the Creative Commons Attribution 4.0 License.
the Creative Commons Attribution 4.0 License.
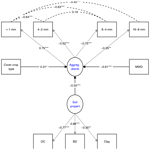
Cover crops improve soil structure and change organic carbon distribution in macroaggregate fractions
Norman Gentsch
Florin Laura Riechers
Jens Boy
Dörte Schweneker
Ulf Feuerstein
Diana Heuermann
Georg Guggenberger
Soil structure is sensitive to intensive soil management. It can be ameliorated by a reduction in soil cultivation and stimulation of plant and microbial mediators for aggregate formation, with the latter being a prerequisite and measure for soil quality. Cover crops (CCs) are part of an integrated approach to stabilize or improve soil quality. Thereby, the incorporation of diverse CC mixtures is hypothesized to increase the positive effects of CC applications. This study entailed an investigation of the legacy effect of CCs on soil aggregates after three crop rotations in the second main crop (winter wheat) after the last CC treatment. Four CCs (mustard, phacelia, clover, and oat) cultivated in pure stands and with a fallow treatment were compared to a mixture of the four CC species (Mix4) and a highly diverse 12-plant-species mixture (Mix12) in a long-term field experiment in Germany. The organic carbon (OC) distribution within macroaggregate fractions (16–8, 8–4, 4–2, 2–1, and <1 mm) and their aggregate stability were measured by dry- and wet-sieving methods, and the mean weight diameter (MWD) was calculated from water-stable aggregates.
The results showed that, compared to the fallow, all CCs increased the MWD between 10 % and 19 % in soil under the following main crop. The average MWD increase over the fallow was slightly higher for CC mixtures (16 %) than for single CCs (12 %). Most of the OC (67.9 % on average) was stored in the <1 mm aggregate fraction, highest in the topsoil and decreasing with soil depth. The intermediate fractions (8–4 mm, 4–2 mm, 2–1 mm) stored 8.5 %, 10.5 %, and 11.0 % of the total OC, while 2.1 % was stored in the 16–8 mm fraction. Higher MWD improvement at the 20–30 cm depth also indicates additional benefits from a reduction in the cultivation depth. Structural equation modelling (SEM) suggests that single CCs were more likely to increase OC storage in small macroaggregates <1 mm, while CC mixtures were more likely to increase OC in the largest fraction (8–16 mm). Different individual CC species or mixtures exhibited varying involvement in the formation of different aggregate fractions. We provide evidence that litter quality, root morphology, and rhizosphere input, which affect microbial mediators of aggregate formation, might be the main reasons for the observed differences between CC treatments. Cover crops are valuable multifunctional tools for sustainable soil management. Here, we showed that they contribute to structure amelioration in arable soils. Increasing the functional diversity of plant species in CC mixtures could be a strategy to further enhance the positive effects of CCs in agroecosystems.
- Article
(1744 KB) - Full-text XML
-
Supplement
(2652 KB) - BibTeX
- EndNote
Restoring soil structure is one of the most important tasks in terms of sustainable soil management and adaptation of cropping systems to climate change (Lal, 2015; Williams and Petticrew, 2009; Obalum et al., 2017). In recent decades, soil structure degradation has been widespread and has been observed due to the loss of aggregate stability and changes in aggregate size distribution towards smaller aggregate classes (Boix-Fayos et al., 2001). The causes of soil structure degradation have been attributed to intensification of soil cultivation (Williams and Petticrew, 2009), loss of organic matter (OM) and soil biological functions (Obalum et al., 2017), and unilateral crop rotation and mineral fertilization (Lal, 2009). Aggregate stability can provide important information about soil functioning, which defines soil quality and soil health in agroecosystems (Seybold and Herrick, 2001; J. Lehmann et al., 2020). Stable soil aggregates are more stress resistant, decrease soil erodibility, and increase the OM protection capacity (Dungait et al., 2012; Six et al., 2000) and climate resilience of soils (Allen et al., 2011; Six and Paustian, 2014).
Aggregation and the arrangement of the aggregates into a defined pattern, i.e. the soil structure, depend on several parameters and are a product of the interactions between the quantity and quality of organic residues entering the soil, indigenous soil organic matter (OM), mineral constituents, soil organisms, and land use history (Tisdall and Oades, 1982). Plants are of particular importance in the management of the soil structure, as the farmer — with the selection of the cultivated plant — can directly intervene in plant-microbial-mediated soil processes. There are four pathways through which plants can directly and indirectly influence aggregate formation: (1) functional root characteristics such as root morphologies vary between plant species and differently affect soil parameters, such as soil pore connectivity, (macro)porosity, or aggregate stability (Kutschera et al., 2009; Bacq-Labreuil et al., 2019; Hudek et al., 2022). (2) Litter quality controls the decomposition of root and shoot litter and provides polysaccharides as binding agents for aggregates (Liu et al., 2005). (3) Rhizodeposits (root fragments, cell debris, exudates, mucilage) can act as binding materials. (4) Plants have a strong impact on soil biota by shaping the microbial community with their rhizosphere inputs (Reinhold-Hurek et al., 2015). Soil biota is a key factor for aggregate formation, where bacteria and fungi appear to be more important than soil fauna (Lehmann et al., 2017). In this context, the sequence of cultivated plants in crop rotation plays a crucial role in the legacy of microbial processes and OM inputs from litter, roots, or microbial residues in arable soils. Therefore, crop rotation and crop history have a strong impact on aggregate formation and stability (Wright and Anderson, 2000; Zhou et al., 2020) and appear to be important tools for soil structure amelioration in arable systems.
Cover crops (CCs) are cultivated for purposes of soil degradation protection, nutrient leaching, and remediation of soil quality (Thorup-Kristensen et al., 2003; Williams and Petticrew, 2009). The CC biomass is either harvested as fodder for energy production or acts as green manure for the following crop. Previous studies have demonstrated strong positive impacts of CCs on soil structure formation and aggregate stability (Mendes et al., 1999; Dabney et al., 2001; Liu et al., 2005; Blanco-Canqui and Ruis, 2020; Stegarescu et al., 2021). Moreover, root activity was found to be the major driver of higher aggregate stability during CC growth (Stegarescu et al., 2021). A recent study examined functional root traits as a basis for classifying seven different cover crops to improve physical soil properties, including aggregate stability (Hudek et al., 2022). The authors demonstrated that the positive effect of macroporosity and aggregate stability during CC growth depended on the morphology of the individual plant root systems. Four out of seven tested species showed positive trends.
