the Creative Commons Attribution 4.0 License.
the Creative Commons Attribution 4.0 License.
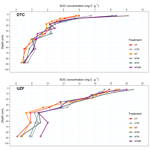
Mulch application as the overarching factor explaining increase in soil organic carbon stocks under conservation agriculture in two 8-year-old experiments in Zimbabwe
Armwell Shumba
Regis Chikowo
Christian Thierfelder
Marc Corbeels
Johan Six
Rémi Cardinael
Conservation agriculture (CA), combining reduced or no tillage, permanent soil cover, and improved rotations, is often promoted as a climate-smart practice. However, our understanding of the impact of CA and its respective three principles on top- and subsoil organic carbon stocks in the low-input cropping systems of sub-Saharan Africa is rather limited. This study was conducted at two long-term experimental sites established in Zimbabwe in 2013. The soil types were abruptic Lixisols at Domboshava Training Centre (DTC) and xanthic Ferralsol at the University of Zimbabwe farm (UZF). The following six treatments, which were replicated four times, were investigated: conventional tillage (CT), conventional tillage with rotation (CTR), no tillage (NT), no tillage with mulch (NTM), no tillage with rotation (NTR), and no tillage with mulch and rotation (NTMR). Maize (Zea mays L.) was the main crop, and treatments with rotation included cowpea (Vigna unguiculata L. Walp.). The soil organic carbon (SOC) concentration and soil bulk density were determined for samples taken from depths of 0–5, 5–10, 10–15, 15–20, 20–30, 30–40, 40–50, 50–75 and 75–100 cm. Cumulative organic inputs to the soil were also estimated for all treatments. SOC stocks at equivalent soil mass were significantly (p<0.05) higher in the NTM, NTR and NTMR treatments compared with the NT and CT treatments in the top 5 cm and top 10 cm layers at UZF, while SOC stocks were only significantly higher in the NTM and NTMR treatments compared with the NT and CT treatments in the top 5 cm at DTC. NT alone had a slightly negative impact on the top SOC stocks. Cumulative SOC stocks were not significantly different between treatments when considering the whole 100 cm soil profile. Our results show the overarching role of crop residue mulching in CA cropping systems with respect to enhancing SOC stocks but also that this effect is limited to the topsoil. The highest cumulative organic carbon inputs to the soil were observed in NTM treatments at the two sites, and this could probably explain the positive effect on SOC stocks. Moreover, our results show that the combination of at least two CA principles including mulch is required to increase SOC stocks in these low-nitrogen-input cropping systems.
- Article
(3597 KB) - Full-text XML
-
Supplement
(1031 KB) - BibTeX
- EndNote
Soil organic carbon (SOC) is an important determinant of soil fertility, productivity and sustainability, and it is also a useful indicator of soil quality in tropical agricultural systems, where nutrient-poor and highly weathered soils are managed with few external inputs (Lal, 1997; Feller and Beare, 1997; Chivenge et al., 2007). Therefore, rebuilding depleted SOC stocks in such soils holds the potential to contribute to climate change mitigation (Bossio et al., 2020; Minasny et al., 2017; Swanepoel et al., 2016) via the sustainable management of agricultural soils (Paustian et al., 2016; Dignac et al., 2017).
Conservation agriculture (CA), based on minimum soil disturbance, crop residue retention and crop rotation, has been known to improve surface SOC, with beneficial effects on soil functioning such as improved water infiltration (Thierfelder and Wall, 2012, 2009) and better aggregate stability (Six et al., 1999; Thierfelder and Wall, 2012). However, the potential of CA to increase SOC stocks and, thus, mitigate climate change has been much debated (Corbeels et al., 2020a), although the general understanding is that this potential is relatively low (Du et al., 2017; Powlson et al., 2014, 2016; Cheesman et al., 2016; Corbeels et al., 2020a). In fact, soil carbon (C) storage has often been overestimated for CA due to shallow soil sampling. Compared with conventional tillage systems, no-tillage management redistributes SOC in the soil profile, with higher concentrations in the topsoil but potentially lower concentrations below, which can result in no differences in the whole-profile SOC stocks between no-tillage and conventional tillage systems (Angers and Eriksen-Hamel, 2008). However, this lack of a significant difference in many studies assessing whole-profile SOC stocks stems from insufficient statistical power to accurately assess the potentially significant SOC changes (Kravchenko and Robertson, 2011).
CA can potentially build SOC in deeper soil layers via, for example, the use of cover crops with deeper root systems (Kell, 2011; Thorup-Kristensen et al., 2020; Yang et al., 2023). However, proper soil sampling strategies that account for both topsoil and subsoil (>30 cm depth) SOC stocks must be prioritized. Over the past 2 decades, soil sampling has often been limited to the topsoil plough layer (0–30 cm) (Dube et al., 2012; Powlson et al., 2016; Patra et al., 2019; Yost and Hartemink, 2020), in which SOC concentrations, root density (Chikowo et al., 2003) and microbial activity (Mtambanengwe et al., 2004) are generally largest (Rumpel et al., 2012); this layer is also the minimum default soil sampling depth recommended by the Intergovernmental Panel for Climate Change (IPCC, 2019). In a meta-analysis of SOC stocks in the top 1 m of soils, Balesdent et al. (2018) found that soils below 0.3 m contain on average 47 % of the total SOC stock and account for 19 % of the SOC that has been recently incorporated. Therefore, focusing on topsoil only could underestimate the SOC storage potential of agricultural management practices (Cardinael et al., 2015). In turn, this could result in incorrect conclusions on the climate change mitigation potential of agricultural management practices.
There have been many studies on the effects of CA on crop productivity and its benefits with respect to soil health (Corbeels et al., 2020b; Kimaro et al., 2016; Mhlanga et al., 2022b; Swanepoel et al., 2018; Thierfelder et al., 2015, 2017; Thierfelder and Mhlanga, 2022). Other studies, however, have fuelled the debate on CA practicality and adoption in sub-Saharan Africa (SSA) (Giller et al., 2009, 2015; Kassam et al., 2019). Despite these studies, the effects of CA on SOC dynamics have not been widely investigated in SSA. Thierfelder et al. (2017) alluded to the fact that data on the climate change mitigation potential of CA in southern Africa are scanty and advocated for more research to better quantify the mitigation effects of CA as a climate-smart technology. It has also been observed that, depending on socio-economic and biophysical conditions, farmers may find it easier to adopt certain CA principles and/or their different combinations (Mbanyele et al., 2021; Baudron et al., 2012), although this assertion has also fuelled new debates (Thierfelder et al., 2018). Therefore, the focus of this work was the individual vs. combined effects of CA principles (no tillage, crop residue retention and crop rotation) on SOC stocks.
As changes in SOC stocks take time to be detected, long-term experiments are crucial; however, these experiments are rare, especially in Africa (Bationo et al., 2013; Cardinael et al., 2022; Powlson et al., 2016; Thierfelder and Mhlanga, 2022). This study was conducted on two long-term experimental sites established in Zimbabwe in 2013. We hypothesized that the full combination of CA components would be associated with higher increases in SOC stocks than the adoption of only one component. This increase in SOC stocks could mainly be due to increased C inputs to the soil, especially under minimum soil disturbance. However, C inputs due to crop rotation could be indirect via increased crop productivity stemming from a reduction in biotic pressure (pests and diseases); therefore, C inputs to the soil might also be increased via the latter process. Cereals, in cereal–legume rotations may benefit from added soil nitrogen via biological nitrogen fixation from the preceding legume crop, thereby enhancing their productivity. Crop diversification, on the other hand, can enhance soil biological processes by increasing the diversity and/or abundance of microfauna like mycorrhizae. This, in turn, improves aggregate stability and offers physical protection for SOC. Lastly, high-quality residues (from the legume crop) have been shown to be preferentially stabilized in the soil due to a higher C use efficiency of soil microbes (Cotrufo et al., 2013; Kopittke et al., 2018).
2.1 Study sites
This study was conducted at two long-term experimental sites established in November 2013 by the International Maize and Wheat Improvement Center (CIMMYT). The site at the University of Zimbabwe Farm (UZF) is located about 12 km north of Harare ( S, E), whereas the site at the Domboshava Training Centre (DTC) is located about 30 km north-east of Harare ( S, E). UZF soils are dolerite-derived xanthic Ferralsols (FAO classification) and are medium-textured sandy clay loams (34 % clay) in the top 20 cm with a subsoil (20–40 cm) of slightly higher clay content (38 %). DTC soils are granite-derived abruptic Lixisols (FAO classification) and are light-textured sandy loams (15 % clay) in the 0–20 cm layer; these topsoils overlay an abruptly heavier-textured subsoil (20–40 cm) of 30 % clay (Fig. 1).
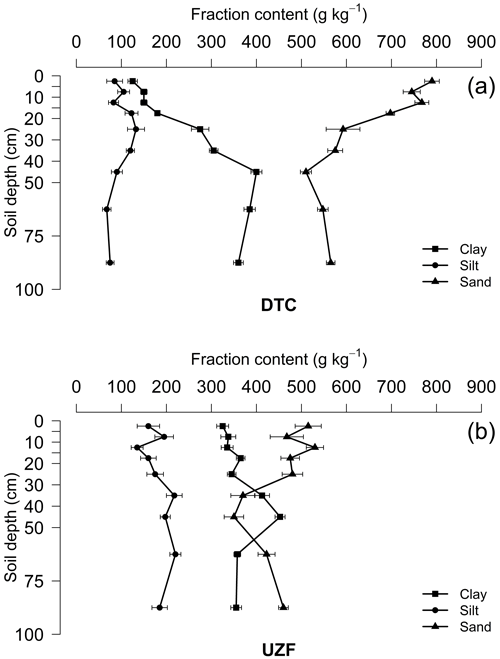
Figure 1Soil texture to 1 m soil depth at the Domboshava Training Centre (DTC) (a) and University of Zimbabwe Farm (UZF) (b) sites in Zimbabwe. Error bars represent standard errors (N=4).
