the Creative Commons Attribution 4.0 License.
the Creative Commons Attribution 4.0 License.
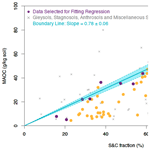
The six rights of how and when to test for soil C saturation
Sebastian Doetterl
Moritz Laub
Claude R. Müller
Marijn Van de Broek
The concept of soil organic carbon (SOC) saturation emerged a bit more than 2 decades ago as our mechanistic understanding of SOC stabilization increased. Recently, the further testing of the concept across a wide range of soil types and environments has led some people to challenge the fundamentals of soil C saturation. Here, we argue that, to test this concept, one should pay attention to six fundamental principles or “rights” (R's): the right measures, the right units, the right dispersive energy and application, the right soil type, the right clay type, and the right saturation level. Once we take care of those six rights across studies, we find a maximum of C stabilized by minerals and estimate based on current data available that this maximum stabilization is around 82 ± 4 g C kg−1 silt + clay for 2 : 1-clay-dominated soils while most likely being only around 46 ± 4 g C kg−1 silt + clay for 1 : 1-clay-dominated soils. These estimates can be further improved using more data, especially for different clay types across varying environmental conditions. However, the bigger challenge is a matter of which C sequestration strategies to implement and how to implement them in order to effectively reach this g C kg−1 silt + clay in soils across the globe.
- Article
(776 KB) - Full-text XML
- BibTeX
- EndNote
In recent years, several studies (e.g., Begill et al., 2023; Salonen et al., 2023) have questioned the concept of soil carbon (C) saturation, i.e., organic C stabilized by soil minerals (Hassink, 1997; Six et al., 2002). Here, we want to draw attention to six fundamentals that we think one should be cognizant about when testing and questioning soil C saturation.
Soil C saturation is, by definition, a non-responsiveness of soil C content upon an increase in C inputs and thus should ideally be assessed by plotting stabilized soil C (e.g., mineral-associated organic C (MAOC)) versus C input (Six et al., 2002). However, most datasets used to test saturation contain a large range of soil textures, clay types, and climates, where C input from vegetation to soil (especially to specific soil C fractions) is usually not known; thus, the resulting plots of soil C versus C input are often not sensible and thus inconclusive (see West and Six, 2007; Stewart et al., 2007; Feng et al., 2014). Hence, the most practical way to test saturation is plotting MAOC vs. silt + clay content as a proxy for the reactive mineral phase in soil. Specifically for fractions of soil C, in Stewart et al. (2007), it was correctly argued that saturation can also be tested by plotting a fraction of soil C, such as MAOC, versus total SOC. However, this does not account for the above-mentioned confounding factors when going across systems or biomes. Therefore, the most elegant, accurate, and thus preferable way to test for saturation is by plotting MAOC versus silt + clay content in the right units.
As for any analysis, using the correct units is of great importance. For C saturation, the best data analysis that can be done is relating MAOC (y axis), expressed per unit total soil (i.e., g C kg−1 total soil or mg C g−1 total soil; the emphasis is on per total soil, which is < 2 mm fine soil) to silt + clay content (in units of g silt + clay kg−1 soil or %) (x axis). Then, to correctly quantify the maximum MAOC in the right unit, one should conduct a boundary analysis (or a quantile regression; Koenker, 2005) where the slope of the linear boundary curve is the maximum MAOC (expressed in g MAOC per unit of silt + clay – i.e., the portion of the soil < 50 or 63 µm; we remark here that it is not total soil but silt + clay that is the right unit for maximum MAOC) (see Feng et al., 2013). Depending on the exact units used on the y axis and x axis, a conversion might have to be done to put the maximum MAOC in the preferred right unit (i.e., g C kg−1 silt + clay or mg C g−1 silt + clay or % C of the silt + clay). It is also pertinent to have the curve (1) not predict beyond the range of available silt + clay measurements; (2) be linear because, from a mechanistic perspective, it does not make sense that the capacity to stabilize C by individual silt and clay particles is dependent on the total amount of silt + clay in the soil; and (3) go through the origin because if there are zero minerals (i.e., silt + clay) then there is, by definition, also zero MAOC – hence, a non-zero intercept is theoretically not possible. Nevertheless, the difference between boundary lines without and with an y intercept could indicate the degree of contamination of MAOC with POC and thus which samples should possibly be omitted from the analysis because of too high levels of contamination. Here, we want to give an example of how the units matter: Georgiou et al. (2022) state that their data-driven maximum MAOC estimate of 86 g C kg−1 mineral (= silt + clay) is a notable update to the model-predicted 45–50 g C kg−1 soil reported by Cotrufo et al. (2019). However, the value of 86 has the unit of g C kg−1 silt + clay, whereas the estimate of 45–50 has the unit of g C kg−1 (total) soil; thus, these are not comparable.