Biodiverse CC mixtures can compensate for the weaknesses of single components and improve the multifunctional positive effects of CC (Couëdel et al., 2019). Cover crop mixtures can have higher root biomass than single components (Heuermann et al., 2019), increase the rhizosphere C input, and thereby stimulate microbial biomass and activity (Gentsch et al., 2020; Chavarría et al., 2016). The biogeochemical cycling rate and particularly the fungal community composition appeared to be strongly affected by CC species or mixtures (Cloutier et al., 2020; Thapa et al., 2021; Gentsch et al., 2020). Furthermore, litter from multispecies CC crop mixtures increased the molecular diversity of OM inputs to the soil and the number of substrate niches for microbes (Drost et al., 2020). In concluding the recent research on CC mixtures as compared to single-CC cultivars, all four pathways in aggregate formation by plant communities (as outlined above) might have a stronger effect in CC mixtures. However, the question of whether biodiverse CC mixtures are able to increase plant-derived soil structure remediation has not yet been investigated. Further, direct root effects of CCs on soil structure formation during their growth have already been documented (Hudek et al., 2022; Blanco-Canqui and Ruis, 2020), but there is still a lack of information on how long term these changes are. Here, we refer to the long-term effect that a CC has on the soil beyond its active growing period as the legacy effect.
We hypothesize that CCs alter soil structure according to their root morphology so that CC mixtures which combine diverse functional root traits enhance aggregate stability as compared to single species. We further hypothesize that consecutive cover cropping results in a legacy effect of soil structure improvement for the subsequent main crops. Therefore, we investigated the aggregation pattern of four single and two CC mixtures of 4 and 12 species, respectively, in comparison to a fallow treatment in a long-term field experiment in Germany. We aimed to explore the legacy effect of CC on soil structure in the second main crop rotation following CC treatments.
2.1 Long-term experimental site and soil sampling
The samples were collected from a long-term field trial at the Asendorf field station of the Deutsche Saatgutveredelung AG (DSV), 70 km north of Hanover, Germany (49 m above sea level; N, E). The climate is temperate oceanic with an annual mean temperature of 9.3 ∘C and a mean annual precipitation of 751 mm (long-term mean, 1981–2010). The soil developed from a shallow loess cover over glaciofluvial sand (>50 cm) and was classified as a Stagnic Cambisol according to the World Reference Base (IUSS Working Group WRB, 2022). Soil texture was a silty loam with low heterogeneity across the field site (Table S1 in the Supplement). The soil pH was slightly acidic (pH 6.0–6.4), and soil organic C decreased from 1.6 % in the topsoil (0–30 cm) to 0.8 % in the subsoil (30–60 cm). The experiment was conducted as a fully randomized block design with three field replications per treatment. In total, 21 plots, 9 m×9 m in size (including 0.7 m edges), were sampled for this study.
The CC field trials were established in 2015 and incorporated in a conventionally managed 2-year crop rotation with winter wheat (Triticum aestivum L.) in the first year, followed by maize (Zea mays L.) in the second. The wheat straw remained on the field and was incorporated into the soil by a cultivator and harrow. The maximum cultivation depth was 15 cm. The maize was harvested as whole-plant silage. Seven CC treatments were investigated: (i) fallow with no CC treatment (pure stands as single crops); (ii) mustard (Sinapis alba L.); (iii) lacy phacelia (Phacelia tanacetifolia BENTH.); (iv) bristle oat (Avena strigosa SCHREB); (v) Egyptian clover (Trifolium alexandrinum L.); (vi) Mix4, a mix of the four single species; and (vii) Mix12, a commercial 12-species CC mix (TerraLife® MaizePro TR Greening, DSV, Lippstadt, Germany). Mix12 was 23 % legumes (in shoot dry mass), namely, field pea (Pisum sativum L.), crimson clover (Trifolium incarnatum L.), alsike clover (Trifolium hybridum L.), Persian clover (Trifolium resupinatum L.), and Hungarian vetch (Vicia pannonica CRANTZ.), and non-legume species, namely, sorghum (Sorghum sudanense STEUD.), common flax (Linum usitatissimum L.), lacy phacelia, deeptill radish (Raphanus sativus L.), ramtil (Guizotia abyssinica CASS.), sunflower (Helianthus annuus L.), and camelina (Camelina sativa L.). The fallow period was maintained mechanically or by herbicide application. Information on the long-term management and agronomic practices are presented in the Supplement (Sect. S1). For further information from this long-term field site on specific topics, we like to refer to the following publications: Gentsch et al. (2020) for net ecosystem exchange and photosynthetic C input to the soil, Gentsch et al. (2022) for soil water availability and mineral N management, Heuermann et al. (2019) vertical root distribution, Heuermann et al., (2022) for nutrient transfer to maize, and Heuermann et al., (2023) for metabolite profiles of the four single CCs. Plant biomass, root-to-shoot ratios, C:N ratios, and total nitrogen (TN) in biomass are presented in Table S4.
Soil samples were collected with minimal disturbance on 19 and 20 October 2020, after sowing of winter wheat, which equates to 7 months after incorporating the CC residues. Sampling occurred after completion of the third crop rotation 6 years after the experimental start. The samples were collected from three soil depth increments (0–10, 10–20, and 30–40 cm) by cutting a clod () with a sharp spatula and were carefully transferred to the sample container that fit the size of the clod. Three replicates were collected per treatment and transported (mounted on foam) to the laboratory, air-dried in their clod forms, and gently crushed. After that, the samples were placed in a drying oven at 40 ∘C for 24 h. Samples for bulk density (BD) determination were collected by a 100 cm3 stainless core cutter. The cores were dried at 105 ∘C, and BD was determined gravimetrically.
2.2 Aggregate fractionation and stability index
Macroaggregate stability was determined according to Hartge and Horn (2009). Dry soil samples from the seven CC variants were separated into five different aggregate sizes by dry sieving. To determine the distribution of the aggregate classes 16–8, 8–4, 4–2, 2–1, and <1 mm, a nest of five sieves with mesh sizes of 16, 8, 4, 2, and 1 mm (VWR International, ISO 3310, 200 mm×50 mm, stainless steel) was used for manual sieving. From each sample, 120 g of the sieved fractions was transferred to glass backers.
To determine the aggregate stability, the 16–8, 8–4, 4–2, and 2–1 mm aggregate classes from dry sieving were mixed together for wet sieving in proportions corresponding to their original aggregate distribution in the sample. A wet-sieving apparatus was used for this purpose according to Hartge and Horn (2009). Each remixed sample was rewetted to 120 % of its dry weight and placed on the top of a sieve nest with mesh sizes of 8, 4, 2, 1, 0.5, and 0.25 mm. The nest was placed in a holder and suspended in a container of water. Thereafter, the nest was lowered to the point where the soil sample on the top sieve was just covered with water. The sieves were lowered and lifted by an electric motor with a standardized oscillation of 4.0 cm at a frequency of 38 cycles min−1. This procedure was performed for 5 min. After wet sieving, each aggregate class was carefully transferred from the sieves into a glass container. The water from the wet-sieving apparatus was decanted after the particles settled. Accordingly, the aggregate classes were flushed again with water into aluminium bowls. After drying at 105 ∘C, the aggregate classes were weighed, and two different stability indices were calculated. The MWD is the mean weight diameter of wet-sieved aggregates calculated according to Eq. (1):
where Xi is the average diameter in millimetres of a certain size fraction, Wi is the proportion by weight of aggregates in the size fraction, and n is the total number of size fractions (Obalum et al., 2019). The higher the MWD is, the more large water-stable aggregates remain in the sample. The GMD is the geometric mean diameter of wet-sieved aggregates calculated according to Eq. (2):
where Wi is the proportion of each aggregate class in relation to the weight of the soil samples (Zhou et al., 2020). The GMD is an estimate of the size of the most frequent aggregate size classes. The higher the value, the larger the mean aggregate size. Both indices assume that larger aggregates imply greater stability (Nimmo and Perkins, 2002).