The two study sites have a sub-tropical climate with cool, dry winters and hot, wet summers with mean annual minimum and maximum temperatures of 12 and 25 ∘C, respectively (Mapanda et al., 2010). The rainy season starts in November and tails off in March with a mean annual rainfall of 826 and 814 mm at UZF and DTC, respectively (Mhlanga et al., 2022a). Cumulative seasonal rainfall in 2019–2020 (474 mm) was almost half of the rainfall received in the 2020–2021 season (932 mm) at DTC (Shumba et al., 2023a). At UZF, the cumulative seasonal rainfall was 551 and 637 mm in the 2019–2020 and 2020–2021 cropping seasons, respectively. On average, the respective cropping season minimum and maximum temperatures were 16.9 and 28.1 ∘C in 2019–2020 and 15.5 and 27.2 ∘C in 2020–2021 at DTC and 15.5 and 28.6 ∘C in 2019–2020 and 15.3 and 27.5 ∘C in the 2020–2021 at UZF.
2.2 Experimental treatments and crop management
Two identical experiments were set up at the study sites, and treatments have been maintained every season since November 2013. The experiments were set up in a randomized complete block design (RCBD) with eight treatments replicated in four blocks. However, we only investigated six of these treatments in this study. All crop residues were removed soon after harvesting in all treatments, stored and then applied prior to planting in treatments with mulch. The six treatments in our study were as follows:
- i.
Conventional tillage (CT). At DTC, land preparation was done by digging with a hand hoe, and maize (Zea mays L.) was sown as a sole crop in rip lines that were created afterwards using an animal-drawn Magoye ripper (a traditional plough with the mouldboard replaced with a ripper tine). At UZF, maize was sown as a sole crop in planting basins (approximately 10 cm diameter and 10 cm depth) created using a hand hoe.
- ii.
Conventional tillage with rotation (CTR). Land preparation was done as in the CT treatment, and maize was rotated with cowpea (Vigna unguiculata L.).
- iii.
No tillage (NT). At DTC, maize was sown as a sole crop in rip lines created using an animal-drawn Magoye ripper (no further soil disturbance occurred). At UZF, maize was sown as a sole crop in planting basins (approximately 15 cm diameter and 15 cm depth) created using a hand hoe.
- iv.
No tillage with mulch (NTM). Maize was sown as in the NT treatment, and maize residues from the previous season were applied on the soil surface between maize rows at planting at a rate of 2.5 t DM ha−1.
- v.
No tillage with rotation (NTR). Maize was sown in rip lines and rotated with cowpea.
- vi.
No tillage with mulch and rotation (NTMR). Maize was sown in rip lines and rotated with cowpea, and maize residues were applied on the soil surface between maize rows at planting at a rate of 2.5 t DM ha−1.
Crop residues were removed every year after harvest and weighed again to maintain the exact 2.5 t DM ha−1 residue weight year after year. There were 24 respective plots at each site that were 6 m wide and 12 m long (72 m2). Treatments with rotation (CTR, NTR and NTMR) were split into 6 m wide and 6 m long (36 m2) subplots where maize and cowpea were grown interchangeably every season (maize was sown on one side of the plot and cowpea was sown on the other).
The inter-row spacing was 90 and 45 cm for maize and cowpea, respectively, and the in-row spacing was 25 cm for both crops; this translated to 44 444 and 88 888 plants ha−1, respectively. Three seeds were planted per planting station and thinned to one after emergence. Basal dressing of nitrogen (N), phosphorus (P) and potassium (K) was applied within 5 cm of the seeds in the form of compound fertilizer for both maize and cowpea at 11.6 kg N ha−1, 10.6 kg P ha−1 and 9.6 kg K ha−1, respectively. Nitrogen top dressing was applied (as ammonium nitrate when soil moisture was adequate) to maize only at 4 and 8 weeks after emergence (WAE) in two equal splits of 23.1 kg N ha−1 each. However, in the 2019–2020 cropping season at both sites and in the 2020–2021 cropping season at UZF, the first N top dressing was delayed by an average of 4.5 and 2.0 weeks, respectively, due to mid-season dry spells. Ammonium nitrate was side dressed within 5 cm of the maize stems. Glyphosate [N-(phosphonomethyl) glycine], a pre-emergent nonselective herbicide, was applied at a rate of 1.025 L of active ingredient per hectare soon after sowing to control weeds. This was followed by manual weeding with a hoe whenever weeds reached a maximum of 10 cm height or 10 cm in diameter for stoloniferous weeds to achieve a weed clean field. More details about the experiment can be found in Shumba et al. (2023a) and Mhlanga et al. (2022b).
2.3 Soil sampling for bulk density determination and soil organic carbon analysis
Soil sampling was done in May 2021 at both sites. For each treatment and replicate, two sampling points in the maize rows and two sampling points in the middle of the inter-rows were randomly selected. The two samples from the rows were pooled into one sample per depth, similarly to the two samples taken in the inter-rows. The following nine depth increments were considered for both SOC and bulk density (BD) measurements: 0–5, 5–10, 10–15, 15–20, 20–30, 30–40, 40–50, 50–75 and 75–100 cm. Undisturbed soil samples for both SOC and BD measurements were taken from the following depth ranges using a metal cylinder (5 cm diameter and 5 cm height): 0–5, 5–10, 10–15, 15–20 and 20–30 cm. A motorized, hand-held soil corer with an inside diameter of 10 cm was used to take samples for the 30–40, 40–50, 50–75 and 75–100 cm depths for SOC analysis from the same positions where undisturbed samples were taken. As no significant differences in BD were found below 20 cm between the different treatments at the two sites (see Sect. 3) and to avoid too much destruction of the experimental plots, two soil pits were opened at the edges of the experimental plots (also cropped with maize since 2013) at each site to take BD samples for the 30–40, 40–50, 50–75 and 75–100 cm depths. As a result, BD below 30 cm depth was assumed to be the same across the treatments.
Soil samples were crumbled, and the fresh weight was determined using a field scale. Soil moisture was determined on a subsample by drying it in an oven at 105 ∘C for 48 h. All samples were then air-dried and sieved through a 2 mm sieve to determine the mass proportion of coarse soil (>2 mm) as a fraction of the whole sample. BD was determined by dividing the dry mass of soil by the volume of the cylinder. Subsamples from the ≤2 mm soil fraction were ground to <200 µm for SOC analysis. SOC was analysed in the ISO9001:2015-certified IRD LAMA laboratory in Dakar via the dry combustion of 100 mg aliquots of soil (ground to <200 µm) using a CHN elemental analyser (Thermo Finnigan FlashEA 1112, Milan, Italy).
2.4 Soil organic carbon stock calculation
The mass proportion of the coarse fraction (>2 mm) was removed to calculate SOC stocks. The equivalent soil mass (ESM) approach was used to determine SOC stocks to avoid systematic bias in the SOC calculation when using the fixed-depth method (Ellert and Bettany, 1995; Wendt and Hauser, 2013; von Haden et al., 2020). We defined reference soil mass profiles for each site based on the lowest cumulative soil mass obtained for each replicate. For these references, the cumulative soil mass layers were 0–650, 650–1340, 1340–2060, 2060–4160, 4160–5590, 5590–7040, 7040–10 550, 10 550–13 770 Mg soil ha−1 at DTC and 0–460, 460–870, 870–1330, 1330–1840, 1840–2760, 2760–4030, 4030–5300, 5300–8190, 8190–11 050 Mg soil ha−1 at UZF, roughly corresponding to soil depth layers of 0–5, 5–10, 10–15, 15–20, 20–30, 30–40, 40–50, 50–75, 75–100 cm, respectively. SOC stocks were calculated on the basis of the same soil mass as the reference profile but different soil depth layers that varied by <1.5 and <0.6 cm at DTC and UZF, respectively. As mulch was only applied between maize rows and fertilizer was only applied on maize rows, it was estimated that the row and inter-row space represented 22 % and 78 %, respectively; hence, SOC calculations were weighted accordingly (Shumba et al., 2023a). Change in cumulative SOC stock between treatments for a given soil depth was determined using the CT treatment as the reference treatment:
where SOC stocktreatment is the cumulative SOC stock per treatment (CTR, NT, NTM, NTR and NTMR) at a given soil layer and (i) represents 0–5, 0–10, 0–15, 0–20, 0–30, 0–40, 0–50, 50–75 or 75–100 cm.
SOC accumulation or loss rates () were calculated by dividing the change in stocks by the number of years between the establishment of the experiment and the time of soil sampling (8 years):
2.5 Estimation of organic carbon inputs to the soil
Maize and cowpea yield and aboveground biomass have been measured since the inception of the experiment, except for cowpea during the 2013–2014 season. This data gap was filled using the average cowpea yield and aboveground biomass values across seasons (from 2013–2014 to 2020–2021). We assumed that 5 % of the maize aboveground vegetative biomass remained in the field, as maize stalk slashing at harvesting did not remove the whole stem. A root : shoot ratio of 0.16 and 0.06 for maize and cowpea, respectively (Amos and Walters, 2006; Kahn and Schroeder, 1999; Kimiti, 2011), was used to estimate the contribution of roots to organic-C inputs to the soils. The organic-C input contribution from weeds was assumed to be insignificant, as there was effective control of weeds via the use of pre-emergence herbicide (glyphosate) and timely manual weeding throughout the cropping season. We also assumed that the relative amount of organic C transferred through rhizodeposition was the same for maize and cowpea, i.e. 0.45 × root C biomass (Balesdent et al., 2011), and that the organic-C content of all plant parts was 430 g kg−1 (Ma et al., 2018). Cumulative organic-C inputs to the soil were then estimated for each treatment (Cardinael et al., 2022).
2.6 Data analysis
The full dataset is available in the CIRAD repository (Shumba et al., 2023b). Statistical analyses were performed using R software, version 4.0.0 (R Core Team, 2020). Normality was tested by the Kolmogorov–Smirnov test. After confirming that data were normally distributed, analysis of variance (ANOVA) was carried out to establish any significant treatment effects on BD, SOC concentration and SOC stock. Separation of means was done with the post hoc Tukey test at a 5 % significance level using the emmeans function from the emmeans package (Bolker et al., 2009).
