.
Baldock, J. A. and Skjemstad, J. O.: Role of the soil matrix and minerals in protecting natural organic materials against biological attack, Org. Geochem., 31, 697–710, https://doi.org/10.1016/S0146-6380(00)00049-8, 2000.
Balesdent, J.: The turnover of soil organic fractions estimated by radiocarbon dating, Sci. Total Environ., 62, 405–408, https://doi.org/10.1016/0048-9697(87)90528-6, 1987.
Balesdent, J.: The significance of organic separates to carbon dynamics and its modelling in some cultivated soils, Eur. J. Soil Sci., 47, 485–493, https://doi.org/10.1111/j.1365-2389.1996.tb01848.x, 1996.
Balesdent, J., Pétraud, J. P., and Feller, C.: Effet des ultrasons sur la distribution granulométrique des matières organiques des sols, Science du Sol, 29, 95–106, 1991.
Balesdent, J., Besnard, E., Arrouays, D., and Chenu, C.: The dynamics of carbon in particle-size fractions of soil in a forest-cultivation sequence, Plant Soil, 201, 49–57, https://doi.org/10.1023/A:1004337314970, 1998.
Barré, P., Plante, A. F., Cécillon, L., Lutfalla, S., Baudin, F., Bernard, S., Christensen, B. T., Eglin, T., Fernandez, J. M., Houot, S., Kätterer, T., Le Guillou, C., Macdonald, A., van Oort, F., and Chenu, C.: The energetic and chemical signatures of persistent soil organic matter, Biogeochemistry, 130, 1–12, https://doi.org/10.1007/s10533-016-0246-0, 2016.
Baudin, F., Disnar, J.-R., Aboussou, A., and Savignac, F.: Guidelines for Rock–Eval analysis of recent marine sediments, Org. Geochem., 86, 71–80, https://doi.org/10.1016/j.orggeochem.2015.06.009, 2015.
Bayer, C., Martin-Neto, L., Mielniczuk, J., Pillon, C. N., and Sangoi, L.: Changes in Soil Organic Matter Fractions under Subtropical No-Till Cropping Systems, Soil Sci. Soc. Am. J., 65, 1473–1478, https://doi.org/10.2136/sssaj2001.6551473x, 2001.
Beare, M. H., McNeill, S. J., Curtin, D., Parfitt, R. L., Jones, H. S., Dodd, M. B., and Sharp, J.: Estimating the organic carbon stabilisation capacity and saturation deficit of soils: a New Zealand case study, Biogeochemistry, 120, 71–87, https://doi.org/10.1007/s10533-014-9982-1, 2014.
Benbi, D. K. and Khosa, M. K.: Effects of Temperature, Moisture, and Chemical Composition of Organic Substrates on C Mineralization in Soils, Commun. Soil Sci. Plan., 45, 2734–2753, https://doi.org/10.1080/00103624.2014.950423, 2014.
Bohoussou, Y. N., Kou, Y.-H., Yu, W.-B., Lin, B., Virk, A. L., Zhao, X., Dang, Y. P., and Zhang, H.-L.: Impacts of the components of conservation agriculture on soil organic carbon and total nitrogen storage: A global meta-analysis, Sci. Total Environ., 842, 156822, https://doi.org/10.1016/j.scitotenv.2022.156822, 2022.
Bruni, E., Chenu, C., Abramoff, R. Z., Baldoni, G., Barkusky, D., Clivot, H., Huang, Y., Kätterer, T., Pikula, D., Spiegel, H., Virto, I., and Guenet, B.: Multi-modelling predictions show high uncertainty of required carbon input changes to reach a 4 ‰ target, Eur. J. Soil Sci., 73, e13330, https://doi.org/10.1111/ejss.13330, 2022.