2.3 Soil organic carbon analysis
An aliquot from each aggregate fraction and the bulk soil were homogenized in a ball mill and analysed for organic carbon (OC), total nitrogen (TN), and stable isotope ratios δ13C and δ15N using an Elementar IsoPrime 100 IRMS (IsoPrime Ltd., Cheadle Hulme, UK) coupled to an Elementar Vario MICRO cube EA C/N analyser (Elementar Analysensysteme GmbH, Hanau, Germany).
2.4 Statistical analyses
Histograms and density plots were used to explore the data distribution. Data were log transformed for statistical tests if the assumption of normality was not fulfilled. Levene's test for homogeneity of variance revealed that the variances of groups of treatments were not equal. Therefore, we used pairwise Welch's t tests (pairwise CI and multcompView package) to compare differences between treatments in R version 4.1.3 (R Core Team, 2022). The pairwise comparison was done for each soil horizon or aggregate fraction separately, and lowercase letters should only be compared within a framed facet (e.g. Fig. 2a). Lowercase letters appear in ascending order in relation to the mean, which means that the letter a always starts with the treatment of the lowest mean. The cut off between the terms significant and not significant was defined to be p<0.05. The overall effect of all CC treatments on MWD in comparison to the fallow was analysed by linear mixed models (LMM, lme4 package) taking CC variant (all treatments) or CC type (single species vs. mixtures) and soil depth as random variables with varying intercepts among CCs and depth. Mean values are provided ± standard error (SE). The impact of CC on OC distribution in aggregate fractions was analysed in a structural equation model (SEM) using the R package lavaan (Rosseel, 2012). We used a two-step approach for SEM construction. First, a base model was tested to confirm the relationship between the latent variables (constructed) and indicator variables (measured). The base model used a covariance matrix based on the correlation pattern between forcing variables (Fig. S9 in the Supplement). The latent variable “aggregate OC distribution” was composed of OC1 (OC in the <1 mm fraction), OC4_2 (OC in the 4–2 mm fraction), OC8_4 (OC in the 8–4 mm fraction), and OC16_8 (OC in the 16–8 mm fraction). Principal component analyses confirmed a similar loading of OC2_1 and OC4_2 on the first two components (eigenvalue>1), explaining 61 % of the variance in the data. As OC2_1 and OC4_2 are redundant variables, including both does not fit to the model structure. Thus, we excluded OC2_1 from the latent variable construction. The second latent variable was “soil properties”, which was composed of the OC content (bulk soil), BD, and clay content. All global and local fit parameters of the base model indicated that the model fit well to the data matrix (see the R markdown file in the data repository). In the second step, the structural equation was included in the base model. The aggregate OC distribution was used as an endogenous variable (variable explained from the model) that was predicted by CC type, soil properties, and MWD (predictor variables). The global and local fit parameters of the final model fitted the data properly, and all predictor variables showed a significant impact on the aggregate OC distribution. The r-squared values of the variables indicate how much of their variance was explained by the SEM. All statistical statements, models, and average values can be recalculated from the metadata and R scripts provided. All data and R scripts for evaluation were uploaded to Zenodo and are publicly available (DOI: https://doi.org/10.5281/zenodo.7147565).
3.1 OC stock changes
Soil OC concentrations and stocks in the topsoil increased significantly from the start of the experiment in 2015 to 2020 (Figs. S3 and S4 in the Supplement). The average OC concentrations increased from 1.80 % ± 0.06 % to 2.07 % ± 0.06 % in the 0–10 cm layer and from 1.79 % ± 0.06 % to 1.84 % ± 0.06 % in the 20–30 cm layer regardless of CC treatment (Fig. S4). No significant differences in OC stocks between the CC treatments and fallow were found in 2020. Total OC stocks to 40 cm soil depth ranged from 78.5 to 120.7 t ha−1 with no significant differences between treatments (Fig. S5b in the Supplement). Only in the 0–10 cm layer did phacelia show higher OC stocks compared to the other treatments (Fig. S5a). There was a strong negative correlation between OC and BD (R2=0.44, p<0.001) but not with soil texture. Reasons for the OC increase in all treatments, including fallow status, are discussed in the Supplement (Sect. S1) but will not be the topic of further discussion here.
3.2 Aggregate distribution and OC in aggregate fractions
Most of the soil aggregates (57.5 % to 74.2 %) were stored in the <1 mm fraction, highest in the topsoil and decreasing with soil depth (Fig. S6 in the Supplement). The intermediate fractions (8–4, 4–2, 2–1 mm) ranged from 5.6 % to 13.8 %, each. The largest fraction (16–8 mm) contributed least to the total soil aggregates (0 % to 7.0 %). A similar distribution was found for the proportion of OC in different aggregate fractions (Fig. S7 in the Supplement). The variability of OC distribution within aggregates was quite large between (Fig. 1) but also within CC treatments (Fig. S8). Thus, only a few of the observed trends were significantly different by pairwise comparison of treatments (Fig. S8). For example, the largest differences among CC treatments were found in the top 0–10 cm, between oat and Mix12, Mix4, and fallow. Of all treatments, oat led to the highest proportion of OC in the <1 mm fraction (Fig. 1), which was a significant difference from Mix12 (p=0.038) in the uppermost layer (Fig. S8). All pure cultivated CCs tended to store more OC in the <1 mm fraction in the 0–10 cm increment, whereas mixtures stored a greater proportion in the 16–8 mm fraction. In the soil increments below 20 cm, the data variability was large between treatments. Here, only Mix4 led to approximately 1 % more OC in the 2–1 mm fraction than oat at the 30–40 cm depth (Fig. S8).