3.1 Soil bulk density
The different cropping systems (CT, CTR, NT, NTM, NTR and NTMR) had no significant (p>0.05) effect on BD across all soil depths except in the 5–10 cm depth in the inter-row at DTC (Fig. 2), where BD was on average 5 % lower in the conventional tillage treatments (CT and CTR) than in the no-tillage treatments (NT and NTR). However, soil depth and location (row or inter-row) as well as the soil depth × location interaction had significant (p<0.001) effects on BD. In the tillage layer (0–15 cm), BD was at least 2 % higher in the inter-rows than in the rows at both sites. In the deeper soil layer (15–30 cm), there were no significant (p>0.05) differences. BD values for depths below 30 cm were the same across treatments, as BD was determined from pits outside the experiment. It ranged between 1.47 and 1.51 and between 1.47 and 1.49 g cm−3 (Table S1 in the Supplement) in the subsoil (30–100 cm layers) at DTC and UZF, respectively.
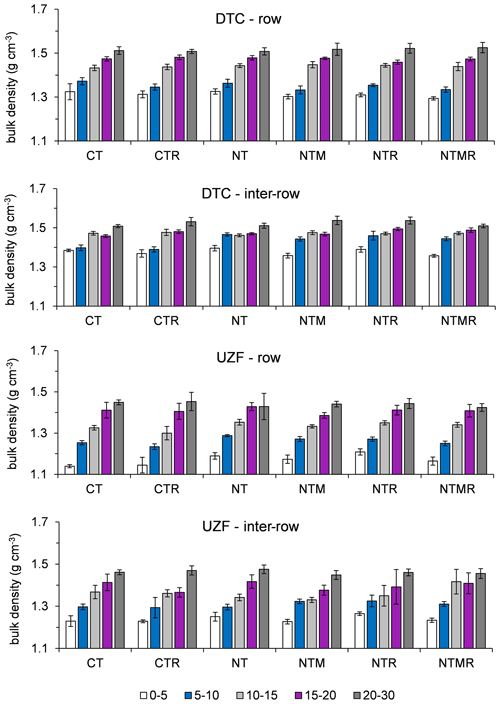
Figure 2Topsoil bulk density (0–30 cm) at the Domboshava Training Centre (DTC) and University of Zimbabwe Farm (UZF) experimental sites in Zimbabwe. The abbreviations used in the figure are as follows: CT – conventional tillage; CTR – conventional tillage with rotation; NT – no tillage; NTM – no tillage with mulch; NTR – no tillage with rotation; NTMR – no tillage with mulch and rotation. Error bars represent standard errors (N=4).
3.2 SOC concentration
The SOC concentration decreased significantly (p<0.001) with soil depth (Fig. 3, Table S2) and was highest in the surface soil (0–5 cm) for all treatments (Table S2). There were significant treatment effects in the 0–5 cm (p=0.001) and 5–10 cm (p=0.005) soil layers at DTC and in the 0–5 cm layer (p<0.001) at UZF. NTM had a significantly (p<0.05) higher SOC concentration compared with conventional tillage treatments (CT and CTR) and NT in the 0–5 cm soil layer at both sites (Fig. 3); the increase in the SOC concentration ranged between 31 % and 46 % and between 14 % and 22 % at DTC and UZF, respectively. However, the SOC concentration in the NTM treatment was equal (p>0.05) to the NTR and NTMR treatments at both sites.
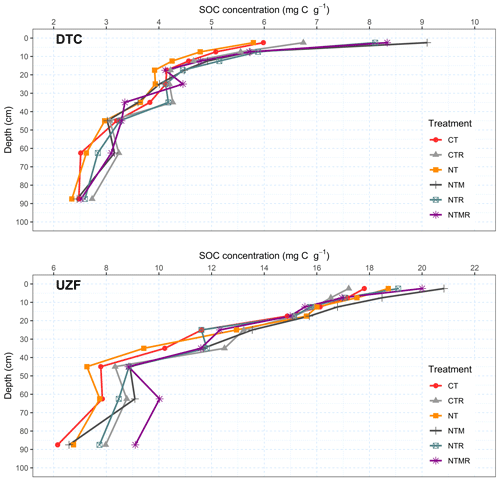
Figure 3Soil depth distribution of the organic-carbon concentration for the different experimental treatments at the Domboshava Training Centre (DTC) and University of Zimbabwe Farm (UZF) experimental sites in Zimbabwe. Error bars represent standard errors (N=4). The abbreviations used in the figure are as follows: CT – conventional tillage; CTR – conventional tillage with rotation; NT – no tillage; NTM – no tillage with mulch; NTR – no tillage with rotation; NTMR – no tillage with mulch and rotation.
In the 5–10 cm soil layer at DTC, SOC concentrations in the NTM and NTR treatments were at least 19 % higher (p=0.005) than in the NT and CT treatments (Table S2). There were no significant (p>0.05) treatment effects on the SOC concentration below 10 cm soil depth at DTC and below 5 cm depth at UZF.
3.3 SOC stock
There were significant (p<0.05) treatment effects on SOC stocks per soil layer in the top 5 cm at UZF and in the top 10 cm at DTC (Table S3). Compared with CT, CTR and NT, NTM had at least 1.1 and 1.3 times more SOC in the top 5 cm and top 10 cm layers at UZF and DTC, respectively. In terms of cumulative SOC stocks, significant (p<0.05) treatment effects were limited to the top 30 cm at DTC and the top 20 cm at UZF, where no tillage with mulching (NTM) increased SOC stocks (Table 1). On the other hand, there were no significant (p>0.05) tillage effects on SOC stocks (CT vs. NT) for either site. The rotation component had no significant (p>0.05) effects on SOC stocks when comparing CTR and NTR at DTC. However, the maize–cowpea rotation in the NT (NTR) treatments had at least 16 % higher SOC stocks in the top 30 cm compared with NT. In contrast, NTR had at least 7 % higher SOC stocks than CTR in the top 10 cm at UZF. Compared with NT and CT, the mulching component significantly (p<0.05) increased SOC stocks by at least 8 % at UZF and by at least 13 % at DTC in the top 20 cm and top 30 cm soil layers, respectively. SOC stocks in the full CA treatment (NTMR) were not significantly (p>0.05) different from the other combinations of CA principles (NTM and NTR) and CTR at DTC. At UZF, the full CA treatment had similar SOC stocks to all of the other NT treatments (NT, NTM and NTR).
Table 1Cumulative SOC stocks at the Domboshava Training Centre (DTC) and University of Zimbabwe Farm (UZF) after 8 years of different tillage, residue and crop management systems. Means in the same row followed by different superscript letters denote that values are significantly different, ns represents not significant, LSD represents least significant difference, and the associated errors are standard errors (N=4). The abbreviations used in the table are as follows: CT – conventional tillage; CTR – conventional tillage with rotation; NT – no tillage; NTM – no tillage with mulch; NTR – no tillage with rotation; NTMR – no tillage with mulch and rotation.
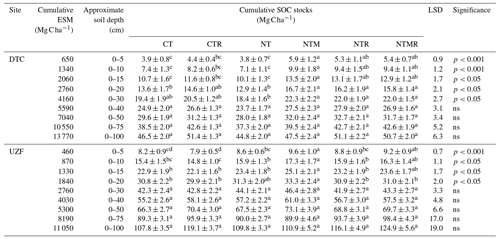
SOC stocks for the whole soil profile for this study (0–100 cm) were at least 8.1, 3.5 and 2.1 times higher at DTC and 11.6, 4.4 and 2.4 times higher at UZF than the SOC stocks in the respective 0–5 cm (surface soil), 0–15 cm (tillage depth) and 0–30 cm (IPCC standard depth) layers. SOC stocks for the subsoil (30–100 cm) ranged from 18.4 to 51.4 Mg C ha−1 at DTC and from 41.9 to 124.9 Mg C ha−1 at UZF. Therefore, subsoil represented more than half (at least 53 % at DTC and 58 % at UZF) of SOC stocks for the whole 100 cm soil profile.
3.4 SOC accumulation and loss rates
SOC accumulation rates at UZF differed significantly (p<0.05) with soil depth: topsoil layers (0–5, 0–10, 0–15, 0–20 and 0–30 cm) had SOC accumulation rates that were at least 6.9 times less than those in the 0–100 cm soil profile (Table 2). In contrast, there were no significant (p>0.05) differences in the SOC accumulation rates with depth at DTC. On average, SOC accumulation rates ranged between 0.13 and 0.08 in the topsoil (0–5 cm) to 0.33 and 1.16 for the whole 1 m soil profile at DTC and UZF, respectively. The depth and treatment interaction had no significant (p>0.05) effect at either site.
Table 2SOC change rates (± standard error, N=4) of the different treatments compared with CT (conventional tillage) at Domboshava Training Centre (DTC) and University of Zimbabwe farm (UZF). Means in the same row followed by different superscripts denote that values are significantly different, ns represents not significant, denotes p<0.05, denotes p<0.001 and LSD represents least significant difference. The abbreviations used in the table are as follows: CTR – conventional tillage with rotation; NT – no tillage; NTM – no tillage with mulch; NTR – no tillage with rotation; NTMR – no tillage with mulch and rotation.