Cambardella, C. A. and Elliott, E. T.: Particulate Soil Organic-Matter Changes across a Grassland Cultivation Sequence, Soil Sci. Soc. Am. J., 56, 777–783, https://doi.org/10.2136/sssaj1992.03615995005600030017x, 1992.
Carbonell-Bojollo, R., González-Sánchez, E. J., Ruibérriz de Torres, M. R., Ordóñez-Fernández, R., Domínguez-Gimenez, J., and Basch, G.: Soil organic carbon fractions under conventional and no-till management in a long-term study in southern Spain, Soil Res., 53, 113, https://doi.org/10.1071/SR13369, 2015.
Carter, M. R., Angers, D. A., Gregorich, E. G., and Bolinder, M. A.: Characterizing organic matter retention for surface soils in eastern Canada using density and particle size fractions, Can. J. Soil Sci., 83, 11–23, https://doi.org/10.4141/S01-087, 2003.
Cécillon, L., Baudin, F., Chenu, C., Houot, S., Jolivet, R., Kätterer, T., Lutfalla, S., Macdonald, A., van Oort, F., Plante, A. F., Savignac, F., Soucémarianadin, L. N., and Barré, P.: A model based on Rock-Eval thermal analysis to quantify the size of the centennially persistent organic carbon pool in temperate soils, Biogeosciences, 15, 2835–2849, https://doi.org/10.5194/bg-15-2835-2018, 2018.
Cécillon, L., Baudin, F., Chenu, C., Christensen, B. T., Franko, U., Houot, S., Kanari, E., Kätterer, T., Merbach, I., van Oort, F., Poeplau, C., Quezada, J. C., Savignac, F., Soucémarianadin, L. N., and Barré, P.: Partitioning soil organic carbon into its centennially stable and active fractions with machine-learning models based on Rock-Eval® thermal analysis (PARTYSOCv2.0 and PARTYSOCv2.0EU), Geosci. Model Dev., 14, 3879–3898, https://doi.org/10.5194/gmd-14-3879-2021, 2021.
Chassé, M., Lutfalla, S., Cécillon, L., Baudin, F., Abiven, S., Chenu, C., and Barré, P.: Long-term bare-fallow soil fractions reveal thermo-chemical properties controlling soil organic carbon dynamics, Biogeosciences, 18, 1703–1718, https://doi.org/10.5194/bg-18-1703-2021, 2021.
Chenu, C., Rumpel, C., and Lehmann, J.: Methods for Studying Soil Organic Matter, in: Soil Microbiology, Ecology and Biochemistry, edited by: Paul, E. A., Elsevier, 383–419, https://doi.org/10.1016/B978-0-12-415955-6.00013-X, 2015.
Chenu, C., Angers, D. A., Barré, P., Derrien, D., Arrouays, D., and Balesdent, J.: Increasing organic stocks in agricultural soils: Knowledge gaps and potential innovations, Soil Till. Res., 188, 41–52, https://doi.org/10.1016/j.still.2018.04.011, 2019.
Christensen, B. T.: Carbon and Nitrogen in Particle Size Fractions Isolated from Danish Arable Soils by Ultrasonic Dispersion and Gravity-Sedimentation, Acta Agr. Scand., 35, 175–187, https://doi.org/10.1080/00015128509435773, 1985.
Christensen, B. T.: Decomposability of organic matter in particle size fractions from field soils with straw incorporation, Soil Biol. Biochem., 19, 429–435, https://doi.org/10.1016/0038-0717(87)90034-4, 1987.
Clivot, H., Mouny, J.-C., Duparque, A., Dinh, J.-L., Denoroy, P., Houot, S., Vertès, F., Trochard, R., Bouthier, A., Sagot, S., and Mary, B.: Modeling soil organic carbon evolution in long-term arable experiments with AMG model, Environ. Modell. Softw., 118, 99–113, https://doi.org/10.1016/j.envsoft.2019.04.004, 2019.