3.3 Aggregate stability indicators
The MWD varied among treatments and soil depths (Fig. 2a). A larger MWD indicates that more large-scale aggregates are present after wet sieving. When focusing on individual soil horizons, pairwise comparison indicated significantly higher MWD compared to the fallow for clover at 0–10 cm (18.8 % higher, p=0.017), Mix12 at 20–30 cm (37.6 % higher, p=0.049), and phacelia (17.0 % higher, p=0.018) and Mix4 (12.8 % higher, p=0.037) at 30–40 cm. A comprehensive data evaluation of the LMMs indicated that the MWD increased with soil depth and was significantly higher in the CC treatments than in the fallow treatment (Fig. 2b). The LMMs across soil depths indicated that all CCs increased the MWD from 10 % for oat and up to 19 % for Mix12 compared to fallow (Table 1, model 1). Only the increase from oat was not significant. A second model on CC type (Table 1, model 2) indicated that CC mixtures showed a higher MWD increase (16 %) than single species (12 %). We explored the relationship between MWD and the soil parameters' OC content, texture, and BD. None of the parameters showed a significant relationship to MWD (see R markdown file in the data repository). The CC litter C:N ratio was significantly negatively related to the MWD in the topsoil (R2=0.35, p=0.01, Fig. S9) but not in the subsoil (data on CC C:N ratios were published in Gentsch et al. (2022) and Table S3 in the Supplement). Similar trends were also found with GMD (see R markdown file in the data repository).
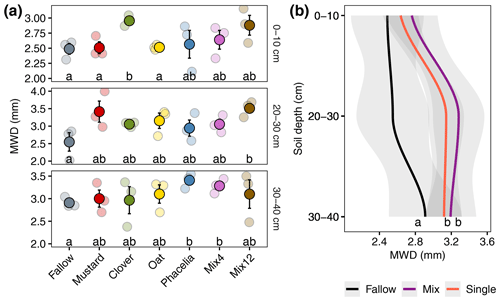
Figure 2Mean weight diameter (MWD) of soil aggregates after wet sieving from different soil depths. Lowercase letters in (a) denote significant differences between CC treatments by pairwise comparison for each soil depth independently. Lowercase letters in (b) denote overall effects of CC from a LMM. Transparent points represent the individual measurements, and full colours are mean values (± SE). Single refers to pure cultivated CCs, and Mix refers to CC mixtures.
Table 1Results of two LMMs with MWD as the response and CC variant or CC type as the predictor variable. Soil depth was set as a random variable. Interpretation: the estimated mean of fallow is the reference group, and changing the treatment from fallow to mustard will increase the MWD by 0.33 mm, which represents an increase of 12.4 %.
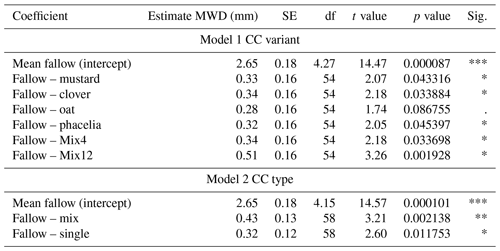
Furthermore, we tested the relationship between MWD and OC in different aggregate size fractions (Fig. S9). There was a significant positive correlation between MWD and OC in the 8–4 mm fraction (R2=0.25, p<0.001), a slightly positive but significant trend with 16–8 mm sized aggregates (Fig. S9), and a negative correlation with OC in the <1 mm fraction (R2=0.19, p<0.001). Similar trends to what we identified for the MWD were also observed for the GMD, but the evaluation with an LMM showed significantly higher GMD compared to fallow only for mixed CC (Fig. S11 in the Supplement).
3.4 Impacts on aggregate OC distribution
The impact of soil parameters and CC type on aggregate OC distribution was evaluated by SEM (Fig. 3). The overall fit parameters indicated that the model fit the data satisfactorily (χ2=28.7, p=0.12, RMSEA=0.08). The r square of the SEM variables indicated that 70 % of the variance in aggregate OC distribution is explained by the model (see R markdown file in the data repository). The regression parameters in the SEM indicated that all of the selected predictor variables showed a significant impact on aggregate OC distribution (p<0.02). The standardized estimates of the predictors indicated that MWD had the highest impact on aggregate OC distribution, followed by soil parameters, and CC type (Fig. 3). The factor CC type was composed of three factors in the order mix, fallow, and single. The interpretation of the CC type in SEM is visualized in the R markdown file (see R markdown file in the data repository). By the change in the CC type from mix to fallow to single CCs, the OC distribution is affected positively, which means that OC in the <1 mm fraction increases, while OC in the larger fractions decreases.
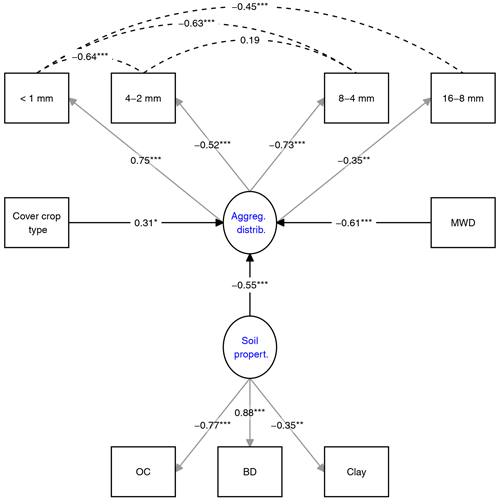
Figure 3Structural equation model (SEM) investigating the impact of parameters on aggregate OC distribution. Latent variables (blue) are predicted by grey-arrowed observed variables. Dashed lines indicate covariance variables. Numbers show standardized estimates with p values as asterisks. All model parameters are shown in the R markdown file (Supplement). MWD refers to mean weight diameter, and BD refers to bulk density.
4.1 Changes in aggregate stability
The MWD and the GMD both serve as aggregate stability indices assuming that more of the larger aggregates resist wet-sieving treatment. The results indicated that the repeated application of CC (Table 1, Fig. 2b) increased the average size of soil aggregates and their stress resistance compared to fallow. Larger aggregates consist of subunits of smaller aggregates that are internally bound more strongly to each other as the MWD and GMD indices increase (Nimmo and Perkins, 2002). We found no correlation between the MWD and soil texture, BD, or bulk soil OC content (see R markdown file in the data repository). This suggested that factors other than soil properties determine differences in aggregate stability at the experimental site. The average MWD increase over fallow was slightly higher for CC mixtures (16 %) than for single CCs (12 %). Despite a partially large variability of the data (Fig. 2a), some CC species appeared to have a stronger effect at a specific soil depth than others. Clover significantly increased the MWD in the 0–10 cm increment, while phacelia increased the MWD in the 30–40 cm increment compared to the fallow (Fig. 2a). As both species are part of the mixtures, these trends can also be observed in the mixtures. Clover, however, was usually only a minor component in Mix4 (<1 %–5 % of the total root biomass, Heuermann et al., 2019), and the effect in the 0–10 cm increment was not present in Mix4. Differences in root morphology between species at the sampling site are well documented in Heuermann et al. (2019) and might be one factor for differences in MWD between species. Clover has its maximum root biomass in the upper 20 cm soil depth, while phacelia can root approximately 70 cm and deeper. Clover also showed the highest root-to-shoot ratio of all CC treatments (Table S4 in the Supplement). In a recent study, total root length and root surface area correlated positively with aggregate stability, and it was shown that several different CCs led to higher aggregate stability in the topsoil (0–15 cm, Hudek et al., 2022).