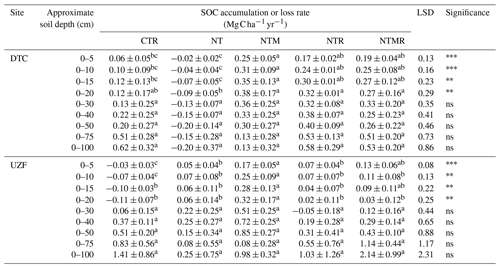
On the other hand, the different treatments in this study had significant (p<0.05) effects on SOC accumulation/loss rates in the top 20 cm soil layer at both sites (Table 2). At DTC, NT resulted in a significant (p<0.05) net loss of SOC in the 0–20 cm layer, ranging between −0.09 and −0.02 , whereas NT treatments (NTM, NTR and NTMR) had SOC accumulation rates ranging from 0.17 to 0.38 . However, maize stover mulching (NTM) resulted in significantly (p<0.05) higher SOC accumulation rates than the CTR (2.9–4.2 times) and NT (5.2–13.5 times) treatments in the top 15 cm and top 20 cm layers, respectively. The different combinations of mulching and rotation in the NT treatments (NTM, NTR and NTMR) resulted in no significant (p>0.05) differences in the SOC accumulation rates. Similarly, rotation treatments (CTR, NTR and NTMR) resulted in no significant (p>0.05) differences in SOC accumulation rates. Thus, the full CA treatment had similar SOC accumulations rates to treatments with at least two combinations of CA principles (NTM and NTR) and to CTR.
In contrast, CTR resulted in a significant (p<0.05) net loss of SOC in the top 20 cm at UZF (Table 2). The no-tillage treatments (NT, NTM, NTR and NTMR) resulted in significantly (p<0.05) higher SOC accumulation rates (0.05–0.25 ) than CTR, which ranged between −0.07 and −0.03 in top 10 cm soil layer. NTM resulted in the highest SOC accumulation rates (0.28–0.32 ) when considering the 0–15 and 0–20 cm soil layers. The SOC accumulation rates in the NTM treatment were at least 3.8, 3.5 and 2.4 times higher than CTR, NT and NTR, respectively, in the top 20 cm. The full CA treatment (NTMR) resulted in significantly (p<0.05) higher SOC accumulation rates compared with CTR (2.5–5.3 times) in the top 10 cm as well as in lower SOC accumulation rates in the 0–10 cm (2.3 times) and 0–20 cm (10.6 times) soil layers compared with NTM. However, there were no significant (p>0.05) differences in the SOC accumulation rates between treatments beyond the 20 cm soil layer at either site.
3.5 Organic-carbon inputs via crops residues, root mortality and rhizodeposition to the soil
There were significant (p<0.001) differences in cumulative organic-carbon (OC) inputs between treatments (Fig. 4). Cumulative OC inputs were at least 1.5 times higher in mulch treatments (NTM and NTMR) than in treatments without mulch. However, the mulch and rotation treatment (NTMR) had significantly (p<0.001) lower cumulative OC inputs than continuous mulching (NTM). Cumulative OC input after eight seasons was as high as 16.0 and 10.5 Mg C ha−1 at DTC and 16.2 and 12.4 Mg C ha−1 at UZF in the respective NTM and NTMR treatments (Fig. 4), resulting in mean annual OC input rates of about 1.3 to 1.6 for NTMR and 2.0 for NTM. The other treatments had mean annual OC input rates of ≤1.0 .
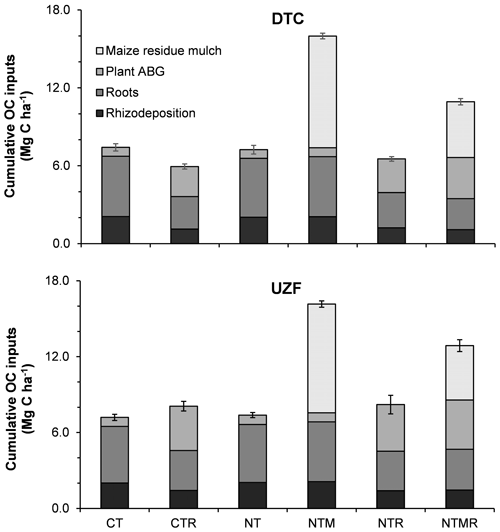
Figure 4Estimated cumulative organic-carbon (OC) inputs to the soil from the 2013–2014 to the 2020–2021 cropping season for the different treatments at the Domboshava Training Centre (DTC) and the University of Zimbabwe Farm (UZF) experimental sites. Error bars represent standard errors (n=4) for the cumulative OC. The abbreviations used in the figure are as follows: CT – conventional tillage; CTR – conventional tillage with rotation; NT – no tillage; NTM – no tillage with mulch; NTR – no tillage with rotation; NTMR – no tillage with mulch and rotation; ABG – aboveground biomass (corresponding to an estimated 5 % of the maize aboveground vegetative biomass remaining in the field, as maize stalk slashing at harvesting did not remove the whole stem).
4.1 SOC distribution across soil depth
Cumulative SOC stocks for the whole soil profile (0–100 cm) in this study were at least 8.0, 4.0 and 2.0 times higher than the respective SOC stocks in the 0–5 cm (surface soil), 0–15 cm (tillage depth for the study) and 0–30 cm (IPCC standard depth for SOC studies) layers at the two sites. This means that over half of the SOC stocks in this study were in the subsoil (30–100 cm), reflecting the importance of subsoil SOC stocks. Significant SOC stocks in the subsoil have also been reported by other authors (Yost and Hartemink, 2020; Balesdent et al., 2018; Cardinael et al., 2015; Harrison et al., 2011; Lal, 2018). Significant effects of mulch and/or rotation in the NT treatment were restricted to the top 30 cm in our study as well as in other studies in SSA (Dube et al., 2012; Powlson et al., 2016) and the world at large (Balesdent et al., 2018; Yost and Hartemink, 2020), which is most likely why the default soil depth for SOC studies according to the IPCC is 0–30 cm (IPCC, 2019). However, this underestimates whole-soil-profile C storage (Harrison et al., 2011; Singh et al., 2018; Lorenz and Lal, 2005). Therefore, it is crucial to consider whole-soil-profile sampling when monitoring SOC storage in agricultural ecosystems to determine their SOC sequestration potential in the pursuit of climate change mitigation (Malepfane et al., 2022). SOC mineralization is relatively low in the subsoil due to the lack of oxygen and physical protection of SOC (aggregate-protected C) (Rumpel et al., 2012; Sanaullah et al., 2016; Shumba et al., 2020; Button et al., 2022). Therefore, in order to improve subsoil (>30 cm) SOC stocks, derived from root mortality and exudates, crop varieties with higher root length densities (Chikowo et al., 2003) in the subsoil are recommended.
4.2 Cumulative SOC stocks and accumulation rates
Overall, the insignificant differences in the cumulative SOC stocks and SOC accumulation/loss rates between the different cropping systems in this and other studies (van der Pol et al., 2022; Leal et al., 2020) when considering whole soil profile (0–100 cm) allude to a dilution effect due to low OC inputs beyond 30 cm. Organic-C inputs in the subsoil (>30 cm) through crop root mortality and root exudates are highly limited due to low root biomass (Button et al., 2022; Chikowo et al., 2003). Several authors have also reported similar cumulative SOC stocks for a soil profile depth >30 cm between different tillage and residue management practices (Angers et al., 1997; Doran et al., 1998; Lal, 2015; Powlson et al., 2014). This can be associated with an accumulation of uncertainty when cumulating SOC stocks in several soil layers with their respective error of measurement. This weakens the ability to detect statistically significant differences, even where such differences exist (Kravchenko and Robertson, 2011). Kravchenko and Robertson (2011) bemoaned the lack of sufficient replication when sampling deep soil horizons due to its effect on the adequate representation of variability, and they also emphasized the importance of post hoc power analysis to reduce Type-II error. This study was limited to four replicates, which might not have enough statistical power to detect significant differences between treatments when considering the whole soil profile.
4.2.1 Mulching
The overarching role of mulching in cumulative SOC stocks and accumulation/loss rates at both sites (Tables 1, 2), albeit in the topsoil (<30 cm), has been shown in this study. Cumulative SOC stocks (Table 1) and SOC accumulation/loss rates (Table 2) did not differ with residue management under NT systems (NTM and NTMR) in the topsoil at DTC, regardless of high external OC inputs due to maize residue application in mulch treatments (Fig. 4, Table S4). This was attributed to the low clay content (<15 % clay) in the top 20 cm and, hence, low physical SOC protection (Chivenge et al., 2007; Mtambanengwe et al., 2004; Sun et al., 2020); thus, the differences in OC inputs had little effect. Alternatively, SOC can be protected from mineralization through adsorption to clay particles (Han et al., 2016; Churchman et al., 2020). However, there was low surface area for SOC adsorption due to the low clay content in the topsoil at DTC. Conversely, maize residue mulching effects were significant at UZF, although NTMR was not significantly different when compared with the other NT treatments. Cumulative OC inputs in the NTMR treatment (12.4 Mg C ha−1) were about 77 % of the cumulative OC inputs in the NTM treatment (16.2 Mg C ha−1) but at least 57 % higher than in the NT and NTR treatments after eight seasons (Fig. 4). This was associated with SOC adsorption and physical protection due to the relatively high clay content at UZF. Nonetheless, several studies have shown that aboveground biomass is less effective with respect to sustaining SOC stocks compared with belowground biomass (Hirte et al., 2018, 2021; Jones et al., 2009; Villarino et al., 2021), and we attribute that to the insignificant cumulative SOC stocks and accumulation rates between the NT treatments other than NTM, regardless of higher aboveground biomass in the NTR and NTMR treatments compared with NT (Fig. 4).