Cotrufo, M. F., Wallenstein, M. D., Boot, C. M., Denef, K., and Paul, E.: The Microbial Efficiency-Matrix Stabilization (MEMS) framework integrates plant litter decomposition with soil organic matter stabilization: do labile plant inputs form stable soil organic matter?, Global Change Biol., 19, 988–995, https://doi.org/10.1111/gcb.12113, 2013.
Cotrufo, M. F., Soong, J. L., Horton, A. J., Campbell, E. E., Haddix, M. L., Wall, D. H., and Parton, W. J.: Formation of soil organic matter via biochemical and physical pathways of litter mass loss, Nat. Geosci., 8, 776–779, https://doi.org/10.1038/ngeo2520, 2015.
Curtin, D., Beare, M. H., and Qiu, W.: Texture effects on carbon stabilisation and storage in New Zealand soils containing predominantly 2:1 clays, Soil Res., 54, 30, https://doi.org/10.1071/SR14292, 2016.
Disnar, J. R., Guillet, B., Keravis, D., Di-Giovanni, C., and Sebag, D.: Soil organic matter (SOM) characterization by Rock-Eval pyrolysis: scope and limitations, Org. Geochem., 34, 327–343, https://doi.org/10.1016/S0146-6380(02)00239-5, 2003.
Ellert, B. H. and Bettany, J. R.: Calculation of organic matter and nutrients stored in soils under contrasting management regimes, Can. J. Soil Sci., 75, 529–538, https://doi.org/10.4141/cjss95-075, 1995.
Elliott, E. T., Burke, I. C., Monz, C. A., Frey, S. B., Paustian, K., Collins, H. P., Paul, E. A., Cole, C. V., Belvins, R. L., Lyon, D. J., Frye, W. W., Halverson, A. D., Huggins, D. R., Turco, R. F., and Hickman, M.: Terrestrial carbon pools in grasslands and agricultural soils: preliminary data from the Corn Belt and Great Plains regions, in: Defining soil quality for a sustainable environment, edited by: Doran, J. W., Coleman, D. C., Bezdicek, D. F., and Stewart, B. A., Soil Sci. Soc. of Am. Special publication number 35, https://doi.org/10.2136/sssaspecpub35.c12, 1994.
Fujisaki, K., Chapuis-Lardy, L., Albrecht, A., Razafimbelo, T., Chotte, J.-L., and Chevallier, T.: Data synthesis of carbon distribution in particle size fractions of tropical soils: Implications for soil carbon storage potential in croplands, Geoderma, 313, 41–51, https://doi.org/10.1016/j.geoderma.2017.10.010, 2018.
Haddaway, N. R., Hedlund, K., Jackson, L. E., Kätterer, T., Lugato, E., Thomsen, I. K., Jørgensen, H. B., and Isberg, P.-E.: How does tillage intensity affect soil organic carbon? A systematic review, Environ. Evid., 6, 30, https://doi.org/10.1186/s13750-017-0108-9, 2017.
Heckman, K., Throckmorton, H., Horwath, W., Swanston, C., and Rasmussen, C.: Variation in the Molecular Structure and Radiocarbon Abundance of Mineral-Associated Organic Matter across a Lithosequence of Forest Soils, Soil Syst., 2, 36, https://doi.org/10.3390/soilsystems2020036, 2018.
Hussain, I., Olson, K. R., and Ebelhar, S. A.: Long-Term Tillage Effects on Soil Chemical Properties and Organic Matter Fractions, Soil Sci. Soc. Am. J., 63, 1335–1341, https://doi.org/10.2136/sssaj1999.6351335x, 1999.
Hsieh, Y.-P.: Pool Size and Mean Age of Stable Soil Organic Carbon in Croplands, Soil Sci. Soc. Am. J., 56, https://doi.org/10.2136/sssaj1992.03615995005600020049x, 1992.