As for any soil C fractionation method, the separation of MAOC is operationally defined; thus, methodological shortcomings should always be considered when interpreting the data. For the testing of MAOC saturation, it is obviously pertinent to separate an as-pure-as-possible mineral fraction that is not contaminated by, for example, particulate organic carbon (POC). The separation of the mineral fraction is done by size and/or density separation after dispersing the soil into primary particles. Crucial here is that enough dispersive energy (through shaking, ultrasonic dispersion, or any other dispersive method) is applied to break up all soil aggregates to release all sand-sized particles without applying too much dispersive energy that would break up POC and contaminate the mineral fraction. In that sense, Amelung and Zech (1999) did a very thorough assessment of the influence of ultrasonic dispersion on POM redistribution to the silt + clay fraction. They concluded that 3 kJ for 10 g soil is optimal and that ≥5 kJ disrupts POC and contaminates MAOC with POC. As an example, Begill et al. (2023) report that they used 100 J mL−1 for 10 g soil in 150 mL water. Hence, they disrupted 10 g soil with 15 kJ, 3 times the maximum level of energy recommended by Amelung and Zech (1999), thus most likely disrupting POC and contaminating MAOC. One could do a density flotation to remove contaminating POC to get a more pure MAOC, but it is our experience that one cannot float all of the POC without also starting to float some MAOC because there is a continuum of densities from POC to MAOC. Thus, even though the ideal dispersive energy is soil dependent, we recommend limiting the dispersive energy to the level recommended by Amelung and Zech (1999) and, most importantly, to always perform a visual inspection with a microscope of the POC fraction to ensure the break-up of all soil aggregates (not concretions; this is specifically important in tropical soils that have very stable concretions (pseudo-sand) that cannot be broken up without POC contamination of MAOC) and of the MAOC to ensure a limited contamination (because no contamination is impossible) of the MAOC with POC (especially in high-POC soils). The principles of Amelung and Zech (1999) still hold up to this day despite the fact that more recent works (e.g., Poeplau and Don, 2014; Graf-Rosenfellner et al., 2018) did emphasize that, even if one stays below the recommended maximum energy input, results between the applications of the same total amount of energy input but at varying intensities and lengths can still significantly affect the overall result of a soil carbon fractionation analysis.