4.2.2 Tillage
Significant changes in SOC stocks and accumulation rates were restricted to the top 20 cm at both sites, below which there were no differences between treatments (Table 2). The consistently low SOC stocks in the NT, CT and CTR treatments and the low SOC accumulation rates (CTR and NT) at both sites were attributed to low OC inputs (Table S4, Fig. 4). The NT and CT treatments have had generally low yields in terms of grain and vegetative aboveground biomass (Mhlanga et al., 2021; Shumba et al., 2023a) since the establishment of the experiment in the 2013–2014 season and, in turn, low OC inputs from stubble, root mortality and root exudates. Our results dovetail with studies done elsewhere (Du et al., 2017; Koga and Tsuji, 2009) and with meta-analyses and reviews (Corbeels et al., 2020a; Lal, 2018, 2015) in which the authors have found that NT alone does not significantly improve SOC. However, higher SOC stocks were observed when NT was combined with at least two CA principles (mulching and rotation) at DTC in the top 20 cm (Table 1). It has been reported that NT cropping systems enhance SOC accumulation by increasing C inputs in the top layers and reducing erosion via minimum soil disturbance (Six et al., 2000; Lal, 2015, 2018; Bai et al., 2019; Cai et al., 2022). Minimum soil disturbance through NT also physically protects SOC in micro-aggregates from exposure to oxidative losses (Shumba et al., 2020; Six et al., 2002; Dolan et al., 2006; Liang et al., 2020). However, NT without mulch is a nonentity compared with other combinations of CA principles for long-term sustainability in cropping systems (Nyamangara et al., 2013; Kodzwa et al., 2020; Mhlanga et al., 2021; Li et al., 2020; Bohoussou et al., 2022), and NT is only effective for increasing SOC stocks when it is associated with other CA principles, especially mulch. Nevertheless, SOC stocks at UZF in the NT, NTR and NTMR treatments were similar, suggesting that rotation had no additional benefits when combined with NT. This can be explained by the low aboveground OC inputs in rotation treatments during the season when cowpeas were grown.
4.2.3 Maize–cowpea rotation
Legume rotations have been found to improve SOC accumulation rates and result in subsequent soil structural improvement (aggregation), induced by the addition of organic residues with a favourable ratio (Virk et al., 2022; Laub et al., 2023; Jephita et al., 2023). However, in our study, the benefits of cowpea rotation on SOC accumulation rates were not significant at UZF (Table 2). Nevertheless, the benefits of cowpea rotation under NT cropping systems (NTR and NTMR) compared with NT alone were significant, albeit in the top 20 cm, at DTC (Table 2). However, the net SOC loss in CTR at UZF was due to seasonal exposure to oxidative losses (SOC mineralization) via the disruption of soil macroaggregates by tillage, as alluded to by Bai et al. (2019), Cambardella and Elliott (1993), and Lal (2018). We underscore that maize–cowpea rotation in the NT treatment improved SOC accumulation in the topsoil due to reduced soil disturbance and alternate high-quality (cowpeas) and low-quality (maize) OC inputs. High-quality OC inputs have a positive priming effect (Chen et al., 2014) and have been shown to be preferentially stabilized in the soil due to a higher carbon use efficiency of soil microbes (Cotrufo et al., 2013; Kopittke et al., 2018). This explains the significant improvement in the SOC stocks under the combination of NT and alternate high- and low-quality OC inputs (maize–cowpea rotation) to the soil at DTC.
4.3 The role of soil texture in SOC accumulation
Soil texture has a widely recognized influence on SOC stocks (Sun et al., 2020) via the physical and chemical protection of SOC against microbially mediated decomposition (Chivenge et al., 2007; Mtambanengwe et al., 2004). In our study, the main difference between the two study sites is soil texture in the topsoil (0–30 cm): DTC had light-textured (sandy loam) soils and UZF had medium- to heavy-textured (sandy clay loam) soils (Fig. 1). These soil textural differences explain why there were no differences in SOC stock nor accumulation rates between NTM, NTR and NTMR at DTC, regardless of the higher cumulative OC inputs in the NTM and NTMR treatments (Fig. 4). The direct SOC inputs in the topsoil at DTC, where SOC was more concentrated (Table S2, Fig. 3), were subject to mineralization because of the low clay content and, thus, low protection of SOC due to soil micro-aggregates (Chivenge et al., 2007; Mtambanengwe et al., 2004; Sun et al., 2020); therefore, the differences in OC inputs had little effect. Light-textured soils have large pores that cannot protect SOC against microbial decomposition (Mtambanengwe et al., 2004; Christensen, 1987; Sun et al., 2020; Kravchenko and Guber, 2017). Additionally, the low clay content meant less surface area for SOC adsorption (Han et al., 2016; Churchman et al., 2020), which is another mechanism for SOC protection from mineralization. In contrast, there were significant differences between NTM and NTR at UZF in the topsoil layers, but there were no significant differences between NTM and NTMR. Cumulative OC inputs in the NTMR treatment (12.4 Mg C ha−1) were about 75 % of the cumulative OC inputs in the NTM treatment (16.2 Mg C ha−1) after eight seasons (Fig. 4). The added C, especially from maize stover mulch, was most likely protected by clay particles as well as by the formation of organo-mineral complexes (Malepfane et al., 2022; Chivenge et al., 2007; Jephita et al., 2023) that protect SOC from mineralization (Dunjana et al., 2012; Shumba et al., 2020; Button et al., 2022; Rumpel et al., 2012; Sanaullah et al., 2016).
Our study has shown the overarching importance of mulching and of the combination of at least two CA principles to improve top SOC stocks. No tillage (NT) alone did not increase SOC stocks, and this treatment even led to a slight decrease compared with CT, due to lower crop productivity in the NT treatment and, therefore, reduced OC inputs to the soil. Nevertheless, whole-profile (0–100 cm) SOC stocks were the same among all the treatments. Our study also showed that sampling of the entire soil profile is necessary for a more accurate understanding of the SOC accumulation potential among different cropping systems.
All data are freely available on the CIRAD data repository: https://doi.org/10.18167/DVN1/VPOCHN (Shumba et al., 2023b).
The supplement related to this article is available online at: https://doi.org/10.5194/soil-10-151-2024-supplement.
CT designed and established the experiments and has maintained them since 2013; RCa and RCh were involved in soil sampling campaigns; AS and RCa performed the statistical analyses, created the graphics and drafted the manuscript; AS, RCa, RCh, MC, JS and CT reviewed and edited the manuscript.
At least one of the (co-)authors is a member of the editorial board of SOIL. The peer-review process was guided by an independent editor, and the authors also have no other competing interests to declare.
Publisher's note: Copernicus Publications remains neutral with regard to jurisdictional claims made in the text, published maps, institutional affiliations, or any other geographical representation in this paper. While Copernicus Publications makes every effort to include appropriate place names, the final responsibility lies with the authors.
The authors are grateful to the International Maize and Wheat Improvement Center (CIMMYT) for setting up and operating the experimental sites. Moreover, we thank Britta Jahn-Humphrey for carrying out the gas analyses at ETH Zurich and Admire Muwati for his help with gas sampling. Special thanks go to the technical personnel at each of the experimental locations, namely, Tarirai Muoni, Sign Phiri, Herbert Chipara and Connie Madembo, who continuously assisted with the trial establishment and management.
This study was funded by the DSCATT project “Agricultural Intensification and Dynamics of Soil Carbon Sequestration in Tropical and Temperate Farming Systems” (grant nos. AF 1802-001 and FT C002181), supported by the Agropolis Fondation (“Programme d'Investissement d'Avenir” LabEx Agro, grant no. ANR-10-LABX-0001-01) and by the TOTAL Foundation (within a patronage agreement). Funding was also received from the donors of the MAIZE CGIAR research programme (https://www.maize.org, last access: 16 February 2024) and the Ukama Ustawi regional CGIAR initiative, who supported the trials up until 2018 and staff time up until 2023.
This paper was edited by Steven Sleutel and reviewed by two anonymous referees.
Amos, B. and Walters, D. T.: Maize Root Biomass and Net Rhizodeposited Carbon, Soil Sci. Soc. Am. J., 70, 1489–1503, https://doi.org/10.2136/sssaj2005.0216, 2006.
Angers, D. A. and Eriksen-Hamel, N. S.: Full-inversion tillage and organic carbon distribution in soil profiles: A meta-analysis, Soil Sci. Soc. Am. J., 72, 1370–1374, https://doi.org/10.2136/sssaj2007.0342, 2008.
Angers, D. A., Bolinder, M. A., Carter, M. R., Gregorich, E. G., Drury, C. F., Liang, B. C., Voroney, R. P., Simard, R. R., Donald, R. G., Bevaert, R. P., and Martel, J.: Impact of tillage practices on organic carbon and nitrogen storage in cool, humid soils of eastern Canada, Soil Till. Res., 41, 191–201, https://doi.org/10.4141/S96-111, 1997.
Bai, X., Huang, Y., Ren, W., Coyne, M., Jacinthe, P.-A., Bo, T., Hui, D., Yang, J., and Matocha, C.: Responses of soil carbon sequestration to climate-smart agriculture practices: A meta-analysis, Glob. Change Biol., 25, 2591–2606, https://doi.org/10.1111/gcb.14658, 2019.
Balesdent, J., Derrien, D., Fontaine, S., Kirman, S., Klumpp, K., Loiseau, P., Marol, C., Nguyen, C., Pean, M., Personeni, E., and Robin, C.: Contribution de la rhizodéposition aux matières organiques du sol, quelques implications pour la modélisation de la dynamique du carbone, Etude Gest. des sols, 18, 201–216, 2011.
Balesdent, J., Basile-Doelsch, I., Chadoeuf, J., Cornu, S., Derrien, D., Fekiacova, Z., and Hatté, C.: Atmosphere–soil carbon transfer as a function of soil depth, Nature, 559, 599–602, https://doi.org/10.1038/s41586-018-0328-3, 2018.
Bationo, A., Kihara, J., Vanlauwe, B., Waswa, B., Adolwa, I., and Saidou, K. (Eds.): Overview of Long Term Experiments in Africa, in: Lessons Learned from Long-Term Soil Fertility Management Experiments in Africa, Springer Science+Business Media, Dordrecht, 1–26, https://doi.org/10.1007/978-94-007-2938-4, 2013.
Baudron, F., Andersson, J. A., Corbeels, M., and Giller, K. E.: Failing to Yield? Ploughs, Conservation Agriculture and the Problem of Agricultural Intensification: An Example from the Zambezi Valley, Zimbabwe, J. Dev. Stud., 48, 393–412, https://doi.org/10.1080/00220388.2011.587509, 2012.