IUSS Working Group WRB: World Reference Base for Soil Resources 2014, Update 2015. International Soil Classification System for Naming Soils and Creating Legends for Soil Maps, World Soil Resources Reports, no. 106, FAO, Rome, 2015.
Jastrow, J. D., Miller, R. M., and Boutton, T. W.: Carbon Dynamics of Aggregate-Associated Organic Matter Estimated by Carbon-13 Natural Abundance, Soil Sci. Soc. Am. J., 60, 801–807, https://doi.org/10.2136/sssaj1996.03615995006000030017x, 1996.
Jolivet, C., Arrouays, D., Lévèque, J., Andreux, F., and Chenu, C.: Organic carbon dynamics in soil particle-size separates of sandy Spodosols when forest is cleared for maize cropping: Organic carbon dynamics in soil fractions, Eur. J. Soil Sci., 54, 257–268, https://doi.org/10.1046/j.1365-2389.2003.00541.x, 2003.
Kallenbach, C. M., Frey, S. D., and Grandy, A. S.: Direct evidence for microbial-derived soil organic matter formation and its ecophysiological controls, Nat. Commun., 7, 13630, https://doi.org/10.1038/ncomms13630, 2016.
Kanari, E., Barré, P., Baudin, F., Berthelot, A., Bouton, N., Gosselin, F., Soucémarianadin, L., Savignac, F., and Cécillon, L.: Predicting Rock-Eval® thermal analysis parameters of a soil layer based on samples from its sublayers; an experimental study on forest soils, Org. Geochem., 160, 104289, https://doi.org/10.1016/j.orggeochem.2021.104289, 2021.
Kanari, E., Cécillon, L., Baudin, F., Clivot, H., Ferchaud, F., Houot, S., Levavasseur, F., Mary, B., Soucémarianadin, L., Chenu, C., and Barré, P.: A robust initialization method for accurate soil organic carbon simulations, Biogeosciences, 19, 375–387, https://doi.org/10.5194/bg-19-375-2022, 2022.
Keiluweit, M., Bougoure, J. J., Nico, P. S., Pett-Ridge, J., Weber, P. K., and Kleber, M.: Mineral protection of soil carbon counteracted by root exudates, Nat. Clim. Change, 5, 588–595, https://doi.org/10.1038/nclimate2580, 2015.
Kim, K., Daly, E. J., Gorzelak, M., and Hernandez-Ramirez, G.: Soil organic matter pools response to perennial grain cropping and nitrogen fertilizer, Soil Till. Res., 220, 105376, https://doi.org/10.1016/j.still.2022.105376, 2022.
Kpemoua, T. P. I., Leclerc, S., Barré, P., Houot, S., Pouteau, V., Plessis, C., and Chenu, C.: Are carbon-storing soils more sensitive to climate change? A laboratory evaluation for agricultural temperate soils, Soil Biol. Biochem., 183, 109043, https://doi.org/10.1016/j.soilbio.2023.109043, 2023.
Kuzyakov, Y. and Bol, R.: Using natural 13C abundances to differentiate between three CO2 sources during incubation of a grassland soil amended with slurry and sugar, Z. Pflanz. Bodenkunde, 167, 669–677, https://doi.org/10.1002/jpln.200421412, 2004.
Lafargue, E., Marquis, F., and Pillot, D.: Rock-Eval 6 applications in hydrocarbon exploration, production and soil contamination studies, Oil Gas Sci. Technol., 53, 421–437, https://doi.org/10.2516/ogst:1998036, 2018.
Lal, R.: Soil Carbon Sequestration Impacts on Global Climate Change and Food Security, Science, 304, 1623–1627, https://doi.org/10.1126/science.1097396, 2004.
Lal, R.: Digging deeper: A holistic perspective of factors affecting soil organic carbon sequestration in agroecosystems, Global Change Biol., 24, 3285–3301, https://doi.org/10.1111/gcb.14054, 2018.