When testing the limits of mineral C stabilization, confounding influences of other stabilization factors should be avoided. For example, soil types characterized by anoxic conditions such as Histosols, Gleysols, Stagnosols, Umbrisols, and Cryosols should not be included in an analysis of saturation of MAOC, even if they are currently drained or melted. In such soils, MAOC has, at least in part, accumulated due to oxygen limitation and not through mineral stabilization. We are not stating that mineral stabilization does not occur or is mechanistically different in such soils, but the anoxic conditions confound the effect of stabilization by interaction with the minerals. Furthermore, these soil types often have high POC levels and thus an increased chance of contamination of MAOC with POC during dispersion. Also, Anthrosols and Technosols are not suitable to assess a “natural” limit because of all the anthropogenic processes that are or were at play. Andosols should probably be treated in their own category due to their specific soil properties (i.e., high levels of allophane and pyrophosphate-extractable aluminum) (see Beare et al., 2014, for a discussion on the soil C stabilization mechanisms in Andosols). For the same reason, Alisols could also be considered in their own category (or together with Andosols) for a saturation limit analysis. For Calcisols and Gypsisols, one obviously has to remove all inorganic carbon before determining MAOC, but this can lead to a high uncertainty in the estimate of MAOC; hence, caution should be applied. Of particular importance is that samples with geogenic C are not included (see Kalks et al., 2021, for the prevalence of geogenic carbon in soils). Lastly, buried soil layers, as found in Colluvisols, should probably not be included due to their current or past conditions that can lead to artificially high levels of MAOC. One could start thinking that the saturation concept is then limited to few soils, but the other 21 (out of 32) WRB soil groups do dominate across the globe, and the soil types indicated to not be included in the analysis are limited in range and are often associated with specific positions within the landscape (except Cryosols, but those are often not managed and are thus not suitable targets to increase soil C content); even Gleysols cover only ∼ 5 % of the globe.
As has been done in most studies estimating a maximum of MAOC (Hassink, 1997; Six et al., 2002; Feng et al., 2013; Matus, 2021; Georgiou et al., 2022), the separation of soils with different phyllosilicate clay types is necessary because of their differences in reactivity and thus in their potential to stabilize MAOC (as we indicated specifically for Andosols, which are dominated by very reactive clays, in the previous section). In that sense, it would be very interesting to have estimates of the saturation level per clay type because then we could define a saturation level for each soil based on the amount of specific clays present in the soil. However, the currently available data do not allow for that; thus, a separation between the broad categories of 1 : 1 versus 2 : 1 clay types is all that can be done. Similarly, what is still missing at larger scales is a better understanding of the role of other, non-silicate clay-sized minerals for MAOC stabilization, such as varying metal phases which do play a dominant role in the mineralogy of many highly weathered soils. However, there are recent efforts to map clay type distributions across the globe (see Ito and Wagai, 2017), and, hopefully soon, the resolution of these maps will be fine enough for C saturation analyses.
Since many soils are not receiving high-enough C inputs to come close to soil C saturation, particularly subsoils, it is important to be cognizant of what level of MAOC can be reached for a certain silt + clay content. A search through the literature led to three publications that estimated the maximum MAOC for 2 : 1-mineral-dominated soils with the “right measures” and “right units”, as outlined above: Feng et al. (2013) estimated 84 ± 4 g C kg−1 silt + clay, Georgiou et al. (2022) estimated 86 ± 9 g C kg−1 silt + clay, and Matus (2021) estimated 81 g C kg−1 silt + clay (note: Matus (2021) estimated it for all mineralogies and did have a small intercept, but samples at the boundary line were 2 : 1 dominated). Although there is overlap in data between the different studies, the similarity is striking, and they average to a value of 84 ± 3 g C kg−1 silt + clay. Very important to note here is that Beare et al. (2014) also estimated a maximum MAOC for allophanic (153 g C kg−1 silt + clay) versus non-allophanic soils (116 g C kg−1 silt + clay), but the non-allophanic soils include Gleysols and some vitric Andosols. Thus, it is not surprising that they have a higher estimate. Nevertheless, their very high estimate for the allophanic soils confirms that they should be treated in their own category. For 1 : 1-mineral-dominated soils, we found two very similar estimates (in part because of an overlap in data points between the two studies): 43 ± 4 g C kg−1 silt + clay (Feng et al., 2013) and 48 ± 6 g C kg−1 silt + clay (Georgiou et al., 2022), averaging to 46 ± 4 g C kg−1 silt + clay.