Our models indicated that the largest differences in MWD (Fig. 2b) and particularly in GMD (Fig. S10b in the Supplement) between CC and the fallow occurred in the 20–30 cm soil layer. As the maximum cultivation depth during the experiment was 15–20 cm, this indicates that the layers below the cultivation depth benefit most from CC treatments. Soil cultivation and tillage have a strong impact on aggregate stability and soil structure, resulting in lower MWD (Obalum et al., 2019) and lower connectivity of the pore network (Lucas et al., 2019). Plant activity, on the other hand, has been demonstrated to establish stable and connected biopore systems within 6 years (Lucas et al., 2019). Here, we demonstrate that, 6 years after the last ploughing to 30 cm depth (see Sect. S1 in the Supplement), the incorporation of CC resulted in a faster improvement of MWD and GMD in comparison to fallow treatments. Therefore, reduced soil cultivation can be even more beneficial for soil structure amelioration if CCs are incorporated into the crop rotation.
The significantly higher MWD and GMD values of Mix12 at 20–30 cm might indicate additional benefits for aggregate stability when more biodiverse CC mixtures are grown. Apart from root morphology, the quality and quantity of root exudates and plant litter (root and shoot) can be quite different between CC plant species. The metabolite profiling of the four single CCs at the sampling site revealed that every plant species showed a characteristic pattern of chemical compounds that are released into the soil (Heuermann et al., 2023). In particular, secondary metabolites with signalling functions were closely related to certain plant species. For example, the presence of phenylpropanoids, which regulate interactions between plants and their microbial associations, was largely confined to clover (Heuermann et al., 2023). The authors found various plant-specific secondary metabolites with functions for improving iron availability: as chemotactic agents for microbes, as plant-microbial signalling, as signals in plant–plant communication, as allelochemicals, as biotic defence, or as nitrogen sources for neighbours. Also the total carbon in field root exudates differed strongly between CCs and was highest for phacelia.
Plants shape the microbial community in their rhizosphere by the composition of their root exudates (Reinhold-Hurek et al., 2015; Ulcuango et al., 2021). Root biomass and root exudates are linked to higher fungal and bacterial biomass (Eisenhauer et al., 2017). The study of Thapa et al. (2021) indicated that CCs resulted in a 41 % greater fungal community than the fallow. But also the fungal community composition and their functional traits are strongly affected by the CC type (Cloutier et al., 2020). Therefore, we suggest that the significantly higher impact of clover in the 0–10 cm layer was derived from the higher rhizosphere input and attraction of microbial mediators suitable for aggregate formation, such as fungi (A. Lehmann et al., 2020). This might also explain the better performance of CC mixtures for aggregate formation compared to single species and the differences between CC treatments in the subsoil. Previous experiments at the same site showed higher photoassimilate C transport rates to the rhizosphere for CC mixtures, prolonged mean residence times of these compounds in the soil, and the connection to higher fungal activity compared to single CC (Gentsch et al., 2020). Additionally, Baumert et al. (2018) found significant promotion of particular fungi by greater exudate release, which have the largest impact on macroaggregate formation in the subsoil.
The OM chemistry that is incorporated into the soil plays an important role in the aggregation process. A recent meta-analysis indicated that plant residue amendments and the type of plant material have a strong positive impact on MWD (Husain and Dijkstra, 2023). In a study of different OM types, Sarker et al. (2018) used 13C NMR spectroscopy to evaluate the impact on MWD (referred to as the aggregation index in the study). The authors found that materials with high protein contents and low C:N ratios, such as alfalfa litter, showed a rapid positive response to MWD. With a similar approach, Halder et al. (2021) showed a positive correlation of MWD to alkyl and carbonyl C and soluble sugars that were highest in legume litter (alfalfa, vetch species) with low C:N ratios. Both of the former studies also showed that materials such as maize litter or wheat straw, rich in cellulose and hemicellulose, with wide C:N ratios but being poor in soluble fractions, had an intermediate but persistent response to MWD. Plant C:N ratios strongly correlate with the quantity of cellulose and hemicellulose in plant residues (Jensen et al., 2005). Thus, cover crops with wider C:N ratios such as oat, mustard, or oilseed radish typically show higher quantities of cellulose and hemicellulose than litters with low C:N ratios, such as legumes (Ţîţei, 2002; Oliveira et al., 2016; Halder et al., 2021). In our study, we found a significant negative correlation between MWD and the C:N ratio of CC litter in the 0–10 cm increment but not in the deeper layers (Fig. S9). This suggested that the litter quality of CC residues plays an important role in aggregate stability when incorporated into the topsoil. The C:N ratios were ranked in the order of clover, Mix12, phacelia, mustard, Mix4, and oat, suggesting that the positive impact of CC on MWD in the topsoil decreased from clover to oat. The low-quality litter input from clover or Mix12 might also help to decompose the more structure-rich residues of the previous winter wheat straw more efficiently (Barel et al., 2019).
Below the cultivation depth, factors other than litter quality, such as root exudates, might be more prominent for aggregate formation. Fresh plant residues induce the formation of macroaggregates as a result of higher microbial activity (Six et al., 2000). Litter quality exerts control on the decomposer community composition, which will change as a result of decomposition (Marschner et al., 2011; Berg and McClaugherty, 2014). These processes result in microbial-derived organic substances that are key to building up soil aggregates.
We conclude that the combination of plants with different litter qualities and rhizodeposits might explain the better performance of CC mixtures, as well as differences between CC species for soil aggregate amelioration. Consecutive integration of CC in the crop rotation resulted in a positive legacy effect on aggregate stability. Reduced cultivation depth in combination with CC mixtures might be an additional benefit to improve the soil structure in the subsoil.
4.2 OC distribution in aggregates
The impact of various parameters on aggregate OC distribution was explored by SEM. The model indicates that the MWD and soil properties had the strongest direct effect on aggregate OC distribution (Fig. 3). Soil properties are well known for their contribution to soil aggregate formation. Schweizer et al. (2019), for example, showed the dependency of macroaggregate formation on clay content, while Blanco-Canqui and Lal (2004) emphasized the role of OM quality and quantity in aggregate formation and OC sequestration. A lower but significant effect on the OC distribution was found for the CC type (Fig. 3), suggesting that it matters if we grow CC instead of fallow as mixtures or single species. Although difficult to detect in pairwise comparisons, mixtures tended to increase OC in the 16–8 mm fraction, while single species supported OC in the <1 mm fraction, at least in the topsoil (Figs. 1, S7, and S8 in the Supplement). The SEM supports these trends and suggests that, with the change in the treatments from CC mixtures to fallow to single CC, OC in the <1 mm fraction will increase, while OC in the larger fractions will decrease. The tendency towards more OC in larger soil fractions might be one of the reasons for the increasing MWD after mixed CC treatments and the positive linear relationship between MWD and OC content in fractions 4–8 and 8–16 mm (Fig. S9). These correlations also underscore that OC stored in the two largest fractions is more stable to withstand induced forces from our wet-sieving treatment.