Lashermes, G., Nicolardot, B., Parnaudeau, V., Thuriès, L., Chaussod, R., Guillotin, M. L., Linères, M., Mary, B., Metzger, L., Morvan, T., Tricaud, A., Villette, C., and Houot, S.: Indicator of potential residual carbon in soils after exogenous organic matter application, Eur. J. Soil Sci., 60, 297–310, https://doi.org/10.1111/j.1365-2389.2008.01110.x, 2009.
Lutfalla, S., Barré, P., Bernard, S., Le Guillou, C., Alléon, J., and Chenu, C.: Multidecadal persistence of organic matter in soils: multiscale investigations down to the submicron scale, Biogeosciences, 16, 1401–1410, https://doi.org/10.5194/bg-16-1401-2019, 2019.
Pacini, L., Adatte, T., Barré, P., Boussafir, M., Bouton, N., Cécillon, L., Lamoureux-Var, V., Sebag, D., Verrecchia, E., Wattripont, A., and Baudin, F.: Reproducibility of Rock-Eval® thermal analysis for soil organic matter characterization, Org. Geochem., 186, 104687, https://doi.org/10.1016/j.orggeochem.2023.104687, 2023.
Paetsch, L., Mueller, C. W., Rumpel, C., Houot, S., and Kögel-Knabner, I.: Urban waste composts enhance OC and N stocks after long-term amendment but do not alter organic matter composition. Agr. Ecosyst. Environ., 223, 211–222, https://doi.org/10.1016/j.agee.2016.03.008, 2016.
Paustian, K., Parton, W. J., and Persson, J.: Modeling Soil Organic Matter in Organic-Amended and Nitrogen-Fertilized Long-Term Plots, Soil Sci. Soc. Am. J., 56, 476–488, https://doi.org/10.2136/sssaj1992.03615995005600020023x, 1992.
Pellerin, S., Bamière, L., Launay, C., Martin, R., Angers, D., Balesdent, J., Basile-Doelsch, I., Bellassen, V., Cardinael, R., Cécillon, L., Ceschia, E., Chenu, C., Constantin, J., Darroussin, J., Delacote, P., Delame, N., Gastal, F., Gilbert, D., and Schiavo, M.: Stocker du carbone dans les sols français, Quel potentiel au regard de l'objectif de 4 pour 1000 et à quel coût? Synthèse du rapport d'étude, INRA (France), 2019.
Peltre, C.: Potential carbon storage in soil after exogenous organic matter applications. Soil study, AgroParisTech, NNT: 2010AGPT0076, pastel-00602825, 2010.
Peltre, C., Christensen, B. T., Dragon, S., Icard, C., Kätterer, T., and Houot, S.: RothC simulation of carbon accumulation in soil after repeated application of widely different organic amendments, Soil Biol. Biochem., 52, 49–60, https://doi.org/10.1016/j.soilbio.2012.03.023, 2012.
Peltre, C., Fernández, J. M., Craine, J. M., and Plante, A. F.: Relationships between Biological and Thermal Indices of Soil Organic Matter Stability Differ with Soil Organic Carbon Level, Soil Sci. Soc. Am. J., 77, 2020–2028, https://doi.org/10.2136/sssaj2013.02.0081, 2013.
Plante, A. F., Fernández, J. M., and Leifeld, J.: Application of thermal analysis techniques in soil science, Geoderma, 153, 1–10, https://doi.org/10.1016/j.geoderma.2009.08.016, 2009.
Poeplau, C. and Don, A.: Carbon sequestration in agricultural soils via cultivation of cover crops – A meta-analysis, Agr. Ecosyst. Environ., 200, 33–41, https://doi.org/10.1016/j.agee.2014.10.024, 2015.