Here, we also want to give two examples of how considering the right saturation level is important. First, Salonen et al. (2023) indicated that their soils sequestered more MAOC than is suggested by saturation estimates and that the relationship between total soil OC and MAOC remained linear without a flex point. A look at their data shows that they are measuring a maximum MAOC of 60 g C kg−1 total soil for a 2 : 1-mineral-dominated soil with 80 % silt + clay (of which 68 % is actually clay). If we consider the average estimate for maximum MAOC in 2 : 1-mineral-dominated soils of 84 g C kg−1 silt + clay and the 80 % silt + clay in their soil, then we estimate saturation to be at 67 (= 84 ⋅ 80 %) g C kg−1 total soil of MAOC. Hence, it is no surprise that Salonen et al. (2023) did not see saturation of the MAOC because their soils were below the limit. Second, Schweizer et al. (2021) argued, based on the observation of a higher MAOC (expressed in the right unit of mg C g−1 fraction) in soils with low clay content and an observed patchy and piled-up structure of SOM binding to clays, that clay content is not necessarily a limiting factor for MAOC storage. However, all their reported MAOC contents are below the 84 mg C g−1 fraction (see Fig. 1e in their publication) and thus perfectly align with our estimate of maximum MAOC.
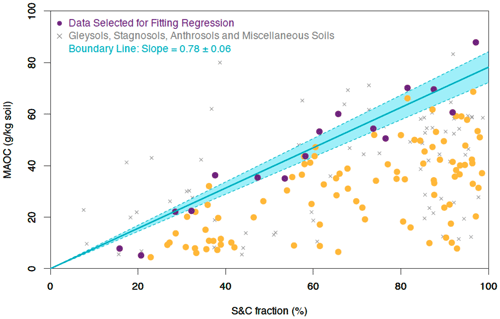
Figure 1Boundary analysis of mineral-associated organic C (MAOC) versus silt and clay content of the fine soil based on the Begill et al. (2023) dataset without Gleysols, Stagnosols, Anthrosols, and “miscellaneous” soils (indicated by gray crosses). The boundary line (in blue) is based on the 90th percentile (purple dots) with a 95 % confidence interval (blue shade). Orange dots are all other soils in the dataset, including Cambisols, Luvisols, Phaeozems, Regosols, and Vertisols. Note that a much weaker trend between MAOC and the silt and clay content is visible for the subgroup of soils with gray crosses, indicating the presence of non-texture-dependent factors (e.g., anoxic conditions) determining the measured MAOC content in those soils.
Lastly, to show that getting the rights right is important, we used the dataset (available at https://doi.org/10.5281/zenodo.7966076) of Begill et al. (2023), who suggested that there is no maximum MAOC content. Given that their dataset is based solely on German soils, the right clay mineralogy is 2 : 1, with a maximum MAOC of 84 ± 3 g C kg−1 silt+clay. Given this, 159 out of their 189 soils are below the MAOC saturation limit; only 30 (<16 % of all samples) are above the saturation limit. Of those 30 soils, 13 are Gleysols, 4 are Stagnosols, 3 are Anthrosols, 1 is a miscellaneous soil, and 1 soil is a Regosol with an extremely high silt + clay content (97 %) while not being a well-developed soil; hence, it cannot be excluded that this soil contains geogenic carbon stemming from the (likely) sedimentary rock parent material. Thus, eight soils remain above the limit after considering the right soil type, which is 4 % of the soils in the dataset. Furthermore, seven out of those soils are below 91 g C kg−1 silt + clay, which is within 1 standard deviation of the value of Georgiou et al. (2022), i.e., exactly what you would expect in a normal distribution of samples and/or errors. However, more interesting is that when all Gleysols, Stagnosols, Anthrosols, and miscellaneous soils are taken out of the dataset and a boundary analysis is done, we find a perfect boundary with an estimated maximum MAOC of 78 ± 6 g C kg−1 silt + clay (see Fig. 1), which is a very similar estimate to others for 2 : 1-dominated soils, thus supporting the use of the rights to estimate rightly the maximum mineral stabilization. Including this additional estimate in the three examples above leads to an average for 2 : 1-mineral-dominated soils of 82 ± 4 g C kg−1 silt + clay or, thus, roughly 8 % C in the mineral fraction.