Management practices were found to have a strong impact on OC distribution within aggregates, and a reduction in tillage resulted in higher OC storage in macroaggregates compared to conventionally tilled soils (Blanco-Canqui and Lal, 2004). Here, we demonstrated that crop history has a legacy effect on the OC distribution and that the incorporation of CC can change the OC distribution within aggregate classes. Classical concepts (such as Tisdall and Oades, 1982) indicate that plants and crop management are strong factors in macroaggregate formation, where the number of stable macroaggregates decreases when rhizosphere products and hyphae are decomposed and not replaced. Later studies confirmed the role of crop rotation in the management of aggregate binding agents (Chan and Heenan, 1999; Blanco-Canqui and Lal, 2004). Overall, our results demonstrate that CC incorporation attenuates negative effects on soil structure that come from soil cultivation. The compensation effect was even stronger when biodiverse CC mixtures were applied.
This study demonstrates that the stability of macroaggregates and OC distribution in aggregate fractions in arable soils partially depend on crop rotation and incorporation of CCs. We argue that CC aggregate formation depends on the litter quality, root morphology, and rhizosphere input of CCs, which stimulate microbial mediators for aggregate formation. Litter quality had positive effects in the topsoil (0–10 cm) when CC residues with low C:N ratios were incorporated into the topsoil. Layers below the cultivation depth (>15 cm) showed the strongest response to CC treatments, and root morphology together with exudate-stimulated microbial growth was more likely to be the factor for the positive effects observed. Cover crop mixtures tended to have additional benefits for the MWD over single CCs and increased OC accumulation in large aggregate fractions (16–8 mm). The consecutive integration of CCs in crop rotation can be used to ameliorate aggregate stability in cultivated soils. Reduced soil cultivation depth can be even more beneficial for soil structure amelioration if CCs are incorporated into the crop rotation. Stable and agglomerated aggregates are core parameters for soil quality and one of the key indicators in the soil health concept. Aggregation affects root penetration, decreases the risk of soil erosion, and increases hydraulic functions. Aggregates are hotspots for biogeochemical cycling and contribute to plant nutrition. Therefore, we conclude that the improvement of soil structure that we observed is one of the factors mediated by CCs, leading to higher and resilient crop yields. Soil structure improvement is one of the multiple services that CCs fulfil in agroecosystems and, as such, is part of a multifunctional concept of CC. Further research might explore multiple functional traits in CC mixtures and identify smart plant species combinations to maximize the benefits of cover cropping.
All metadata and R code to reproduce the content of this study are publicly available under the Creative Commons Attribution 3.0 Germany. The files are accessible from the Zenodo archive through the following DOI: https://doi.org/10.5281/zenodo.7147565 (Gentsch, 2022).
The supplement related to this article is available online at: https://doi.org/10.5194/soil-10-139-2024-supplement.
NG designed the experiment and carried out the fieldwork and all statistical analyses. FLR participated in field work carried out most of the laboratory work and provided raw data. NG and FLR were writing the paper. DH participated in the data analyses and helped with the discussion and improvements of the paper. DS and UF maintained the field sites and carried out all agronomic measures. GG and JB developed the project ideas, recruited funding, and participated in improvement of the paper.
The contact author has declared that none of the authors has any competing interests.
Publisher's note: Copernicus Publications remains neutral with regard to jurisdictional claims made in the text, published maps, institutional affiliations, or any other geographical representation in this paper. While Copernicus Publications makes every effort to include appropriate place names, the final responsibility lies with the authors.
This work is part of the BonaRes (Soil as a Sustainable Resource for the Bioeconomy) project CATCHY (Catch-cropping as an agrarian tool for continuing soil health and yield increase), funded by the German Federal Ministry of Education and Research (BMBF). We are grateful to Silke Bokeloh and the whole laboratory team from the Institute of Soil Science for the assistance with sample preparation and measurements.
This research has been supported by the Bundesministerium für Bildung und Forschung (grant no. 031B1060C).
The publication of this article was funded by the open-access fund of Leibniz Universität Hannover.
This paper was edited by Ping He and reviewed by two anonymous referees.
Allen, D. E., Singh, B. P., and Dalal, R. C.: Soil Health Indicators Under Climate Change: A Review of Current Knowledge, in: Soil Health and Climate Change, edited by: Singh, B. P., Cowie, A. L., and Chan, K. Y., Springer, Berlin, Heidelberg, 25–45, https://doi.org/10.1007/978-3-642-20256-8_2, 2011.
Bacq-Labreuil, A., Crawford, J., Mooney, S. J., Neal, A. L., and Ritz, K.: Cover crop species have contrasting influence upon soil structural genesis and microbial community phenotype, Sci. Rep.-UK, 9, 7473, https://doi.org/10.1038/s41598-019-43937-6, 2019.
Barel, J. M., Kuyper, T. W., Paul, J., de Boer, W., Cornelissen, J. H. C., and De Deyn, G. B.: Winter cover crop legacy effects on litter decomposition act through litter quality and microbial community changes, J. Appl. Ecol., 56, 132–143, https://doi.org/10.1111/1365-2664.13261, 2019.
Baumert, V. L., Vasilyeva, N. A., Vladimirov, A. A., Meier, I. C., Kögel-Knabner, I., and Mueller, C. W.: Root Exudates Induce Soil Macroaggregation Facilitated by Fungi in Subsoil, Front. Environ. Sci., 6, https://doi.org/10.3389/fenvs.2018.00140, 2018.
Berg, B. and McClaugherty, C.: Plant litter, Springer, Berlin, Heidelberg, https://doi.org/10.1007/978-3-662-05349-2, 2014.
Blanco-Canqui, H. and Lal, R.: Mechanisms of Carbon Sequestration in Soil Aggregates, Crit. Rev. Plant Sci., 23, 481–504, https://doi.org/10.1080/07352680490886842, 2004.
Blanco-Canqui, H. and Ruis, S. J.: Cover crop impacts on soil physical properties: A review, Soil Sci. Soc. Am. J., 84, 1527–1576, https://doi.org/10.1002/saj2.20129, 2020.
Boix-Fayos, C., Calvo-Cases, A., Imeson, A. C., and Soriano-Soto, M. D.: Influence of soil properties on the aggregation of some Mediterranean soils and the use of aggregate size and stability as land degradation indicators, CATENA, 44, 47–67, https://doi.org/10.1016/S0341-8162(00)00176-4, 2001.
Chan, K. Y. and Heenan, D. P.: Microbial-induced soil aggregate stability under different crop rotations, Biol. Fert. Soils, 30, 29–32, https://doi.org/10.1007/s003740050583, 1999.
Chavarría, D. N., Verdenelli, R. A., Munoz, E., Conforto, E. C., Restovich, S. B., Andriulo, A. E., Meriles, J. M., and Vargas Gil, S.: Soil microbial functionality in response to the inclusion of cover crop mixtures in agricultural systems, Spanish J. Agr. Res., 14, e0304, https://doi.org/10.5424/sjar/2016142-8395, 2016.