Poeplau, C., Don, A., Six, J., Kaiser, M., Benbi, D., Chenu, C., Cotrufo, M. F., Derrien, D., Gioacchini, P., Grand, S., Gregorich, E., Griepentrog, M., Gunina, A., Haddix, M., Kuzyakov, Y., Kühnel, A., Macdonald, L. M., Soong, J., Trigalet, S., Vermeire, M.-L., Rovira, P., van Wesemael, B., Wiesmeier, M., Yeasmin, S., Yevdokimov, I., and Nieder, R.: Isolating organic carbon fractions with varying turnover rates in temperate agricultural soils – A comprehensive method comparison, Soil Biol. Biochem., 125, 10–26, https://doi.org/10.1016/j.soilbio.2018.06.025, 2018.
Poeplau, C., Barré, P., Cécillon, L., Baudin, F., and Sigurdsson, B. D.: Changes in the Rock-Eval signature of soil organic carbon upon extreme soil warming and chemical oxidation – A comparison, Geoderma, 337, 181–190, https://doi.org/10.1016/j.geoderma.2018.09.025, 2019.
Prairie, A. M., King, A. E., and Cotrufo, M. F.: Restoring particulate and mineral-associated organic carbon through regenerative agriculture, P. Natl. Acad. Sci. USA, 120, e2217481120, https://doi.org/10.1073/pnas.2217481120, 2023.
Rumpel, C., Amiraslani, F., Chenu, C., Cardenas, M. G., Kaonga, M., Koutika, L. S., Ladha, J., Madari, B., Shirato, Y., Smith, P., Soudi, B., Soussana, J. F., Whitehead, D., and Wollenberg, E.: The 4p1000 initiative: Opportunities, limitations and challenges for implementing soil organic carbon sequestration as a sustainable development strategy, Ambio, 49, 350–360, https://doi.org/10.1007/s13280-019-01165-2, 2020.
Salvo, L., Hernández, J., and Ernst, O.: Distribution of soil organic carbon in different size fractions, under pasture and crop rotations with conventional tillage and no-till systems, Soil Till. Res., 109, 116–122, https://doi.org/10.1016/j.still.2010.05.008, 2010.
Samson, M.-E., Chantigny, M. H., Vanasse, A., Menasseri-Aubry, S., Royer, I., and Angers, D. A.: Management practices differently affect particulate and mineral-associated organic matter and their precursors in arable soils, Soil Biol. Biochem., 148, 107867, https://doi.org/10.1016/j.soilbio.2020.107867, 2020.
Sanderman, J. and Grandy, A. S.: Ramped thermal analysis for isolating biologically meaningful soil organic matter fractions with distinct residence times, SOIL, 6, 131–144, https://doi.org/10.5194/soil-6-131-2020, 2020.
Sanderman, J., Fillery, I. R. P., Jongepier, R., Massalsky, A., Roper, M. M., MacDonald, L. M., Maddern, T., Murphy, D. V., and Baldock, J. A.: Carbon sequestration under subtropical perennial pastures II: Carbon dynamics, Soil Res., 51, 771–780, https://doi.org/10.1071/SR12351, 2013.
Schädel, C., Schuur, E. A. G., Bracho, R., Elberling, B., Knoblauch, C., Lee, H., Luo, Y., Shaver, G. R., and Turetsky, M. R.: Circumpolar assessment of permafrost C quality and its vulnerability over time using long-term incubation data, Glob. Change Biol., 20, 641–652, https://doi.org/10.1111/gcb.12417, 2014.
Schädel, C., Beem-Miller, J., Aziz Rad, M., Crow, S. E., Hicks Pries, C. E., Ernakovich, J., Hoyt, A. M., Plante, A., Stoner, S., Treat, C. C., and Sierra, C. A.: Decomposability of soil organic matter over time: the Soil Incubation Database (SIDb, version 1.0) and guidance for incubation procedures, Earth Syst. Sci. Data, 12, 1511–1524, https://doi.org/10.5194/essd-12-1511-2020, 2020.