Bohoussou, Y. N. D., Kou, Y. H., Yu, W. B., Lin, B. J., Virk, A. L., Zhao, X., Dang, Y. P., and Zhang, H. L.: Impacts of the components of conservation agriculture on soil organic carbon and total nitrogen storage: A global meta-analysis, Sci. Total Environ., 842, 156822, https://doi.org/10.1016/J.SCITOTENV.2022.156822, 2022.
Bolker, B. M., Brooks, M. E., Clark, C. J., Geange, S. W., Poulsen, J. R., Stevens, M. H. H., and White, J. S. S.: Generalized linear mixed models: a practical guide for ecology and evolution, Trends Ecol. Evol., 24, 127–135, https://doi.org/10.1016/j.tree.2008.10.008, 2009.
Bossio, D. A., Cook-Patton, S. C., Ellis, P. W., Fargione, J., Sanderman, J., Smith, P., Wood, S., Zomer, R. J., von Unger, M., Emmer, I. M., and Griscom, B. W.: The role of soil carbon in natural climate solutions, Nat. Sustain., 3, 391–398, https://doi.org/10.1038/s41893-020-0491-z, 2020.
Button, E. S., Pett-Ridge, J., Murphy, D. V., Kuzyakov, Y., Chadwick, D. R., and Jones, D. L.: Deep-C storage: Biological, chemical and physical strategies to enhance carbon stocks in agricultural subsoils, Soil Biol. Biochem., 170, 108697, https://doi.org/10.1016/j.soilbio.2022.108697, 2022.
Cai, A., Han, T., Ren, T., Sanderman, J., Rui, Y., Wang, B., Smith, P., Xu, M., and Li, Y.: Declines in soil carbon storage under no tillage can be alleviated in the long run, Geoderma, 425, 1–3, https://doi.org/10.1016/j.geoderma.2022.116028, 2022.
Cambardella, C. A. and Elliott, E. T.: Carbon and Nitrogen Distribution in Aggregates from Cultivated and Native Grassland Soils, Soil Sci. Soc. Am. J., 57, 1071–1076, https://doi.org/10.2136/SSSAJ1993.03615995005700040032X, 1993.
Cardinael, R., Chevallier, T., Barthès, B. G., Saby, N. P. A., Parent, T., Dupraz, C., Bernoux, M., and Chenu, C.: Impact of alley cropping agroforestry on stocks, forms and spatial distribution of soil organic carbon – A case study in a Mediterranean context, Geoderma, 259–260, 288–299, https://doi.org/10.1016/j.geoderma.2015.06.015, 2015.
Cardinael, R., Guibert, H., Kouassi Brédoumy, S. T., Gigou, J., N'Goran, K. E., and Corbeels, M.: Sustaining maize yields and soil carbon following land clearing in the forest–savannah transition zone of West Africa: Results from a 20 year experiment, Field Crop. Res., 275, 108335, https://doi.org/10.1016/j.fcr.2021.108335, 2022.
Cheesman, S., Thierfelder, C., Eash, N. S., Kassie, G. T., and Frossard, E.: Soil carbon stocks in conservation agriculture systems of Southern Africa, Soil Till. Res., 156, 99–109, https://doi.org/10.1016/j.still.2015.09.018, 2016.
Chen, R., Senbayram, M., Blagodatsky, S., Myachina, O., Dittert, K., Lin, X., Blagodatskaya, E., and Kuzyakov, Y.: Soil C and N availability determine the priming effect: Microbial N mining and stoichiometric decomposition theories, Global Change Biol., 20, 2356–2367, https://doi.org/10.1111/gcb.12475, 2014.
Chikowo, R., Mapfumo, P., Nyamugafata, P., Nyamadzawo, G., and Giller, K. E.: Nitrate-N dynamics following improved fallows and maize root development in a Zimbabwean sandy clay loam, Agroforest. Syst., 59, 187–195, https://doi.org/10.1023/B:AGFO.0000005219.07409.a0, 2003.
Chivenge, P. P., Murwira, H. K., Giller, K. E., Mapfumo, P., and Six, J.: Long-term impact of reduced tillage and residue management on soil carbon stabilization: Implications for conservation agriculture on contrasting soils, Soil Till. Res., 94, 328–337, https://doi.org/10.1016/j.still.2006.08.006, 2007.
Christensen, B. T.: Decomposability of organic matter in particle size fractions from field soils with straw incorporation, Soil Biol. Biochem., 19, 429–435, https://doi.org/10.1016/0038-0717(87)90034-4, 1987.
Churchman, G. J., Singh, M., Schapel, A., Sarkar, B., and Bolan, N.: Clay Minerals As the Key To the Sequestration of Carbon in Soils, Clay. Clay Miner., 68, 135–143, https://doi.org/10.1007/s42860-020-00071-z, 2020.
Corbeels, M., Cardinael, R., Powlson, D., Chikowo, R., and Gerard, B.: Carbon sequestration potential through conservation agriculture in Africa has been largely overestimated: Comment on: “Meta-analysis on carbon sequestration through conservation agriculture in Africa,” Soil Till. Res., 196, 104300, https://doi.org/10.1016/j.still.2019.104300, 2020a.
Corbeels, M., Naudin, K., Whitbread, A. M., Kühne, R., and Letourmy, P.: Limits of conservation agriculture to overcome low crop yields in sub-Saharan Africa, Nat. Food, 1, 447–454, https://doi.org/10.1038/s43016-020-0114-x, 2020b.
Cotrufo, M. F., Wallenstein, M. D., Boot, C. M., Denef, K., and Paul, E.: The Microbial Efficiency-Matrix Stabilization (MEMS) framework integrates plant litter decomposition with soil organic matter stabilization: Do labile plant inputs form stable soil organic matter?, Global Change Biol., 19, 988–995, https://doi.org/10.1111/gcb.12113, 2013.
Dignac, M. F., Derrien, D., Barré, P., Barot, S., Cécillon, L., Chenu, C., Chevallier, T., Freschet, G. T., Garnier, P., Guenet, B., Hedde, M., Klumpp, K., Lashermes, G., Maron, P. A., Nunan, N., Roumet, C., and Basile-Doelsch, I.: Increasing soil carbon storage: mechanisms, effects of agricultural practices and proxies. A review, Agron. Sustain. Dev., 37, 14, https://doi.org/10.1007/s13593-017-0421-2, 2017.
Dolan, M. S., Clapp, C. E., Allmaras, R. R., Baker, J. M., and Molina, J. A. E.: Soil organic carbon and nitrogen in a Minnesota soil as related to tillage, residue and nitrogen management, Soil Till. Res., 89, 221–231, https://doi.org/10.1016/j.still.2005.07.015, 2006.
Doran, J. W., Elliott, E. T., and Paustian, K.: Soil microbial activity, nitrogen cycling, and long-term changes in organic carbon pools as related to fallow tillage management, Soil Till. Res., 49, 3–18, https://doi.org/10.1016/S0167-1987(98)00150-0, 1998.
Du, Z., Angers, D. A., Ren, T., Zhang, Q., and Li, G.: The effect of no-till on organic C storage in Chinese soils should not be overemphasized: A meta-analysis, Agr. Ecosyst. Environ., 236, 1–11, https://doi.org/10.1016/j.agee.2016.11.007, 2017.
Dube, E., Chiduza, C., and Muchaonyerwa, P.: Conservation agriculture effects on soil organic matter on a Haplic Cambisol after four years of maize-oat and maize-grazing vetch rotations in South Africa, Soil Till. Res., 123, 21–28, https://doi.org/10.1016/j.still.2012.02.008, 2012.
Dunjana, N., Nyamugafata, P., Shumba, A., Nyamangara, J., and Zingore, S.: Effects of cattle manure on selected soil physical properties of smallholder farms on two soils of Murewa, Zimbabwe, Soil Use Manage., 28, 221–228, https://doi.org/10.1111/j.1475-2743.2012.00394.x, 2012.
Ellert, B. H. and Bettany, J. R.: Calculation of organic matter and nutrients stored in soils under contrasting management regimes, Can. J. Soil Sci., 75, 529–538, https://doi.org/10.4141/cjss95-075, 1995.
Feller, C. and Beare, M. H.: Physical control of soil organic matter dynamics in the tropics, Geoderma, 79, 69–116, https://doi.org/10.1016/S0016-7061(97)00039-6, 1997.
Giller, K. E., Witter, E., Corbeels, M., and Tittonell, P.: Conservation agriculture and smallholder farming in Africa: The heretics' view, Field Crop. Res., 114, 23–34, https://doi.org/10.1016/j.fcr.2009.06.017, 2009.
Giller, K. E., Andersson, J. A., Corbeels, M., Kirkegaard, J., Mortensen, D., Erenstein, O., and Vanlauwe, B.: Beyond conservation agriculture, Front. Plant Sci., 6, 1–14, https://doi.org/10.3389/fpls.2015.00870, 2015.
Han, L., Sun, K., Jin, J., and Xing, B.: Some concepts of soil organic carbon characteristics and mineral interaction from a review of literature, Soil Biol. Biochem., 94, 107–121, https://doi.org/10.1016/j.soilbio.2015.11.023, 2016.
Harrison, R. B., Footen, P. W., Harrison, R. B., Footen, P. W., and Strahm, B. D.: Deep Soil Horizons: Contribution and Importance to Soil Carbon Pools and in Assessing Whole-Ecosystem Response to Management and Global Change, For. Sci., 57, 67–76, 2011.
Hirte, J., Leifeld, J., Abiven, S., Oberholzer, H. R., and Mayer, J.: Below ground carbon inputs to soil via root biomass and rhizodeposition of field-grown maize and wheat at harvest are independent of net primary productivity, Agr. Ecosyst. Environ., 265, 556–566, https://doi.org/10.1016/j.agee.2018.07.010, 2018.
Hirte, J., Walder, F., Hess, J., Büchi, L., Colombi, T., van der Heijden, M. G., and Mayer, J.: Enhanced root carbon allocation through organic farming is restricted to topsoils, Sci. Total Environ., 755, 143551, https://doi.org/10.1016/j.scitotenv.2020.143551, 2021.