Cloutier, M., Murrell, E., Barbercheck, M., Kaye, J., Finney, D., García-González, I., and Bruns, M.: Fungal community shifts in soils with varied cover crop treatments and edaphic properties, Sci. Rep.-UK, 10, 6198, https://doi.org/10.1038/s41598-020-63173-7, 2020.
Couëdel, A., Kirkegaard, J., Alletto, L., and Justes, É.: Crucifer-legume cover crop mixtures for biocontrol: Toward a new multi-service paradigm, in: Advances in Agronomy, vol. 157, Elsevier, 55–139, https://doi.org/10.1016/bs.agron.2019.05.003, 2019.
Dabney, S. M., Delgado, J. A., and Reeves, D. W.: Using Winter Cover Crops to Improve Soil and Water Quality, Commun. Soil Sci. Plan., 32, 1221–1250, https://doi.org/10.1081/CSS-100104110, 2001.
Drost, S. M., Rutgers, M., Wouterse, M., de Boer, W., and Bodelier, P. L. E.: Decomposition of mixtures of cover crop residues increases microbial functional diversity, Geoderma, 361, 114060, https://doi.org/10.1016/j.geoderma.2019.114060, 2020.
Dungait, J. A. J., Hopkins, D. W., Gregory, A. S., and Whitmore, A. P.: Soil organic matter turnover is governed by accessibility not recalcitrance, Glob. Change Biol., 18, 1781–1796, https://doi.org/10.1111/j.1365-2486.2012.02665.x, 2012.
Eisenhauer, N., Lanoue, A., Strecker, T., Scheu, S., Steinauer, K., Thakur, M. P., and Mommer, L.: Root biomass and exudates link plant diversity with soil bacterial and fungal biomass, Sci. Rep.-UK, 7, 1–8, https://doi.org/10.1038/srep44641, 2017.
Gentsch, N.: Cover crops improve soil structure and change OC distribution in aggregate fractions (Version 2), Zenodo [data set and code], https://doi.org/10.5281/zenodo.7147565, 2022.
Gentsch, N., Boy, J., Batalla, J. D. K., Heuermann, D., von Wirén, N., Schweneker, D., Feuerstein, U., Groß, J., Bauer, B., Reinhold-Hurek, B., Hurek, T., Céspedes, F. C., and Guggenberger, G.: Catch crop diversity increases rhizosphere carbon input and soil microbial biomass, Biol. Fert. Soils, 56, 943–957, https://doi.org/10.1007/s00374-020-01475-8, 2020.
Gentsch, N., Heuermann, D., Boy, J., Schierding, S., von Wirén, N., Schweneker, D., Feuerstein, U., Kümmerer, R., Bauer, B., and Guggenberger, G.: Soil nitrogen and water management by winter-killed catch crops, SOIL, 8, 269–281, https://doi.org/10.5194/soil-8-269-2022, 2022.
Halder, M., Liu, S., Zhang, Z. B., Guo, Z. C., and Peng, X. H.: Effects of residue stoichiometric, biochemical and C functional features on soil aggregation during decomposition of eleven organic residues, CATENA, 202, 105288, https://doi.org/10.1016/j.catena.2021.105288, 2021.
Hartge, K. H. and Horn, R.: Die physikalische Untersuchung von Böden, 4th edn., E. Schweitzerbart'sche Verlagsbuchhandlung (Nägele u. Obermiller), Stuttgart, 2009.
Heuermann, D., Gentsch, N., Boy, J., Schweneker, D., Feuerstein, U., Groß, J., Bauer, B., Guggenberger, G., and von Wirén, N.: Interspecific competition among catch crops modifies vertical root biomass distribution and nitrate scavenging in soils, Sci. Rep.-UK, 9, 1–11, https://doi.org/10.1038/s41598-019-48060-0, 2019.
Heuermann, D., Gentsch, N., Guggenberger, G., Reinhold-Hurek, B., Schweneker, D., Feuerstein, U., Heuermann, M. C., Groß, J., Kümmerer, R., Bauer, B., and von Wirén, N.: Catch crop mixtures have higher potential for nutrient carry-over than pure stands under changing environments, Eur. J. Agron., 136, 126504, https://doi.org/10.1016/j.eja.2022.126504, 2022.
Heuermann, D., Döll, S., Schweneker, D., Feuerstein, U., Gentsch, N., and von Wirén, N.: Distinct metabolite classes in root exudates are indicative for field- or hydroponically-grown cover crops, Front. Plant Sci., 14, 1122285, https://doi.org/10.3389/fpls.2023.1122285, 2023.
Hudek, C., Putinica, C., Otten, W., and De Baets, S.: Functional root trait-based classification of cover crops to improve soil physical properties, Eur. J. Soil Sci., 73, e13147, https://doi.org/10.1111/ejss.13147, 2022.
Husain, H. and Dijkstra, F. A.: The influence of plant residues on soil aggregation and carbon content: A meta-analysis, J. Plant Nutr. Soil Sc., 186, 177–187, https://doi.org/10.1002/jpln.202200297, 2023.
IUSS Working Group WRB: World Reference Base for Soil Resources. International soil classification system for naming soils and creating legends for soil maps, 4th edn., International Union of Soil Sciences (IUSS), Vienna, Austria, 2022.
Jensen, L. S., Salo, T., Palmason, F., Breland, T. A., Henriksen, T. M., Stenberg, B., Pedersen, A., Lundström, C., and Esala, M.: Influence of biochemical quality on C and N mineralisation from a broad variety of plant materials in soil, Plant Soil, 273, 307–326, https://doi.org/10.1007/s11104-004-8128-y, 2005.
Kutschera, L., Lichtenegger, E., and Sobotik, M.: Wurzelatlas der Kulturpflanzen gemäßigter gebiete mit Arten des Feldgemüsebaues, 2nd edn., DLG-Verlag, Frankfurt am Main, 2009.
Lal, R.: Soil degradation as a reason for inadequate human nutrition, Food Secur., 1, 45–57, https://doi.org/10.1007/s12571-009-0009-z, 2009.
Lal, R.: Restoring Soil Quality to Mitigate Soil Degradation, Sustainability, 7, 5875–5895, https://doi.org/10.3390/su7055875, 2015.
Lehmann, A., Zheng, W., and Rillig, M. C.: Soil biota contributions to soil aggregation, Nat. Ecol. Evol., 1, 1828–1835, https://doi.org/10.1038/s41559-017-0344-y, 2017.
Lehmann, A., Zheng, W., Ryo, M., Soutschek, K., Roy, J., Rongstock, R., Maaß, S., and Rillig, M. C.: Fungal Traits Important for Soil Aggregation, Front. Microbiol., 10, 2904, https://doi.org/10.3389/fmicb.2019.02904, 2020.