Schiedung, M., Don, A., Wordell-Dietrich, P., Alcántara, V., Kuner, P., and Guggenberger, G.: Thermal oxidation does not fractionate soil organic carbon with differing biological stabilities, J. Plant Nutr. Soil Sc., 180, 18–26, https://doi.org/10.1002/jpln.201600172, 2017.
Sokol, N. W., Sanderman, J., and Bradford, M. A.: Pathways of mineral-associated soil organic matter formation: Integrating the role of plant carbon source, chemistry, and point of entry, Global Change Biol., 25, 12–24, https://doi.org/10.1111/gcb.14482, 2019.
Sollins, P., Swanston, C., Kleber, M., Filley, T., Kramer, M., Crow, S., Caldwell, B. A., Lajtha, K., and Bowden, R.: Organic C and N stabilization in a forest soil: evidence from sequential density fractionation, Soil Biol. Biochem., 38, 3313–3324, https://doi.org/10.1016/j.soilbio.2006.04.014, 2006.
Tiemann, L. K., Grandy, A. S., Atkinson, E. E., Marin-Spiotta, E., and McDaniel, M. D.: Crop rotational diversity enhances belowground communities and functions in an agroecosystem, Ecol. Lett., 18, 761–771, https://doi.org/10.1111/ele.12453, 2015.
Torn, M. S., Kleber, M., Zavaleta, E. S., Zhu, B., Field, C. B., and Trumbore, S. E.: A dual isotope approach to isolate soil carbon pools of different turnover times, Biogeosciences, 10, 8067–8081, https://doi.org/10.5194/bg-10-8067-2013, 2013.
Védère, C., Vieublé Gonod, L., Pouteau, V., Girardin, C., and Chenu, C.: Spatial and temporal evolution of detritusphere hotspots at different soil moistures. Soil Biol. Biochem. 150, 107975, https://doi.org/10.1016/j.soilbio.2020.107975, 2020.
Virto, I., Barré, P., Burlot, A., and Chenu, C.: Carbon input differences as the main factor explaining the variability in soil organic C storage in no-tilled compared to inversion tilled agrosystems, Biogeochemistry, 108, 17–26, https://doi.org/10.1007/s10533-011-9600-4, 2012.
Von Lützow, M., Kögel-Knabner, I., Ekschmitt, K., Matzner, E., Guggenberger, G., Marschner, B., and Flessa, H.: Stabilization of organic matter in temperate soils: mechanisms and their relevance under different soil conditions – a review: Mechanisms for organic matter stabilization in soils, Eur. J. Soil Sci., 57, 426–445, https://doi.org/10.1111/j.1365-2389.2006.00809.x, 2006.
Von Lützow, M., Kögel-Knabner, I., Ekschmitt, K., Flessa, H., Guggenberger, G., Matzner, E., and Marschner, B.: SOM fractionation methods: Relevance to functional pools and to stabilization mechanisms, Soil Biol. Biochem., 39, 2183–2207, https://doi.org/10.1016/j.soilbio.2007.03.007, 2007.
Wander, M. M., Bidart, M. G., and Aref, S.: Tillage Impacts on Depth Distribution of Total and Particulate Organic Matter in Three Illinois Soils, Soil Sci. Soc. Am. J., 62, 1704–1711, https://doi.org/10.2136/sssaj1998.03615995006200060031x, 1998.
Yeasmin, S., Singh, B., Johnston, C. T., Sparks, D. L., and Hua, Q.: Changes in Particulate and Mineral Associated Organic Carbon with Land Use in Contrasting Soils, Biogeosciences Discuss. [preprint], https://doi.org/10.5194/bg-2019-416, 2019.
Zhang, F., Chen, X., Yao, S., Ye, Y., and Zhang, B.: Responses of soil mineral-associated and particulate organic carbon to carbon input: A meta-analysis, Sci. Total Environ., 829, 154626, https://doi.org/10.1016/j.scitotenv.2022.154626, 2022.