IPCC: 2019 Refinement to the 2006 IPCC Guidelines for National Greenhouse Gas Inventories, edited by: Calvo Buendia, E., Tanabe, K., Kranjc, A., Baasansuren, J., Fukuda, M., Ngarize, S., Osako, A., Pyrozhenko, Y., Shermanau, P. and Federici, S., IPCC, Switzerland, ISBN 978-4-88788-232-4, 2019.
Jephita, G., Jefline, K., Willis, G., and Justice, N.: Carbon stock, aggregate stability and hydraulic properties of soils under tillage, crop rotation and mineral fertiliser application in sub-humid Zimbabwe, Heliyon, 9, Heliyon, e15846, https://doi.org/10.1016/j.heliyon.2023.e15846, 2023.
Jones, D. L., Nguyen, C., and Finlay, R. D.: Carbon flow in the rhizosphere: carbon trading at the soil – root interface, Plant Soil, 321, 5–33, https://doi.org/10.1007/s11104-009-9925-0, 2009.
Kahn, B. A. and Schroeder, J. L.: Root Characteristics and Seed Yields of Cowpeas Grown with and without Added Nitrogen Fertilizer, HortScience, 34, 1238–1239, https://doi.org/10.21273/hortsci.34.7.1238, 1999.
Kassam, A., Friedrich, T., and Derpsch, R.: Global spread of Conservation Agriculture, Int. J. Environ. Stud., 76, 29–51, https://doi.org/10.1080/00207233.2018.1494927, 2019.
Kell, D. B.: Breeding crop plants with deep roots: their role in sustainable carbon, nutrient and water sequestration, Ann. Bot.-London, 108, 407–418, https://doi.org/10.1093/aob/mcr175, 2011.
Kimaro, A. A., Mpanda, M., Rioux, J., Aynekulu, E., Shaba, S., Thiong'o, M., Mutuo, P., Abwanda, S., Shepherd, K., Neufeldt, H., and Rosenstock, T. S.: Is conservation agriculture “climate-smart” for maize farmers in the highlands of Tanzania?, Nutr. Cycl. Agroecosys., 105, 217–228, https://doi.org/10.1007/s10705-015-9711-8, 2016.
Kimiti, J. M.: Influence of integrated soil nutrient management on cowpea root growth in the semi-arid Eastern Kenya, Afr. J. Agr. Res., 6, 3084–3091, 2011.
Kodzwa, J. J., Gotosa, J., and Nyamangara, J.: Mulching is the most important of the three conservation agriculture principles in increasing crop yield in the short term, under sub humid tropical conditions in Zimbabwe, Soil Till. Res., 197, 104515, https://doi.org/10.1016/j.still.2019.104515, 2020.
Koga, N. and Tsuji, H.: Effects of reduced tillage, crop residue management and manure application practices on crop yields and soil carbon sequestration on an Andisol in northern Japan, Soil Sci. Plant Nutr., 55, 546–557, https://doi.org/10.1111/j.1747-0765.2009.00385.x, 2009.
Kopittke, P. M., Hernandez-Soriano, M. C., Dalal, R. C., Finn, D., Menzies, N. W., Hoeschen, C., and Mueller, C. W.: Nitrogen-rich microbial products provide new organo-mineral associations for the stabilization of soil organic matter, Global Change Biol., 24, 1762–1770, https://doi.org/10.1111/gcb.14009, 2018.
Kravchenko, A. N. and Guber, A. K.: Soil pores and their contributions to soil carbon processes, Geoderma, 287, 31–39, https://doi.org/10.1016/j.geoderma.2016.06.027, 2017.
Kravchenko, A. N. and Robertson, G. P.: Whole-Profile Soil Carbon Stocks: The Danger of Assuming Too Much from Analyses of Too Little, Soil Sci. Soc. Am. J., 75, 235–240, https://doi.org/10.2136/sssaj2010.0076, 2011.
Lal, R.: Residue management, conservation tillage and soil restoration for mitigating greenhouse effect by CO2-enrichment, Soil Till. Res., 43, 81–107, 1997.
Lal, R.: Sequestering carbon and increasing productivity by conservation agriculture, J. Soil Water Conserv., 70, 55A–62A, https://doi.org/10.2489/jswc.70.3.55A, 2015.
Lal, R.: Digging deeper: A holistic perspective of factors affecting soil organic carbon sequestration in agroecosystems, Glob. Change Biol., 24, 3285–3301, https://doi.org/10.1111/gcb.14054, 2018.
Laub, M., Corbeels, M., Couëdel, A., Ndungu, S. M., Mucheru-Muna, M. W., Mugendi, D., Necpalova, M., Waswa, W., Van de Broek, M., Vanlauwe, B., and Six, J.: Managing soil organic carbon in tropical agroecosystems: evidence from four long-term experiments in Kenya, SOIL, 9, 301–323, https://doi.org/10.5194/soil-9-301-2023, 2023.
Leal, O. A., Amado, T. J. C., Fiorin, J. E., Keller, C., Reimche, G. B., Rice, C. W., Nicoloso, R. S., Bortolotto, R. P., and Schwalbert, R.: Linking cover crop residue quality and tillage system to CO2-C emission, soil C and N stocks and crop yield based on a long-term experiment, Agronomy, 10, 1848, https://doi.org/10.3390/agronomy10121848, 2020.
Li, Y., Li, Z., Chang, S. X., Cui, S., Jagadamma, S., Zhang, Q., and Cai, Y.: Residue retention promotes soil carbon accumulation in minimum tillage systems: Implications for conservation agriculture, Sci. Total Environ., 740, 140147, https://doi.org/10.1016/j.scitotenv.2020.140147, 2020.
Liang, B. C., VandenBygaart, A. J., MacDonald, J. D., Cerkowniak, D., McConkey, B. G., Desjardins, R. L., and Angers, D. A.: Revisiting no-till's impact on soil organic carbon storage in Canada, Soil Till. Res., 198, 104529, https://doi.org/10.1016/j.still.2019.104529, 2020.
Lorenz, K. and Lal, R.: The Depth Distribution of Soil Organic Carbon in Relation to Land Use and Management and the Potential of Carbon Sequestration in Subsoil Horizons, Adv. Agron., 88, 35–66, https://doi.org/10.1016/S0065-2113(05)88002-2, 2005.
Ma, S., He, F., Tian, D., Zou, D., Yan, Z., Yang, Y., Zhou, T., Huang, K., Shen, H., and Fang, J.: Variations and determinants of carbon content in plants: a global synthesis, Biogeosciences, 15, 693–702, https://doi.org/10.5194/bg-15-693-2018, 2018.
Malepfane, N. M., Muchaonyerwa, P., Hughes, J. C., and Zengeni, R.: Land use and site effects on the distribution of carbon in some humic soil profiles of KwaZulu-Natal, South Africa, Heliyon, 8, e08709, https://doi.org/10.1016/j.heliyon.2021.e08709, 2022.
Mapanda, F., Mupini, J., Wuta, M., Nyamangara, J., and Rees, R. M.: A cross-ecosystem assessment of the effects of land cover and land use on soil emission of selected greenhouse gases and related soil properties in Zimbabwe, Eur. J. Soil Sci., 61, 721–733, https://doi.org/10.1111/j.1365-2389.2010.01266.x, 2010.
Mbanyele, V., Mtambanengwe, F., Nezomba, H., Groot, J. C. J., and Mapfumo, P.: Comparative short-term performance of soil water management options for increased productivity of maize-cowpea intercropping in semi-arid Zimbabwe, J. Agric. Food Res., 5, 100189, https://doi.org/10.1016/j.jafr.2021.100189, 2021.
Mhlanga, B., Ercoli, L., Pellegrino, E., Onofri, A., and Thierfelder, C.: The crucial role of mulch to enhance the stability and resilience of cropping systems in southern Africa, Agron. Sustain. Dev., 41, 29, https://doi.org/10.1007/s13593-021-00687-y, 2021.
Mhlanga, B., Ercoli, L., Thierfelder, C., and Pellegrino, E.: Conservation agriculture practices lead to diverse weed communities and higher maize grain yield in Southern Africa, Field Crop. Res., 289, 108724, https://doi.org/10.1016/j.fcr.2022.108724, 2022a.
Mhlanga, B., Pellegrino, E., Thierfelder, C., and Ercoli, L.: Conservation agriculture practices drive maize yield by regulating soil nutrient availability, arbuscular mycorrhizas, and plant nutrient uptake, Field Crop. Res., 277, 108403, https://doi.org/10.1016/j.fcr.2021.108403, 2022b.
Minasny, B., Malone, B. P., McBratney, A. B., Angers, D. A., Arrouays, D., Chambers, A., Chaplot, V., Chen, Z. S., Cheng, K., Das, B. S., Field, D. J., Gimona, A., Hedley, C. B., Hong, S. Y., Mandal, B., Marchant, B. P., Martin, M., McConkey, B. G., Mulder, V. L., O'Rourke, S., Richer-de-Forges, A. C., Odeh, I., Padarian, J., Paustian, K., Pan, G., Poggio, L., Savin, I., Stolbovoy, V., Stockmann, U., Sulaeman, Y., Tsui, C. C., Vågen, T. G., van Wesemael, B., and Winowiecki, L.: Soil carbon 4 per mille, Geoderma, 292, 59–86, https://doi.org/10.1016/j.geoderma.2017.01.002, 2017.
Mtambanengwe, F., Mapfumo, P., and Kirchmann, H.: Decomposition of organic matter in soil as influenced by texture and pore size distribution, in: Managing Nutrient Cycles to Sustain Soil Fertility in Sub-Saharan Africa, edited by: Bationo, A., Academy Science Publishers and TSBF CIAT, Nairobi, 261–276, ISBN 9966240756, 2004.
Nyamangara, J., Masvaya, E. N., Tirivavi, R., and Nyengerai, K.: Effect of hand-hoe based conservation agriculture on soil fertility and maize yield in selected smallholder areas in Zimbabwe, Soil Till. Res., 126, 19–25, https://doi.org/10.1016/j.still.2012.07.018, 2013.