Lehmann, J., Bossio, D. A., Kögel-Knabner, I., and Rillig, M. C.: The concept and future prospects of soil health, Nat. Rev. Earth Environ., 1, 544–553, https://doi.org/10.1038/s43017-020-0080-8, 2020.
Liu, A., Ma, B. L., and Bomke, A. A.: Effects of Cover Crops on Soil Aggregate Stability, Total Organic Carbon, and Polysaccharides, Soil Sci. Soc. Am. J., 69, 2041–2048, https://doi.org/10.2136/sssaj2005.0032, 2005.
Lucas, M., Schlüter, S., Vogel, H.-J., and Vetterlein, D.: Soil structure formation along an agricultural chronosequence, Geoderma, 350, 61–72, https://doi.org/10.1016/j.geoderma.2019.04.041, 2019.
Marschner, P., Umar, S., and Baumann, K.: The microbial community composition changes rapidly in the early stages of decomposition of wheat residue, Soil Biol. Biochem., 43, 445–451, https://doi.org/10.1016/j.soilbio.2010.11.015, 2011.
Mendes, I. C., Bandick, A. K., Dick, R. P., and Bottomley, P. J.: Microbial Biomass and Activities in Soil Aggregates Affected by Winter Cover Crops Oregon State Univ. Agric. Exp. Stn. Technical paper 11 224, Soil Sci. Soc. Am. J., 63, 873–881, https://doi.org/10.2136/sssaj1999.634873x, 1999.
Nimmo, J. R. and Perkins, K. S.: 2.6 Aggregate Stability and Size Distribution, in: Methods of Soil Analysis, John Wiley & Sons, Ltd, 317–328, https://doi.org/10.2136/sssabookser5.4.c14, 2002.
Obalum, S. E., Chibuike, G. U., Peth, S., and Ouyang, Y.: Soil organic matter as sole indicator of soil degradation, Environ. Monit. Assess., 189, 176, https://doi.org/10.1007/s10661-017-5881-y, 2017.
Obalum, S. E., Uteau-Puschmann, D., and Peth, S.: Reduced tillage and compost effects on soil aggregate stability of a silt-loam Luvisol using different aggregate stability tests, Soil Till. Res., 189, 217–228, https://doi.org/10.1016/j.still.2019.02.002, 2019.
Oliveira, R. A. de, Brunetto, G., Loss, A., Gatiboni, L. C., Kürtz, C., Müller Júnior, V., Lovato, P. E., Oliveira, B. S., Souza, M., and Comin, J. J.: Cover Crops Effects on Soil Chemical Properties and Onion Yield, Rev. Bras. Ciênc. Solo, 40, e0150099, https://doi.org/10.1590/18069657rbcs20150099, 2016.
R Core Team: R: A Language and Environment for Statistical Computing. R Foundation for Statistical Computing, Vienna, 2022.
Reinhold-Hurek, B., Bünger, W., Burbano, C. S., Sabale, M., and Hurek, T.: Roots Shaping Their Microbiome: Global Hotspots for Microbial Activity, Annu. Rev. Phytopathol., 53, 403–424, https://doi.org/10.1146/annurev-phyto-082712-102342, 2015.
Rosseel, Y.: lavaan: An R Package for Structural Equation Modeling, J. Stat. Softw., 48, 1–36, https://doi.org/10.18637/jss.v048.i02, 2012.
Sarker, T. C., Incerti, G., Spaccini, R., Piccolo, A., Mazzoleni, S., and Bonanomi, G.: Linking organic matter chemistry with soil aggregate stability: Insight from 13C NMR spectroscopy, Soil Biol. Biochem., 117, 175–184, https://doi.org/10.1016/j.soilbio.2017.11.011, 2018.
Schweizer, S. A., Bucka, F. B., Graf-Rosenfellner, M., and Kögel-Knabner, I.: Soil microaggregate size composition and organic matter distribution as affected by clay content, Geoderma, 355, 113901, https://doi.org/10.1016/j.geoderma.2019.113901, 2019.
Seybold, C. A. and Herrick, J. E.: Aggregate stability kit for soil quality assessments, CATENA, 44, 37–45, https://doi.org/10.1016/S0341-8162(00)00175-2, 2001.
Six, J. and Paustian, K.: Aggregate-associated soil organic matter as an ecosystem property and a measurement tool, Soil Biol. Biochem., 68, A4–A9, https://doi.org/10.1016/j.soilbio.2013.06.014, 2014.
Six, J., Elliott, E. T., and Paustian, K.: Soil macroaggregate turnover and microaggregate formation: a mechanism for C sequestration under no-tillage agriculture, Soil Biol. Biochem., 32, 2099–2103, https://doi.org/10.1016/S0038-0717(00)00179-6, 2000.
Stegarescu, G., Reintam, E., and Tõnutare, T.: Cover crop residues effect on soil structural stability and phosphatase activity, Acta Agr. Scand. B-S. P., 71, 992–1005, https://doi.org/10.1080/09064710.2021.1973083, 2021.
Thapa, V. R., Ghimire, R., Acosta-Martínez, V., Marsalis, M. A., and Schipanski, M. E.: Cover crop biomass and species composition affect soil microbial community structure and enzyme activities in semiarid cropping systems, Appl. Soil Ecol., 157, 103735, https://doi.org/10.1016/j.apsoil.2020.103735, 2021.
Thorup-Kristensen, K., Magid, J., and Jensen, L. S.: Catch crops and green manures as biological tools in nitrogen management in temperate zones, Adv. Agron., 79, 227–302, 2003.
Tisdall, J. M. and Oades, J. M.: Organic matter and water-stable aggregates in soils, J. Soil Sci., 33, 141–163, https://doi.org/10.1111/j.1365-2389.1982.tb01755.x, 1982.
Ţîţei, V.: The quality of fresh and ensiled biomass from white mustard, Sinapis alba, and its potential uses, 2002.
Ulcuango, K., Navas, M., Centurión, N., Ibañez, M. Á., Hontoria, C., and Mariscal-Sancho, I.: Interaction of Inherited Microbiota from Cover Crops with Cash Crops, Agronomy, 11, 2199, https://doi.org/10.3390/agronomy11112199, 2021.
Williams, N. D. and Petticrew, E. L.: Aggregate stability in organically and conventionally farmed soils, Soil Use Manage., 25, 284–292, https://doi.org/10.1111/j.1475-2743.2009.00223.x, 2009.
Wright, S. F. and Anderson, R. L.: Aggregate stability and glomalin in alternative crop rotations for the central Great Plains, Biol. Fert. Soils, 31, 249–253, https://doi.org/10.1007/s003740050653, 2000.
Zhou, M., Liu, C., Wang, J., Meng, Q., Yuan, Y., Ma, X., Liu, X., Zhu, Y., Ding, G., Zhang, J., Zeng, X., and Du, W.: Soil aggregates stability and storage of soil organic carbon respond to cropping systems on Black Soils of Northeast China, Sci. Rep.-UK, 10, 265, https://doi.org/10.1038/s41598-019-57193-1, 2020.