In conclusion, we can confidently state that (1) there is strong empiric evidence for a maximum of C stabilization by soil minerals, and (2) without more mineralogy data, the estimate of 82 g C kg−1 silt + clay for 2 : 1-clay-dominated soils is rather solid, while the value of 46 g C kg−1 silt + clay for 1 : 1-clay-dominated soils has a larger uncertainty. Furthermore, from a practical standpoint, we conclude the following:
-
Most “managed” soils have a substantial C saturation deficit.
-
We should focus our efforts to sequester C (both as MAOC and POC) in soils with a high silt + clay content that are far below the maximum MAOC.
-
In sandy soils, we should focus on how to get POC stored because the MAOC will be saturated fairly quickly (unless they have anoxic conditions).
-
When estimating potential rates and amounts of sequestering soil C with models, saturation dynamics should be considered (see Stewart et al., 2008; Georgiou et al., 2022) and made spatially explicit based on environmental conditions.
We only used data from Begill et al. (2023) that are available at https://doi.org/10.5281/zenodo.7966076 (Poeplau, 2023).
Conceptualization was done by JS. Formal analysis was done by CRM and JS. All the authors conducted the investigation. JS wrote the initial draft. All the authors reviewed and edited several drafts, including the final paper.
At least one of the (co-)authors is a member of the editorial board of SOIL. The peer-review process was guided by an independent editor, and the authors also have no other competing interests to declare.
Publisher’s note: Copernicus Publications remains neutral with regard to jurisdictional claims made in the text, published maps, institutional affiliations, or any other geographical representation in this paper. While Copernicus Publications makes every effort to include appropriate place names, the final responsibility lies with the authors.
Funding to Claude R. Müller and Marijn Van de Broek was provided by a Swiss National Science Foundation grant (SNSF; 2020; PZ00P2_193617/1). Funding to Moritz Laub was provided by a Swiss National Science Foundation grant (SNSF; 2017; 200021_172940).
This paper was edited by Bas van Wesemael and Jeanette Whitaker and reviewed by two anonymous referees.
Amelung, W. and Zech, W.: Minimisation of organic matter disruption during particle-size fractionation of grassland epipedons, Geoderma, 92, 73–85, https://doi.org/10.1016/S0016-7061(99)00023-3, 1999.
Beare, M. H., McNeill, S. J., Curtin, D., Parfitt, R. L., Jones, H. S., Dodd, M. B., and Sharp, J.: Estimating the organic carbon stabilisation capacity and saturation deficit of soils: a New Zealand case study, Biogeochemistry, 120, 71–87, https://doi.org/10.1007/s10533-014-9982-1, 2014.
Begill, N., Don, A., and Poeplau, C.: No detectable upper limit of mineral-associated organic carbon in temperate agricultural soils, Glob. Change Biol., 29, 4662–4669, https://doi.org/10.1111/gcb.16804, 2023.
Cotrufo, M. F., Ranalli, M. G., Haddix, M. L., Six, J., and Lugato, E.: Soil carbon storage informed by particulate and mineral-associated organic matter, Nat. Geosci., 12, 989–994, https://doi.org/10.1038/s41561-019-0484-6, 2019.
Feng, W., Plante, A. F., and Six, J.: Improving estimates of maximal organic carbon stabilization by fine soil particles, Biogeochemistry, 112, 81–93, https://doi.org/10.1007/s10533-011-9679-7, 2013.
Feng, W., Xu, M., Fan, M., Malhi, S. S., Schoenau, J. J., Six, J., and Plante, A. F.: Testing for soil carbon saturation behavior in agricultural soils receiving long-term manure amendments, Can. J. Soil Sci., 94, 281–294, https://doi.org/10.4141/cjss2013-012, 2014.