Patra, S., Julich, S., Feger, K. H., Jat, M. L., Sharma, P. C., and Schwärzel, K.: Effect of conservation agriculture on stratification of soil organic matter under cereal-based cropping systems, Arch. Agron. Soil Sci., 65, 2013–2028, https://doi.org/10.1080/03650340.2019.1588462, 2019.
Paustian, K., Lehmann, J., Ogle, S., Reay, D., Robertson, G. P., and Smith, P.: Climate-smart soils, Nature, 532, 49–57, https://doi.org/10.1038/nature17174, 2016.
Powlson, D. S., Stirling, C. M., Jat, M. L., Gerard, B. G., Palm, C. A., Sanchez, P. A., and Cassman, K. G.: Limited potential of no-till agriculture for climate change mitigation, Nat. Clim. Change, 4, 678–683, https://doi.org/10.1038/nclimate2292, 2014.
Powlson, D. S., Stirling, C. M., Thierfelder, C., White, R. P., and Jat, M. L.: Does conservation agriculture deliver climate change mitigation through soil carbon sequestration in tropical agro-ecosystems?, Agr. Ecosyst. Environ., 220, 164–174, https://doi.org/10.1016/j.agee.2016.01.005, 2016.
R Core Team: R: A Language and Environment for Statistical Computing, R Foundation for Statistical Computing, Vienna, Austria, https://www.R-project.org/ (last access: 14 February 2024), 2020.
Rumpel, C., Chabbi, A., and Marschner, B.: Carbon Storage and Sequestration in Subsoil Horizons: Knowledge, Gaps and Potentials, in: Recarbonization of the Biosphere: Ecosystems and the Global Carbon Cycle, edited by: Lal, R., Lorenz, K., Hüttl, R. F., Schneider, B. U., and Von Braun, J., Springer Science+Business Media B.V, 445–464, https://doi.org/10.1007/978-94-007-4159-1, 2012.
Sanaullah, M., Chabbi, A., Maron, P. A., Baumann, K., Tardy, V., Blagodatskaya, E., Kuzyakov, Y., and Rumpel, C.: How do microbial communities in top- and subsoil respond to root litter addition under field conditions?, Soil Biol. Biochem., 103, 28–38, https://doi.org/10.1016/j.soilbio.2016.07.017, 2016.
Shumba, A., Dunjana, N., Nyamasoka, B., Nyamugafata, P., Madyiwa, S., and Nyamangara, J.: Maize (Zea mays) yield and its relationship to soil properties under integrated fertility, mulch and tillage management in urban agriculture, South African J. Plant Soil, 37, 1–10, https://doi.org/10.1080/02571862.2019.1678686, 2020.
Shumba, A., Chikowo, R., Corbeels, M., Six, J., Thierfelder, C., and Cardinael, R.: Long-term tillage, residue management and crop rotation impacts on N2O and CH4 emissions on two contrasting soils in sub-humid Zimbabwe, Agr. Ecosyst. Environ., 341, 108207, https://doi.org/10.1016/j.agee.2022.108207, 2023a.
Shumba, A., Chikowo, R., Thierfelder, C., Corbeels, M., Six, J., and Cardinael, R.: Data for “Mulch application as the overarching factor explaining increase in soil organic carbon stocks under conservation agriculture in two 8 year-old experiments in Zimbabwe”, https://doi.org/10.18167/DVN1/VPOCHN, CIRAD Dataverse, 2023b.
Singh, G., Schoonover, J. E., Williard, K. W. J., Kaur, G., and Crim, J.: Carbon and Nitrogen Pools in Deep Soil Horizons at Different Landscape Positions, Soil Sci. Soc. Am. J., 82, 1512–1525, https://doi.org/10.2136/sssaj2018.03.0092, 2018.
Six, J., Paustian, K., and Elliott, E.: Aggregate and soil organic matter dynamics under conventional and no-tillage systems, Soil Sci. Soc. Am. J., 63, 1350–1358, 1999.
Six, J., Elliott, E. T., and Paustian, K.: Soil macroaggregate turnover and microaggregate formation: A mechanism for C sequestration under no-tillage agriculture, Soil Biol. Biochem., 32, 2099–2103, https://doi.org/10.1016/S0038-0717(00)00179-6, 2000.
Six, J., Conant, R. T., Paul, E. A., and Paustian, K.: Stabilization mechanisms of soil organic matter: Implications for C-saturation of soils, Plant Soil, 241, 155–176, https://doi.org/10.1023/a:1016125726789, 2002.
Sun, W., Canadell, J. G., Yu, L. L., Yu, L. L., Zhang, W., Smith, P., Fischer, T., and Huang, Y.: Climate drives global soil carbon sequestration and crop yield changes under conservation agriculture, Global Change Biol., 26, 3325–3335, https://doi.org/10.1111/gcb.15001, 2020.
Swanepoel, C. M., van der Laan, M., Weepener, H. L., du Preez, C. C., and Annandale, J. G.: Review and meta-analysis of organic matter in cultivated soils in southern Africa, Nutr. Cycl. Agroecosys., 104, 107–123, https://doi.org/10.1007/s10705-016-9763-4, 2016.
Swanepoel, C. M., Rötter, R. P., van der Laan, M., Annandale, J. G., Beukes, D. J., du Preez, C. C., Swanepoel, L. H., van der Merwe, A., and Hoffmann, M. P.: The benefits of conservation agriculture on soil organic carbon and yield in southern Africa are site-specific, Soil Till. Res., 183, 72–82, https://doi.org/10.1016/j.still.2018.05.016, 2018.
Thierfelder, C. and Mhlanga, B.: Short-term yield gains or long-term sustainability? – a synthesis of Conservation Agriculture long-term experiments in Southern Africa, Agr. Ecosyst. Environ., 326, 107812, https://doi.org/10.1016/j.agee.2021.107812, 2022.
Thierfelder, C. and Wall, P. C.: Effects of conservation agriculture techniques on infiltration and soil water content in Zambia and Zimbabwe, Soil Till. Res., 105, 217–227, https://doi.org/10.1016/j.still.2009.07.007, 2009.
Thierfelder, C. and Wall, P. C.: Effects of conservation agriculture on soil quality and productivity in contrasting agro-ecological environments of Zimbabwe, Soil Use Manage., 28, 209–220, https://doi.org/10.1111/j.1475-2743.2012.00406.x, 2012.
Thierfelder, C., Matemba-Mutasa, R., and Rusinamhodzi, L.: Yield response of maize (Zea mays L.) to conservation agriculture cropping system in Southern Africa, Soil Till. Res., 146, 230–242, https://doi.org/10.1016/j.still.2014.10.015, 2015.
Thierfelder, C., Chivenge, P., Mupangwa, W., Rosenstock, T. S., Lamanna, C., and Eyre, J. X.: How climate-smart is conservation agriculture (CA)? – its potential to deliver on adaptation, mitigation and productivity on smallholder farms in southern Africa, Food Secur., 9, 537–560, https://doi.org/10.1007/s12571-017-0665-3, 2017.
Thierfelder, C., Baudron, F., Setimela, P., Nyagumbo, I., Mupangwa, W., Mhlanga, B., Lee, N., and Gérard, B.: Complementary practices supporting conservation agriculture in southern Africa. A review, Agron. Sustain. Dev., 38, 16, https://doi.org/10.1007/s13593-018-0492-8, 2018.
Thorup-Kristensen, K., Halberg, N., Nicolaisen, M., Olesen, J. E., Crews, T. E., Hinsinger, P., Kirkegaard, J., Pierret, A., and Dresbøll, D. B.: Digging Deeper for Agricultural Resources, the Value of Deep Rooting, Trends Plant Sci., 25, 406–417, https://doi.org/10.1016/j.tplants.2019.12.007, 2020.
van der Pol, L. K., Robertson, A., Schipanski, M., Calderon, F. J., Wallenstein, M. D., and Cotrufo, M. F.: Addressing the soil carbon dilemma: Legumes in intensified rotations regenerate soil carbon while maintaining yields in semi-arid dryland wheat farms, Agr. Ecosyst. Environ., 330, 107906, https://doi.org/10.1016/j.agee.2022.107906, 2022.
Villarino, S. H., Pinto, P., Jackson, R. B., and Piñeiro, G.: Plant rhizodeposition: A key factor for soil organic matter formation in stable fractions, Sci. Adv., 7, 1–14, https://doi.org/10.1126/sciadv.abd3176, 2021.
Virk, A. L., Lin, B. J., Kan, Z. R., Qi, J. Y., Dang, Y. P., Lal, R., Zhao, X., and Zhang, H. L.: Simultaneous effects of legume cultivation on carbon and nitrogen accumulation in soil, Adv. Agron., 171, 75–110, https://doi.org/10.1016/bs.agron.2021.08.002, 2022.
von Haden, A. C., Yang, W. H., and DeLucia, E. H.: Soils' dirty little secret: Depth-based comparisons can be inadequate for quantifying changes in soil organic carbon and other mineral soil properties, Global Change Biol., 26, 3759–3770, https://doi.org/10.1111/gcb.15124, 2020.
Wendt, J. W. and Hauser, S.: An equivalent soil mass procedure for monitoring soil organic carbon in multiple soil layers, Eur. J. Soil Sci., 64, 58–65, https://doi.org/10.1111/ejss.12002, 2013.
Yang, L., Luo, Y., Lu, B., Zhou, G., Chang, D., Gao, S., Zhang, J., Che, Z., and Cao, W.: Long-term maize and pea intercropping improved subsoil carbon storage while reduced greenhouse gas emissions, Agr. Ecosyst. Environ., 349, 108444, https://doi.org/10.1016/J.AGEE.2023.108444, 2023.
Yost, J. L. and Hartemink, A. E.: How deep is the soil studied – an analysis of four soil science journals, Plant Soil, 452, 5–18, https://doi.org/10.1007/s11104-020-04550-z, 2020.