Georgiou, K., Jackson, R. B., Vindušková, O., Abramoff, R. Z., Ahlström, A., Feng, W., Harden, J. W., Pellegrini, A. F. A., Polley, H. W., Soong, J. L., Riley, W. J., and Torn, M. S.: Global stocks and capacity of mineral-associated soil organic carbon, Nat. Commun., 13, 3797, https://doi.org/10.1038/s41467-022-31540-9, 2022.
Graf-Rosenfellner, M., Kayser G., Guggenberger, G., Kaiser, K., Büks, F., Kaiser, M., Mueller, C. W., Schrumpf, M., Welp, G., and Lang, F.: Replicability of aggregate disruption by sonication – an inter-laboratory test using three different soils from Germany, J. Plant Nutr. Soil Sc., 181, 894–904, https://doi.org/10.1002/jpln.201800152, 2018.
Hassink, J.: The capacity of soils to preserve organic C and N by their association with clay and silt particles, Plant Soil, 191, 77–87, https://doi.org/10.1023/A:1004213929699, 1997.
Ito, A. and Wagai, R.: Global distribution of clay-size minerals on land surface for biogeochemical and climatological studies, Sci. Data, 4, 170103, https://doi.org/10.1038/sdata.2017.103, 2017.
Kalks, F., Noren, G., Mueller, C. W., Helfrich, M., Rethemeyer, J., and Don, A.: Geogenic organic carbon in terrestrial sediments and its contribution to total soil carbon, SOIL, 7, 347–362, https://doi.org/10.5194/soil-7-347-2021, 2021.
Koenker, R.: Quantile Regression, Cambridge University Press, Cambridge, https://doi.org/10.1017/CBO9780511754098, 2005.
Matus, F. J.: Fine silt and clay content is the main factor defining maximal C and N accumulations in soils: a meta-analysis, Sci. Rep., 11, 6438, https://doi.org/10.1038/s41598-021-84821-6, 2021.
Poeplau, C.: Dataset of manuscript “No detectable upper limit of mineral associated carbon in temperate agricultural soils”, Zenodo [data set], https://doi.org/10.5281/zenodo.7966076, 2023.
Poeplau, C. and Don, A.: Effect of ultrasonic power on soil organic carbon fractions, J. Plant Nutr. Soil Sc., 177, 137–140, https://doi.org/10.1002/jpln.201300492, 2014.
Salonen, A.-R., Soinne, H., Creamer, R., Lemola, R., Ruoho, N., Uhlgren, O., de Goede, R., and Heinonsalo, J.: Assessing the effect of arable management practices on carbon storage and fractions after 24 years in boreal conditions of Finland, Geoderma Regional, 34, e00678, https://doi.org/10.1016/j.geodrs.2023.e00678, 2023.
Schweizer, S. A., Mueller, C. W., Höschen, C., Ivanov, P., and Kögel-Knabner, I.: The role of clay content and mineral surface area for soil organic carbon storage in an arable toposequence, Biogeochemistry, 156, 401–420, https://doi.org/10.1007/s10533-021-00850-3, 2021.
Six, J., Conant, R. T., Paul, E. A., and Paustian, K.: Stabilization mechanisms of soil organic matter: Implications for C-saturation of soils, Plant Soil, 241, 155–176, 2002.
Stewart, C. E., Paustian, K., Conant, R. T., Plante, A. F., and Six, J.: Soil carbon saturation: concept, evidence and evaluation, Biogeochemistry, 86, 19–31, https://doi.org/10.1007/s10533-007-9140-0, 2007.
Stewart, C. E., Paustian, K., Conant, R. T., Plante, A. F., and Six, J.: Soil carbon saturation: Evaluation and corroboration by long-term incubations, Soil Biol. Biochem., 40, 1741–1750, https://doi.org/10.1016/j.soilbio.2008.02.014, 2008.
West, T. O. and Six, J.: Considering the influence of sequestration duration and carbon saturation on estimates of soil carbon capacity, Climatic Change, 80, 25–41, https://doi.org/10.1007/s10584-006-9173-8, 2007.